DOI:
10.1039/D1RA00587A
(Paper)
RSC Adv., 2021,
11, 14607-14614
A new donor for charge-transfer systems: synthesis and properties of 2,4,7,9-tetramethyl-1,6-dithiapyrene (TMDTP) and structure of (TMDTP)3(PF6)2·2THF and TMDTP–TCNQ†
Received
22nd January 2021
, Accepted 30th March 2021
First published on 20th April 2021
Abstract
The heterocyclic donor molecule 2,4,7,9-tetramethyl-1,6-dithiapyrene (TMDTP) has been synthesized in five steps. Oxidation of TMDTP is facile (E11/2 = 0.27 V and E21/2 = 0.79 V vs. SCE). The charge-transfer complex, TMDTP–TCNQ, has been prepared and the salt, (TMDTP)3(PF6)2·2THF, obtained by electrocrystallization. The structure of TMDTP, TMDTP–TCNQ and (TMDTP)3(PF6)2·2THF has been characterized by X-ray crystallography and computationally.
Introduction
Polycyclic aromatic compounds are interesting in materials science for applications in organic electronics. Some of the earliest known organic semiconductors were radical cation salts of pyrene and perylene1 formed by reaction with bromine. The high oxidation potentials of most polycyclic aromatic compounds make them less attractive for applications due to the high reactivity of the corresponding radical cations. However, they can still be highly useful in devices like OLEDs and FETs.2,3 Recently, radical cation salts have also gained interest because some of them have a high thermoelectric effect making them highly interesting for small generators of electricity.4
Having large conjugated π-systems in conducting organic materials is advantageous because the transport of charge takes place through the stacked molecules and having a large contact surface should lead to smaller on-site electron to electron repulsion and a higher transfer integral in terms of the Hubbard-model.5 Furthermore, the hydrogen atoms on the periphery of a polycyclic aromatic compound can be substituted with groups that can be used to engineer the packing in the solid state and to influence the redox potentials. Substitution of CH-groups in a polycyclic aromatic compound like pyrene with chalcogen atoms lead to systems, where the dications are isoelectronic with pyrene itself (Fig. 1) and the corresponding oxidation potentials are therefore expected to be lower leading to more stable materials.
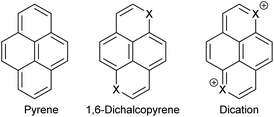 |
| Fig. 1 Pyrene (16 π system), a 1,6-dichalcopyrene (18 π system) and the corresponding dichalcopyrene dication (16 π system). | |
The effect of substituting CH-groups with chalcogens has been a topic of interest for a long time. The discovery of the first organic metal TTF-TCNQ in 1973 led to an interest in radical cation salts and the discovery of first organic superconductors based on tetramethyltetraselenafulvalene (TMTSF) (TMTSF)2PF6 (superconducting at 0.9 K and 12 kbar)6] and (TMTSF)2ClO4 (superconducting at 1.3 K and atmospheric pressure).7 The corresponding sulphur analogue (tetramethyltetrathiafulvalene) forms a series of isostructural radical cation salts, but none becomes superconducting. In fact, the Bechgaard-salts have a rich phase diagram, where the solid-state properties depend on the anion, temperature, pressure, magnetic and electric fields.8 Replacement of sulphur and selenium with the other group 16 elements tellurium and oxygen was a holy grail for many years, and although the first tetratellