DOI:
10.1039/D0RA09686E
(Paper)
RSC Adv., 2021,
11, 3703-3712
Bis-BODIPY linked-triazole based on catechol core for selective dual detection of Ag+ and Hg2+†
Received
14th November 2020
, Accepted 10th January 2021
First published on 19th January 2021
Abstract
Herein, we introduced a new chemosensor, bis-BODIPY linked-triazole based on catechol (BODIPY-OO) prepared by bridging two units of BODIPY fluorophore/triazole binding group with a catechol unit. A solution of this compound displayed 4- and 2-fold enhancements in fluorescence intensity after adding a mole equivalent amount of Ag+ and Hg2+ ions in methanol media, respectively. 1H NMR titrations of BODIPY-OO with Ag+ and Hg2+ suggested that the triazole was involved in the recognition process. BODIPY-OO showed high sensitivity toward Ag+ and Hg2+ over other metal ions with detection limits of 0.45 μM and 1 μM, respectively. It can also distinguish Hg2+ from Ag+ by addition of an EDTA. This compound can therefore be employed as practical fluorescent probe for monitoring the presence of Ag+ and Hg2+ ions.
Introduction
Since toxicity of heavy and transition metal ions affects biological and environmental systems, it is highly desirable to develop sensing probes to monitor and quantify these ions.1–3 Specifically, mercury is used in various industries such as pharmaceuticals, batteries, semi-conductors, agriculture, and paper industry.4 It is also widely used in dentistry and scientific research as amalgam.5,6 The Agency for Toxic Substances and Disease Registry (ATSDR) has ranked mercury as the third of most toxic substances.7 Its widespread use leads to the release and accumulation of mercury in general environment.8 Humans are exposed to mercury mainly through consumption of contaminated food, inhalation, and even through the skin causing gut lining and kidney damage, pneumonitis and disorder of many parts of the brain and peripheral nervous system.6 In addition to mercury, silver has been extensively used by many industrial applications such as in photovoltaics, photography, jewelry and silverware production.9,10 Silver has also long been known for its antimicrobial properties and has been used for years in the medical field.11 Although it shows a low level of toxicity to human,12 high levels of the silver ion can inhibit glutathione (GSH), markers, and antioxidant enzymes.13 Silver also reduces superoxide dismutase (SOD) levels causing an oxidative stress and production of reactive oxygen species (ROS). In addition, prolonged exposure to silver may lead to irreversible discoloration of skin or eyes, i.e. argyria or argyrosis.14
There are a lot of analytical techniques for mercury and silver determination such as atomic absorption spectrometry (AAS),15 inductively coupled plasma mass spectroscopy (ICP-MS),16 inductively coupled plasma-atomic emission spectrometry (ICP-AES),17 and electrochemical methods.18 However, these methods are time-consuming and require sophisticated instrumentation. A simple, inexpensive, and efficient alternative is fluorescent sensor where binding of the analyte leads to a change in fluorescence which requires only cheap routine spectrometers.19,20 A good fluorescent sensor should not only be sensitive and selective but also allows real-time monitoring of targeted metal ions with low response time.21,22
Small-molecule fluorescent chemosensors are usually composed of a fluorophore and a chelating or recognition unit. The fluorophores with excellent optical properties, such as coumarin,23 fluorescein,24 rhodamine25 and BODIPY26 provide highly sensitive fluorescent chemosensors. Among these, BODIPY derivatives show great potentials owing to their outstanding photophysical properties, i.e. spectral bands tunable along the whole visible spectrum, high molar absorption coefficients (>80
000 cm−1 M−1), and high fluorescence quantum yields.27,28 Owing to their soft character, nitrogen heterocyclic systems, e.g. pyridine,29 quinoline,30 imidazole,31 and triazole32 are mostly used as recognition units for various metal ions and tend to react with soft acids such as mercury and silver ions. The triazole ring has emerged as an exciting moiety in the study of sensors. This stems from a robust and green protocol for the synthesis of triazole derivatives.33 In addition to metal sensors, triazole is extensively used in the synthesis of catalyst,34 dendrimers,35 biomolecule conjugates,36 and metal organic frameworks.37
BODIPY–triazole combinations were reported as fluorescent chemosensors for the detection of metal ions, e.g. Hg2+,38 Cu2+,39 Al3+,40 and Ag+.41 This construction pattern offers following advantages: (i) click reaction affords triazoles in high yields with simple synthetic protocols; (ii) two triazole units acting as a chelation pocket can efficiently capture metal ions; (iii) the presence of two BODIPY fluorophore units improves the sensitivity of detection; and (iv) optical properties of the triazole containing BODIPY are easily tuned by modifications to spacers and chelating moieties.38–41 However, there are no reports on using such a fluorescent chemosensor template for recognizing multiple target ions which has the advantages of being cost-effective and highly efficient. In addition, the fluorescent chemosensors that can serve as bifunctional detection of mercury and silver ion are still rare.42–45 Herein, we designed and synthesized a new fluorescent sensor (BODIPY-OO) composed of two units of azido-BODIPY (2) and one unit of 1,2-bis(prop-2-ynyloxy)benzene (3) covalently linked by an alkyne–azide click chemistry approach (Scheme 1). Its spectroscopic properties were investigated. BODIPY-OO presented “turn-on” fluorescence behaviors toward the silver and mercury ions over other interfering metal ions.
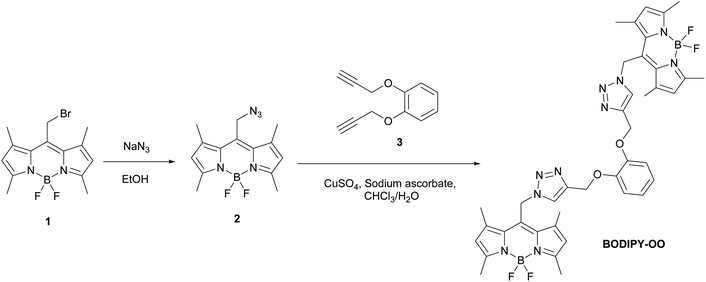 |
| Scheme 1 BODIPY-OO synthesis. | |
Experimental
Materials and equipment
Unless otherwise noted, all chemicals are of analytical reagent grade obtained from commercial suppliers and are used without further purification. Dichloromethane (CH2Cl2) was distilled from calcium hydride and stored under 4 Å molecular sieves. Water utilized in the analysis processes was obtained from Millipore system and deionized prior to use. Azido BODIPY 2 was synthesized following literature procedures.47 Alkyne 3 was prepared by conventional propargylation reaction using propargyl bromide and K2CO3. Column chromatography was performed on Merck silica gel 60 (70–230 mesh). 1H and 13C NMR spectra were recorded on Bruker Ascend™ 400 MHz spectrometers. The chemical shifts (δ) and coupling constants (J) are reported in parts per million (ppm) and Hertz (Hz), respectively. Tetramethylsilane (TMS) or residual non-deuterated solvents are used as internal reference materials in 1H NMR experiments. HRMS spectra were recorded on a HR-TOF-MS Micromass model VQ-TOF2. UV-Vis and fluorescence spectrometers were monitored on a UV-2600 (Shimadzu) and a FluoroMax® Plus (HORIBA), respectively.
Syntheses
Azido-BODIPY (2). 1 (100 mg, 0.29 mmol) was dissolved in ethanol (50 mL). To this mixture, sodium azide (89.0 mg, 1.37 mmol) was added. The round-bottom flask was then wrapped with aluminum foil and placed under argon. The reaction was stirred at 40 °C for 18 h. The solvent was removed under reduced pressure. The crude mixture was dissolved in ethyl acetate, washed with water, and dried over anhydrous Na2SO4. After removal of the organic solvent, the crude product was then purified on a silica gel column using an elution of 50% CH2Cl2 in hexane to afford an orange-red solid in 80% yield (70 mg). 1H NMR (400 MHz, CDCl3): δ 6.10 (s, 2H), 4.60 (s, 2H), 2.53 (s, 6H), 2.46 (s, 6H). 13C NMR (100 MHz, CDCl3): δ 156.81, 141.36, 133.23, 132.18, 122.70, 44.78, 16.23, 14.83. HRMS (ESI) m/z: calcd for C14H16BF2N5Na [M + Na]+, 326.1359; found, 326.1360.
1,2-Bis(prop-2-yn-1-yloxy)benzene (3). Propargyl bromide (294 mg, 2 mmol) and K2CO3 (276 mg, 2 mmol) were added to a solution of o-dihydroxybenzene (100 mg, 0.91 mmol) in acetone (5 mL). The mixture was heated to refluxing under argon for 18 h. After removal of solvent, the crude product was extracted with ethyl acetate, washed with water, and dried over anhydrous Na2SO4. It was then evaporated under reduced pressure and purified by chromatography on silica gel to afford 122 mg (85%) of 3 as a pale-yellow oil. 1H NMR (400 MHz, CDCl3): δ 7.08–7.05 (m, 2H), 7.01–6.97 (m, 2H), 4.76 (d, J = 2.2 Hz, 4H), 2.51 (t, J = 2.2 Hz, 2H). 13C NMR (100 MHz, CDCl3): δ 147.79, 122.32, 115.33, 78.75, 75.87, 57.06. HRMS (ESI) m/z: calcd for C12H10NaO2 [M + Na]+, 209.0573; found, 209.0572.
Sensor (BODIPY-OO). In a Schlenk tube, 3 (20 mg, 0.10 mmol) and 2 (65 mg, 0.21 mmol) were stirred in 3 mL of CHCl3 at room temperature under argon. To the solution mixture were added 20 mol% of CuSO4·5H2O and 40 mol% of sodium ascorbate dissolved in deionized water. The solution was stirred for 18 h, poured into water and extracted with CH2Cl2. The combined organic layers were washed with water and dried over anhydrous Na2SO4. After solvent evaporation, the crude product was further purified by column chromatography with a gradient eluent (25%, 50%, and 100% EtOAc in hexane). BODIPY-OO was obtained as a red solid in 66% yield (52 mg, 0.066 mmol). 1H NMR (400 MHz, CDCl3): δ 7.57 (s, 2H), 6.96–6.92 (m, 2H), 6.86–6.82 (m, 2H), 6.08 (s, 4H), 5.77 (s, 4H), 5.13 (s, 4H), 2.53 (s, 12H), 2.15 (s, 12H). 13C NMR (100 MHz, CDCl3): δ 157.81, 148.55, 144.49, 141.56, 132.36, 129.95, 123.16, 122.57, 122.23, 116.65, 63.56, 45.74, 15.82, 14.89. 11B NMR (128 MHz, CDCl3): δ −0.45 (t, JBF = 33.3 Hz). 19F NMR (377 MHz, CDCl3): δ −145.9 (q, JFB = 33.9 Hz). HRMS (ESI) m/z: calcd for C40H42B2F4N10NaO2 [M + Na]+, 815.3507; found, 815.3509.
Measurements of UV-Vis and fluorescence spectra
In UV-Vis studies, all metal salts including NaNO3, Mg(NO3)2·6H2O, Fe(NO3)3·9H2O, Co(NO3)2·6H2O, Ni(NO3)2·6H2O, Cu(NO3)2·3H2O, Zn(NO3)2·6H2O, Cd(NO3)2·4H2O, AgNO3, Pb(NO3)2, Hg(NO3)2, and Cr(NO3)3·9H2O were prepared in deionized water to get 10−2 M stock solutions. Solutions of the BODIPY-OO sensor (5 μM) were prepared in methanol. Test solutions were prepared by mixing 30 μL of each metal stock solution with 3 mL of the sensor solution in a quartz cuvette (path length = 1 cm). In fluorescence studies, solutions of sensor (0.5 μM) were prepared in methanol. Test solutions were prepared by mixing 3 μL of the metal stock solution with 3 mL of the sensor solution in a quartz cuvette (path length = 1 cm). The excitation wavelength was set at 470 nm and the emission wavelength range was 500–700 nm. The slit width was 3.0 nm for both excitation and emission experiments.
Fluorescence titrations
A solution (1 μM) of the BODIPY-OO sensor was prepared in methanol. 3–30 μL of a 10−3 M stock solution of Hg(NO3)2 or AgNO3 were added to 3 mL of the sensor solution. After mixing them in a quartz cuvette (path length = 1 cm) for a few seconds, fluorescence spectra were taken at room temperature.
Competition experiments
A solution of the sensor BODIPY-OO (0.5 μM) was prepared in MeOH. Stock solutions (10−2 M) of various metal ions were prepared. 3 μL of each metal solution was added to 3 mL of the sensor solution to make 20 equiv. of the metal ion. 3 μL of the Hg2+ or Ag+ solution was then added into the mixed solution to get 20 equiv. of Hg2+ or Ag+. After mixing them for a few seconds, fluorescence spectra were taken at room temperature.
1H NMR titrations
A solution of the sensor BODIPY-OO (1.00 mg, 0.001 mmol) in DMSO-d6 was prepared. 2–6 μL of 0.32 M AgNO3 solution in DMSO-d6 was added to the sensor solution to make a 1
:
0.5, 1
:
1, and 1
:
1.5 molar ratios of ligand to metal. After shaking them for a minute, 1H NMR spectra were taken at room temperature. For Hg2+, the same procedure was performed but HgCl2 and acetone-d6 were used as mercury source and solvent, respectively.
Computational calculation
The structure and electronic properties of BODIPY-OO, BODIPY-OO–Ag+ and BODIPY-OO–Hg2+ were calculated using density functional theory (DFT) and its extension to the time-dependent formulation (TDDFT) as implemented in Gaussian 09 package.48 The ground state structures were optimized in gas-phase with the B3LYP49,50 functional and 6-31G(d) basis set51,52 for the main group elements and LANL2DZ effective core potential (ECP)53–55 for Hg and Ag atoms. TDDFT calculations of the 10 lowest singlet–singlet transitions were carried out on the optimized ground state geometries using CAM-B3LYP56/6-31G(d), and CAM-B3LYP/GENECP for the free ligand and its complexes, respectively, adopting the conductor-like polarization (CPCM)57,58 model for the solvent (methanol) effect. Molecular orbitals were plotted using Avogadro program.59,60
Results and discussion
Construction of BODIPY-OO fluorescent platform
BODIPY-OO designed as a sensor for detection of multiple metal ions contains a catechol moiety and two triazole units in the binding core. Copper-catalyzed azide–alkyne cycloaddition (CuAAC) was used to synthesize BODIPY-OO as depicted in Scheme 1. Azido-BODIPY 2 was obtained by azidation of its bromo analog 1, while the terminal bisalkyne 3 was prepared by the propargylation reaction of catechol. The click reaction between bisalkyne-terminated catechol ether and azido-terminated BODIPY in the presence of CuSO4 and sodium ascorbate in CHCl3/H2O media resulted in the formation of the target compound in 66% yield.
BODIPY-OO was purified by column chromatography and characterized by 1H, 13C, 11B, 19F NMR, and FTIR spectroscopy, and HRMS spectrometry. The triazole protons of BODIPY-OO in the 1H NMR spectrum resonated at δ 7.57 ppm as a singlet. Aromatic protons of the catechol unit appeared over the range of δ 6.96–6.92 ppm and 6.86–6.82 ppm whereas signals at δ 6.08 ppm corresponded to protons at 2,6 positions of the BODIPY core. 1H chemical shifts of methylene protons attached to the BODIPY core and those attached to oxygen atoms were observed at 5.77 and 5.13 ppm, respectively. Methyl protons of the BODIPY units resonated at δ 2.53 and 2.15 ppm. In addition, the 11B and 19F NMR spectra of BODIPY-OO exhibited a typical triplet (11B) and quartet (19F) due to B–F coupling (Fig. S15 and S16, ESI†).
Sensing behaviors of BODIPY-OO
Optical properties of BODIPY-OO in methanol were first studied by absorption and emission spectroscopic methods (Fig. 1). The absorption spectrum displays three typical bands in the visible region: an intense main band at 517 nm attributed to typical π–π* transition (S0 → S1) of the BODIPY units; a small shoulder on the high-energy side of the main band at 489 nm ascribed to the 0–1 vibrational transition; and a weaker broad band at 375 nm which can be assigned to S0 → Sn (n ≥ 2) transitions.61 The fluorescence spectrum of BODIPY-OO was obtained after excitation within the spectral region of the absorption maximum which showed a single green fluorescence peak at 527 nm. BODIPY-OO has a fluorescence quantum yield (ΦF) of 0.06 relative to fluorescein (ΦF = 0.95 in 0.1 N NaOH) as shown in Table 1.46
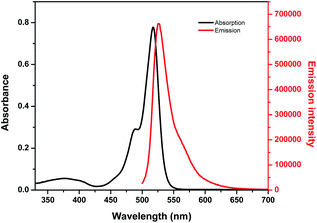 |
| Fig. 1 Absorption and emission spectra of BODIPY-OO (5 μM) in MeOH (λex = 470 nm, slit: 3). | |
Table 1 Photophysical properties of BODIPY-OO and its complexes
Compound |
λmaxabs (nm) |
ε (M−1 cm−1) |
λmaxem (nm) |
ΦFa |
Binding constant (M−1) |
Relative to fluorescein in 0.1 N NaOH (ΦF = 0.95).46 |
BODIPY-OO |
517 |
1.5 × 105 |
527 |
0.06 |
— |
BODIPY-OO–Ag+ |
517 |
1.8 × 105 |
527 |
0.22 |
1.56 × 105 |
BODIPY-OO–Hg2+ |
521 |
1.6 × 105 |
531 |
0.12 |
1.41 × 105 |
Our investigation began with the evaluation of the optical behaviors of BODIPY-OO in response to the addition (20 equiv.) of different ions such as Na+, Mg2+, Fe3+, Co2+, Ni2+, Cu2+, Zn2+, Cd2+, Ag+, Pb2+, Hg2+, and Cr3+. Fluorescence and UV-visible spectra indicated that BODIPY-OO was only sensitive to the Hg2+ and Ag+ ions (Fig. 2). Both ions increased the fluorescence intensity of the probe BODIPY-OO, when excited with λex = 470 nm. The addition of Hg2+ to BODIPY-OO resulted in a small redshift of the absorption spectrum whereas the emission curves displayed a 2-fold enhancement of the fluorescence intensity at 531 nm with ΦF of 0.12. In contrast, the addition of Ag+ led to a hyperchromic shift with molar extinction coefficient increasing from 1.5 × 105 M−1 cm−1 (BODIPY-OO) to 1.8 × 105 M−1 cm−1 (BODIPY-OO–Ag+). Notably, the chelation of BODIPY-OO with the Ag+ ion increased the fluorescence intensity at 527 nm of ca. 4-fold (ΦF = 0.22) with respect to BODIPY-OO (ΦF = 0.06). As shown in Fig. 3, our probe exhibited turn-on fluorescence toward Ag+ and Hg2+ likely as a result of chelation-enhanced fluorescence (CHEF) effect62,63 that is caused by the restriction of the intramolecular rotations in BODIPY-OO.64 The effect also decreases the non-radiative decay of the excited state. Notably, these results are different from the previously reported bifunctional probes for Ag+ and Hg2+ which mainly involved turn-off processes due to heavy atom effect and spin–orbit coupling (Table S1, ESI†).42,65–67
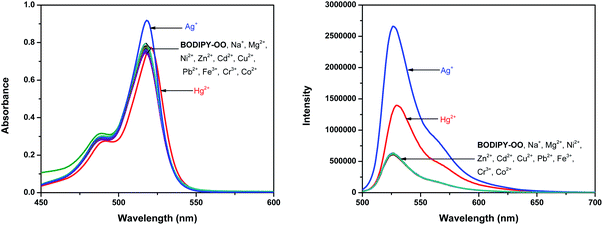 |
| Fig. 2 Left: absorption spectra of BODIPY-OO (5 μM) in the presence of various metal ions (20 equiv.) in MeOH. Right: emission spectra of BODIPY-OO (0.5 μM) in the presence of various metal ions (20 equiv.) in MeOH (λex = 470 nm, slit: 3). | |
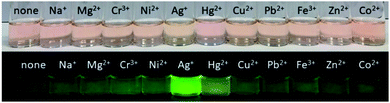 |
| Fig. 3 Top: photograph of BODIPY-OO (5 μM) toward tested metal ions (20 equiv.) in MeOH. Bottom: fluorogenic responses of BODIPY-OO (5 μM) toward tested metal ions (20 equiv.) in MeOH under UV light (λex = 365 nm). | |
Fluorescence titrations of BODIPY-OO with different ion concentrations
To further investigate the sensing behaviors of BODIPY-OO toward various concentrations of Ag+ and Hg2+, the fluorescence titration experiment was performed. The plots of fluorescence intensity as a function of the Ag+ and Hg2+ concentrations are shown in Fig. 4a and b, respectively. Upon sequential addition of Ag+ (0–10 equiv.) to the solution of BODIPY-OO, the fluorescence intensity of BODIPY-OO gradually increased and became saturated when the amount of Ag+ reached 9 equivalents when excited with λex = 470 nm. The detection limit of BODIPY-OO for the Ag+ detection was estimated to be 0.45 μM using the equation: LOD = 3σ/S,68 where S is the slope of the curve between the fluorescence intensities of the probe BODIPY-OO and the concentrations of Ag+, and σ is the standard deviation of four replicate measurements of the zero level (σ) (Fig. S1b, ESI†). After addition of increasing concentrations of Hg2+ (0–15 equiv.) to the solution of BODIPY-OO, the fluorescence intensity gradually increased, and eventually reached the plateau when the amount of Hg2+ reached 9 equivalents. Furthermore, the emission peak gradually redshifted from 527 nm to 531 nm. According to the fluorescence titration experiment, the detection limit of BODIPY-OO for the Hg2+ detection was 1 μM (Fig. S2b, ESI†).
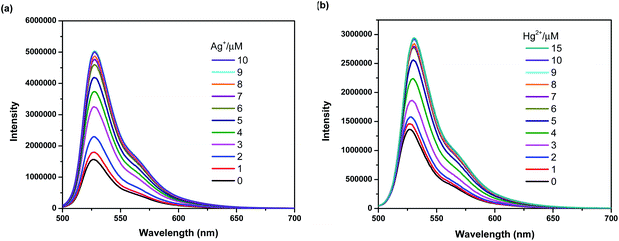 |
| Fig. 4 (a) Fluorescence spectra of BODIPY-OO (1 μM) with Ag+ in MeOH (λex = 470 nm, slit: 3). (b) Fluorescence spectra of BODIPY-OO (1 μM) with Hg2+ in MeOH (λex = 470 nm, slit: 3). | |
To obtain further insight into the binding characteristics of the Ag+ and Hg2+ ions with BODIPY-OO, Job plot analysis was carried out using fluorescence experiments in the presence of various molar fractions of Ag+ and Hg2+, respectively (Fig. S3 and S4, ESI†). A maximum emission was observed when the molar fraction of each metal ion reached the value of 0.5, indicating the formation of a 1
:
1 binding stoichiometry of both interactions between BODIPY-OO and Ag+, and between BODIPY-OO and Hg2+. In addition, association constant (Ka) of BODIPY-OO with Ag+ was calculated to be 1.56 × 105 M−1 using the Benesi–Hildebrand equation69 (Fig. S5, ESI†) and Ka of BODIPY-OO with Hg2+ was calculated to be 1.41 × 105 M−1 (Fig. S6, ESI†).
Interference of other metal ions
Competition experiments were conducted to investigate high selectivity toward specific analytes over other competitive species by adding 10 equiv. of either Ag+ or Hg2+ to the solution of BODIPY-OO in the presence of other common metal ions such as Na+, Mg2+, Fe3+, Co2+, Ni2+, Cu2+, Zn2+, Cd2+, Ag+, Pb2+, Hg2+, and Cr3+ (20 equiv.). Fig. 5a clearly revealed no changes in the fluorescence intensity of the BODIPY-OO–Ag+ complex upon addition of other metal ions with the exception of Hg2+. The addition of Hg2+ substantially perturbed the fluorescence emission of BODIPY-OO–Ag+ as it reduced about a half of the initial fluorescence intensity. This result implies that the Hg2+ ion can interfere the binding between BODIPY-OO and the Ag+ ion. A possible factor that affects the higher affinity of the Hg2+ ion as compared to Ag+ toward the receptor is the smaller ionic diameter (Hg2+, 2.0 Å; Ag+, 2.3 Å).70 Based on the interference experiment for the BODIPY-OO–Hg2+ complex, no significant changes occurred for the contact with the other ions (20 equiv.). As shown in Fig. 5b, the specific recognition of Hg2+ by BODIPY-OO was observed. When other interfering metal ions were added, there was no interference in the detection of Hg2+ from most of the metal ions.
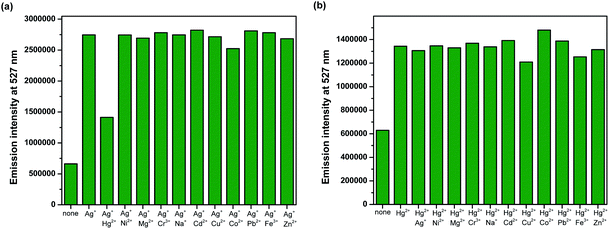 |
| Fig. 5 Competitive selectivity of: (a) BODIPY-OO (0.5 μM) toward Ag+ (10 equiv.) with other metal ions, and (b) BODIPY-OO (0.5 μM) toward Hg2+ (10 equiv.) with other metal ions. | |
The response behavior of BODIPY-OO toward the Ag+ and Hg2+ ions in terms of the binding ability and reversibility was further determined to discriminate the Ag+ from the Hg2+. The discriminative experiment was performed using ethylenediamine tetraacetic acid (EDTA) calcium disodium salt. The addition of EDTA to a solution of BODIPY-OO–Hg2+ led to an immediate decrease in the fluorescence intensity (Fig. 6). The intensity can be revived by the addition of Hg2+. It was also found that the sequentially alternative addition of Hg2+ and EDTA resulted in the reversible emission changes even after several cycles. These results suggested a potential of the probe to be recyclable simply through treatment with a proper reagent such as EDTA. In contrast, the emission changes were not observed for the BODIPY-OO–Ag+ complex after the addition of EDTA, (Fig. 7). In other words, the complexation reaction of BODIPY-OO with the Ag+ ion was irreversible. This is because EDTA is a good chelating agent for di- and trivalent metal ions and can be seen from the greater formation constant (Kf) for the Hg2+–EDTA complex (log(Kf) = 21.5) when compared with the Ag+–EDTA complex (log(Kf) = 7.20).71 Therefore, the addition of EDTA could assist to distinguish BODIPY-OO–Hg2+ from BODIPY-OO–Ag+.
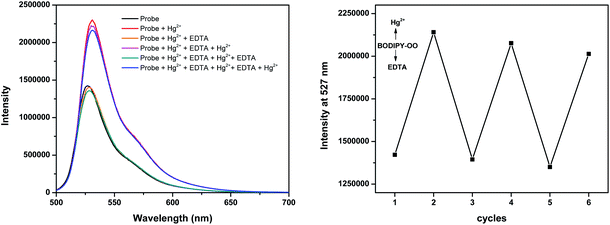 |
| Fig. 6 Fluorescence spectral changes of BODIPY-OO (1 μM) after the sequential addition of Hg2+ and EDTA. | |
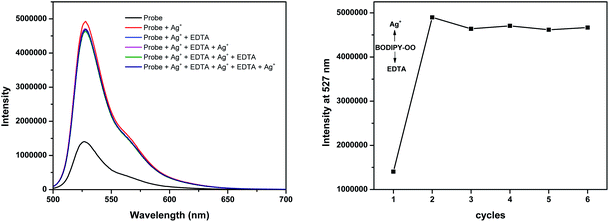 |
| Fig. 7 Fluorescence spectral changes of BODIPY-OO (1 μM) after the sequential addition of Ag+ and EDTA. | |
1H-NMR titration for BODIPY-OO with Ag+ and Hg2+
To understand complexation of BODIPY-OO with the Ag+ and Hg2+ ions, 1H NMR titrations were performed. Fig. 8 shows 1H NMR spectra of BODIPY-OO recorded in DMSO-d6 with different concentrations of Ag+ ranging from 0 to 1.5 equivalents. It was observed that the triazole (Ha) and methylene protons (He and Hf) at δ 8.21, 5.79, and 5.09 ppm were obviously downfield shifted to δ 8.38, 5.83, and 5.15 ppm, respectively, upon increasing concentrations of the Ag+. The downfield shift of the triazole and methylene protons may arise from the interaction of Ag+ with the probe through triazole nitrogen and catechol oxygen atoms. In contrast, coordination of BODIPY-OO with the Hg2+ changed the chemical shifts of neighboring protons in the binding site very slightly. As shown in Fig. 9, Ha, He, and Hf at δ 8.01, 5.90, and 5.12 ppm were shifted to δ 8.03, 5.91, and 5.14 ppm, respectively. Very small changes in the 1H NMR spectra of BODIPY-OO upon increasing concentrations of the Hg2+ are possibly the effect of the rapid quadrupolar relaxation of the Hg2+ ion.72–74
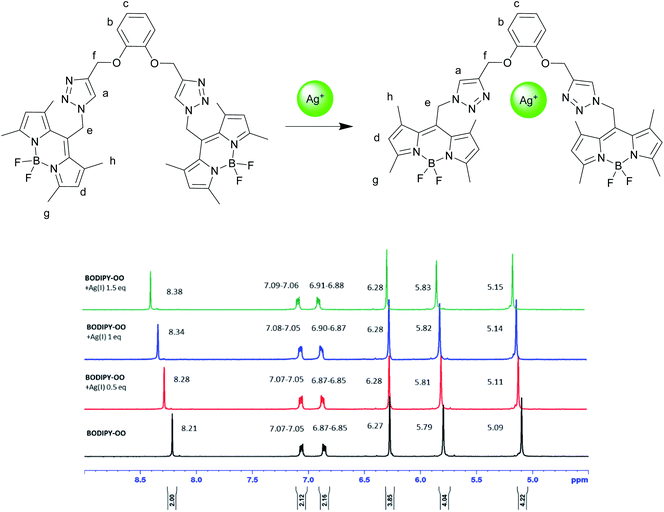 |
| Fig. 8 Partial 1H NMR titration spectra of BODIPY-OO in DMSO-d6 upon addition of Ag+ in various amounts. | |
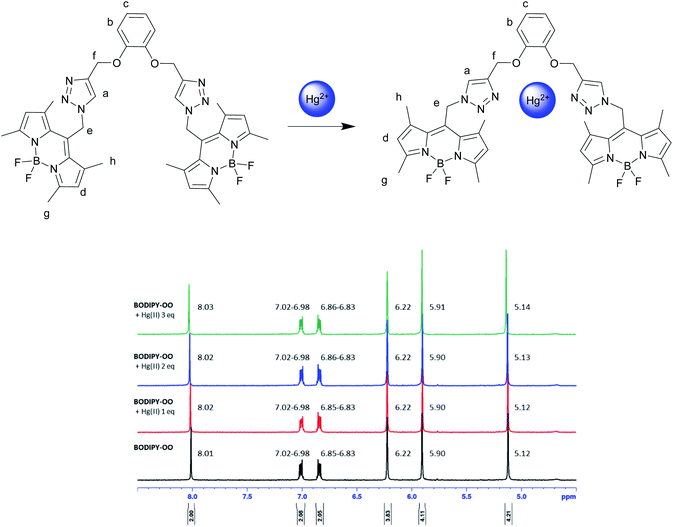 |
| Fig. 9 Partial 1H NMR titration spectra of BODIPY-OO in acetone-d6 upon addition of Hg2+ in various amounts. | |
Theoretical calculation studies
To get insight into the mechanism on the fluorescence sensing of BODIPY-OO toward Ag+ and Hg2+, density functional theory (DFT) calculations of the sensor and its complexes were performed. Fig. 10 illustrates the calculated energy-minimized structures of BODIPY-OO, BODIPY-OO–Ag+, and BODIPY-OO–Hg2+ complexes. It can be seen that the triazole units in the free ligand are arranged in a “face-to-face” fashion (Fig. 10a). Dihedral angles of O1–C1–C2–N1 and O1′–C1′–C2′–N1′ are found nearly the same, i.e. 114.886° and 114.883°, respectively. On the other hand, BODIPY-OO–Ag+ and BODIPY-OO–Hg2+ complexes showed significant changes in their geometries. In Fig. 10b, the optimized geometry of BODIPY-OO–Ag+ indicates that a stable complex was formed with the lone electron pairs of triazole nitrogen atoms (N1 and N1′) turned toward the bound Ag+ ion. The bond lengths of N1–Ag+ and N1′–Ag+ were 2.19 Å, whereas the dihedral angles of O1–C1–C2–N1 and O1′–C1′–C2′–N1′ were 13.734° and −9.848°, respectively. The bond angle between N1–Ag–N1′ of 168.77° was observed. In contrast, the optimized geometry of BODIPY-OO–Hg+ showed that not only N1 and N1′ of the triazole rings but also O1 and O1′ of the catechol unit were involved in the coordination with the Hg2+ center with the coordinated bond lengths of 2.22 Å (N1–Hg and N1′–Hg) and 2.48 Å (O1–Hg and O1′–Hg) (Fig. 10c). The calculated bond angle between N1–Hg–N1′ was 157.83°, whereas the dihedral angles of O1–C1–C2–N1 and O1′–C1′–C2′–N1′ were 0.020° and 0.033°, respectively. These results suggested that two coordinated nitrogens are not exactly linear and two triazole rings are almost in the same plane.
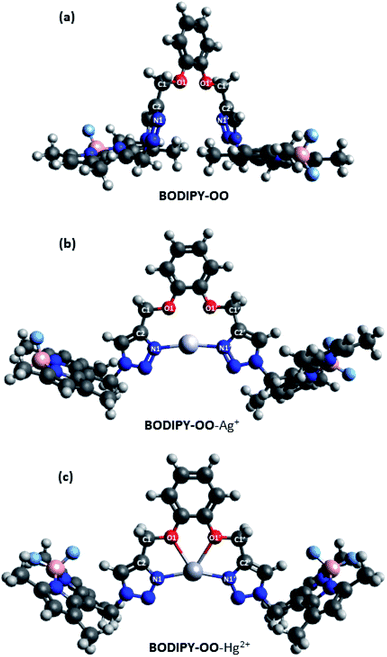 |
| Fig. 10 Optimized structures of (a) BODIPY-OO, (b) BODIPY-OO–Ag+, and (c) BODIPY-OO–Hg2+. | |
Furthermore, calculated frontier molecular orbitals (FMOs) of BODIPY-OO and its complexes are shown in Table S2, ESI,† and their calculated absorption data are summarized in Table 2. The main transition of BODIPY-OO was at 442 nm (λexp = 517 nm) which is related to π → π* transition of the BODIPY units. That of BODIPY-OO–Ag+ was found to be very similar at 440 nm (λexp = 517 nm) since there were no significant changes in electronic transition energies of BODIPY-OO and BODIPY-OO–Ag+, i.e. 2.80 eV and 2.82 eV, respectively. In contrast, the π → π* transition of BODIPY-OO–Hg2+ was observed at 456 nm (λexp = 531 nm) which was red-shifted with respect to that of BODIPY-OO and BODIPY-OO–Ag+ as a result of the lower energy transition (2.72 eV). These theoretical results are in good agreement with the experimental spectra. On the other hand, comparing with BODIPY-OO–Ag+, the lower energies of the HOMOs of BODIPY-OO–Hg2+ can be ascribed to a weaker electrostatic repulsion between the metal electrons and lone electron pairs of the triazole and catechol ligands directed toward the metal center.
Table 2 Calculated electronic transition energies and oscillator strengths of BODIPY-OO, BODIPY-OO–Ag+, and BODIPY-OO–Hg2+
Compound |
λmaxabs (nm) |
Band assignment |
Excitation energy (eV) |
Oscillator strength |
BODIPY-OO |
442 |
H−0 → L+0 (49%) |
2.80 |
1.21 |
H−1 → L+1 (49%) |
BODIPY-OO–Ag+ |
440 |
H−0 → L+1 (57%) |
2.82 |
1.08 |
H−1 → L+0 (41%) |
BODIPY-OO–Hg2+ |
456 |
H−0 → L+1 (49%) |
2.72 |
1.17 |
H−1 → L+0 (49%) |
Notably, the electron density in FMOs of the sensor and its complexes was distributed almost only over the BODIPY units. In other words, the HOMO and the LUMO are heavily biased to the BODIPY units and receive only very minor contributions from the coordination center. As a consequence, the relevant excitation is adequately described as a BODIPY-based π → π* transition with significantly no charge-transfer contributions from the triazole fragments. Therefore, mechanisms like intramolecular charge transfer (ICT) and photoinduced electron transfer (PET) were ruled out.
Conclusions
We have synthesized a new bifunctional chemosensor BODIPY-OO that can be used as a “turn-on” fluorescent probe for dual detection of Hg2+ and Ag+ ions. BODIPY-OO can clearly distinguish Hg2+ from Ag+ by the use of an EDTA. Modifications to chelating sites of this bis-BODIPY linked-triazole platform can not only bring more sensing information but also efficient differentiation of multiple analytes.
Conflicts of interest
There are no conflicts to declare.
Acknowledgements
This work was supported by the Faculty of Science, Mahidol University and the Center of Excellence for Innovation in Chemistry (PERCH-CIC), Ministry of Higher Education, Science, Research and Innovation. WS acknowledges the Science Achievement Scholarship of Thailand (SAST) for his scholarship. Finally, the authors would like to thank Assoc. Prof. Panida Surawatanawong and Mr Phiphop Naweephattana for their helpful advice and supports on DFT calculations.
Notes and references
- M. Jaishankar, T. Tseten, N. Anbalagan, B. B. Mathew and K. N. Beeregowda, Interdiscip. Toxicol., 2014, 7, 60–72 Search PubMed.
- P. C. Nagajyoti, K. D. Lee and T. V. M. Sreekanth, Environ. Chem. Lett., 2010, 8, 199–216 CrossRef CAS.
- Z. Xu, S. J. Han, C. Lee, J. Yoon and D. R. Spring, Chem. Commun., 2010, 46, 1679–1681 RSC.
- G. Guzzi and C. A. M. La Porta, Toxicology, 2008, 244, 1–12 CrossRef CAS.
- O. R. Luca and A. Q. Fenwick, J. Photochem. Photobiol., B, 2015, 152, 26–42 CrossRef CAS.
- R. A. Bernhoft, J. Environ. Health, 2012, 2012, 460508 Search PubMed.
- K. M. Rice, E. M. Walker, Jr, M. Wu, C. Gillette and E. R. Blough, J. Prev. Med. Public Health, 2014, 47, 74–83 CrossRef.
- Y. Zhuang, J. S. Thompson, C. J. Zygarlicke and J. H. Pavlish, Environ. Sci. Technol., 2004, 38, 5803–5808 CrossRef CAS.
- I. R. Gould, J. R. Lenhard, A. A. Muenter, S. A. Godleski and S. Farid, J. Am. Chem. Soc., 2000, 122, 11934–11943 CrossRef CAS.
- W. Shen, J. Tang, Y. Wang, J. Liu, L. Huang, W. Chen, L. Yang, W. Wang, Y. Wang, R. Yang, J. Yun and L. A. Belfiore, ACS Appl. Mater. Interfaces, 2017, 9, 5358–5365 CrossRef CAS.
- S. Prabhu and E. K. Poulose, Int. Nano Lett., 2012, 2, 32 CrossRef.
- J. Hardes, H. Ahrens, C. Gebert, A. Streitbuerger, H. Buerger, M. Erren, A. Gunsel, C. Wedemeyer, G. Saxler, W. Winkelmann and G. Gosheger, Biomaterials, 2007, 28, 2869–2875 CrossRef CAS.
- M. B. Gumpu, S. Sethuraman, U. M. Krishnan and J. B. B. Rayappan, Sens. Actuators, B, 2015, 213, 515–533 CrossRef CAS.
- S.-j. Yu, Y.-g. Yin and J.-f. Liu, Environ. Sci.: Processes Impacts, 2013, 15, 78–92 RSC.
- B. C. Mondal, D. Das and A. K. Das, Anal. Chim. Acta, 2001, 450, 223–230 CrossRef CAS.
- Y.-T. Li, S.-J. Jiang and A. C. Sahayam, Food Anal. Method., 2017, 10, 434–441 CrossRef.
- M. Hosoba, K. Oshita, R. K. Katarina, T. Takayanagi, M. Oshima and S. Motomizu, Anal. Chim. Acta, 2009, 639, 51–56 CrossRef CAS.
- X.-B. Zhang, Z.-X. Han, Z.-H. Fang, G.-L. Shen and R.-Q. Yu, Anal. Chim. Acta, 2006, 562, 210–215 CrossRef CAS.
- A. Promchat, K. Wongravee, M. Sukwattanasinitt and T. Praneenararat, Sci. Rep., 2019, 9, 10390 CrossRef.
- A. Kathiravan, A. Gowri, T. Khamrang, M. D. Kumar, N. Dhenadhayalan, K.-C. Lin, M. Velusamy and M. Jaccob, Anal. Chem., 2019, 91, 13244–13250 CrossRef CAS.
- M. Saleem, M. Rafiq and M. Hanif, J. Fluoresc., 2017, 27, 31–58 CrossRef CAS.
- K. P. Carter, A. M. Young and A. E. Palmer, Chem. Rev., 2014, 114, 4564–4601 CrossRef CAS.
- S. Voutsadaki, G. K. Tsikalas, E. Klontzas, G. E. Froudakis and H. E. Katerinopoulos, Chem. Commun., 2010, 46, 3292–3294 RSC.
- L. Jin, M. Xu, H. Jiang, W. Wang and Q. Wang, Anal. Methods, 2018, 10, 4562–4569 RSC.
- K. Bera, A. K. Das, M. Nag and S. Basak, Anal. Chem., 2014, 86, 2740–2746 CrossRef CAS.
- Y. Liu, Z. Li, L. Chen and Z. Xie, Dyes Pigm., 2017, 141, 5–12 CrossRef CAS.
- G. Ulrich, R. Ziessel and A. Harriman, Angew. Chem., Int. Ed., 2008, 47, 1184–1201 CrossRef CAS.
- A. Loudet and K. Burgess, Chem. Rev., 2007, 107, 4891–4932 CrossRef CAS.
- K. Velmurugan, A. Raman, S. Easwaramoorthi and R. Nandhakumar, RSC Adv., 2014, 4, 35284–35289 RSC.
- H. Liu, Y. Tan, Q. Dai, H. Liang, J. Song, J. Qu and W.-Y. Wong, Dyes Pigm., 2018, 158, 312–318 CrossRef CAS.
- Q. Lai, Q. Liu, Y. He, K. Zhao, C. Wei, L. Wojtas, X. Shi and Z. Song, Org. Biomol. Chem., 2018, 16, 7801–7805 RSC.
- M. Vedamalai, D. Kedaria, R. Vasita, S. Mori and I. Gupta, Dalton Trans., 2016, 45, 2700–2708 RSC.
- J. J. Bryant and U. H. F. Bunz, Chem.–Asian J., 2013, 8, 1354–1367 CrossRef CAS.
- M. Zurro and O. G. Mancheño, Chem. Rec., 2017, 17, 485–498 CrossRef CAS.
- K. Rajavelu, M. Subaraja and P. Rajakumar, New J. Chem., 2018, 42, 3282–3292 RSC.
- A. I. Germeroth, J. R. Hanna, R. Karim, F. Kundel, J. Lowther, P. G. N. Neate, E. A. Blackburn, M. A. Wear, D. J. Campopiano and A. N. Hulme, Org. Biomol. Chem., 2013, 11, 7700–7704 RSC.
- Y. Ling, Z.-X. Chen, F.-P. Zhai, Y.-M. Zhou, L.-H. Weng and D.-Y. Zhao, Chem. Commun., 2011, 47, 7197–7199 RSC.
- R. S. Singh, R. K. Gupta, R. P. Paitandi, A. Misra and D. S. Pandey, New J. Chem., 2015, 39, 2233–2239 RSC.
- A. N. Kursunlu, M. Ozmen and E. Güler, J. Fluoresc., 2019, 29, 1321–1329 CrossRef CAS.
- A. N. Kursunlu, RSC Adv., 2015, 5, 41025–41032 RSC.
- A. N. Kursunlu and E. Güler, J. Mol. Struct., 2017, 1134, 345–349 CrossRef CAS.
- X. Liu, X. Yang, H. Peng, C. Zhu and Y. Cheng, Tetrahedron Lett., 2011, 52, 2295–2298 CrossRef CAS.
- S. Y. Lee, K. H. Bok and C. Kim, RSC Adv., 2017, 7, 290–299 RSC.
- S. Khatua and M. Schmittel, Org. Lett., 2013, 15, 4422–4425 CrossRef CAS.
- X. Zhang, Y. Xu, P. Guo and X. Qian, New J. Chem., 2012, 36, 1621–1625 RSC.
- J. H. Brannon and D. Magde, J. Phys. Chem., 1978, 82, 705–709 CrossRef CAS.
- F. Heisig, S. Gollos, S. J. Freudenthal, A. El-Tayeb, J. Iqbal and C. E. Müller, J. Fluoresc., 2014, 24, 213–230 CrossRef CAS.
- M. J. Frisch, G. W. Trucks, H. B. Schlegel, G. E. Scuseria, M. A. Robb, J. R. Cheeseman, G. Scalmani, V. Barone, B. Mennucci, G. A. Petersson, H. Nakatsuji, M. Caricato, X. Li, H. P. Hratchian, A. F. Izmaylov, J. Bloino, G. Zheng, J. L. Sonnenberg, M. Hada, M. Ehara, K. Toyota, R. Fukuda, J. Hasegawa, M. Ishida, T. Nakajima, Y. Honda, O. Kitao, H. Nakai, T. Vreven, J. A. Montgomery Jr., J. E. Peralta, F. Ogliaro, M. Bearpark, J. J. Heyd, E. Brothers, K. N. Kudin, V. N. Staroverov, T. Keith, R. Kobayashi, J. Normand, K. Raghavachari, A. Rendell, J. C. Burant, S. S. Iyengar, J. Tomasi, M. Cossi, N. Rega, J. M. Millam, M. Klene, J. E. Knox, J. B. Cross, V. Bakken, C. Adamo, J. Jaramillo, R. Gomperts, R. E. Stratmann, O. Yazyev, A. J. Austin, R. Cammi, C. Pomelli, J. W. Ochterski, R. L. Martin, K. Morokuma, V. G. Zakrzewski, G. A. Voth, P. Salvador, J. J. Dannenberg, S. Dapprich, A. D. Daniels, Ö. Farkas, J. B. Foresman, J. V. Ortiz, J. Cioslowski and D. J. Fox, Gaussian 09, Revision C.01, Gaussian, Inc., Wallingford CT, 2010 Search PubMed.
- C. Lee, W. Yang and R. G. Parr, Phys. Rev. B: Condens. Matter Mater. Phys., 1988, 37, 785–789 CrossRef CAS.
- A. D. Becke, J. Chem. Phys., 1993, 98, 5648–5652 CrossRef CAS.
- P. C. Hariharan and J. A. Pople, Theor. Chim. Acta, 1973, 28, 213–222 CrossRef CAS.
- G. A. Petersson, A. Bennett, T. G. Tensfeldt, M. A. Al-Laham, W. A. Shirley and J. Mantzaris, J. Chem. Phys., 1988, 89, 2193–2218 CrossRef CAS.
- P. J. Hay and W. R. Wadt, J. Chem. Phys., 1985, 82, 270–283 CrossRef CAS.
- W. R. Wadt and P. J. Hay, J. Chem. Phys., 1985, 82, 284–298 CrossRef CAS.
- P. J. Hay and W. R. Wadt, J. Chem. Phys., 1985, 82, 299–310 CrossRef CAS.
- T. Yanai, D. P. Tew and N. C. Handy, Chem. Phys. Lett., 2004, 393, 51–57 CrossRef CAS.
- V. Barone and M. Cossi, J. Phys. Chem. A, 1998, 102, 1995–2001 CrossRef CAS.
- M. Cossi, N. Rega, G. Scalmani and V. Barone, J. Comput. Chem., 2003, 24, 669–681 CrossRef CAS.
- Avogadro: an open-source molecular builder and visualization tool. Version 1.2.0, http://avogadro.cc/ Search PubMed.
- M. D. Hanwell, D. E. Curtis, D. C. Lonie, T. Vandermeersch, E. Zurek and G. R. Hutchison, J. Cheminf., 2012, 4, 17 CAS.
- H. Lu, J. Mack, Y. Yang and Z. Shen, Chem. Soc. Rev., 2014, 43, 4778–4823 RSC.
- I. T. Ho, T.-L. Lai, R.-T. Wu, M.-T. Tsai, C.-M. Wu, G.-H. Lee and W.-S. Chung, Analyst, 2012, 137, 5770–5776 RSC.
- D.-T. Shi, X.-L. Wei, Y. Sheng, Y. Zang, X.-P. He, J. Xie, G. Liu, Y. Tang, J. Li and G.-R. Chen, Sci. Rep., 2014, 4, 4252 CrossRef.
- Z. Shi, X. Han, W. Hu, H. Bai, B. Peng, L. Ji, Q. Fan, L. Li and W. Huang, Chem. Soc. Rev., 2020, 49, 7533–7567 RSC.
- H.-C. Hung, C.-W. Cheng, Y.-Y. Wang, Y.-J. Chen and W.-S. Chung, Eur. J. Org. Chem., 2009, 2009, 6360–6366 CrossRef.
- J. Fan, C. Chen, Q. Lin and N. Fu, Sens. Actuators, B, 2012, 173, 874–881 CrossRef CAS.
- W. Shi, Y. Chen, X. Chen, Z. Xie and Y. Hui, J. Lumin., 2016, 174, 56–62 CrossRef CAS.
- B. Sen, M. Mukherjee, S. Banerjee, S. Pal and P. Chattopadhyay, Dalton Trans., 2015, 44, 8708–8717 RSC.
- V. S. Jisha, A. J. Thomas and D. Ramaiah, J. Org. Chem., 2009, 74, 6667–6673 CrossRef CAS.
- Y. Zhang, X. Li, L. Gao, J. Qiu, L. Heng, B. Z. Tang and L. Jiang, ChemPhysChem, 2014, 15, 507–513 CrossRef CAS.
- D. C. Harris, Quantitative Chemical Analysis, W.H. Freeman and Company, New York, 2010 Search PubMed.
- J. Kronenbitter, U. Schweizer and A. Schwenk, Z. Naturforsch., 1980, 35, 319 Search PubMed.
- R. E. Wasylishen, R. E. Lenkinski and C. Rodger, Can. J. Chem., 1982, 60, 2113–2117 CrossRef CAS.
- M. Maliarik and I. Persson, Magn. Reson. Chem., 2005, 43, 835–842 CrossRef CAS.
Footnote |
† Electronic supplementary information (ESI) available. See DOI: 10.1039/d0ra09686e |
|
This journal is © The Royal Society of Chemistry 2021 |
Click here to see how this site uses Cookies. View our privacy policy here.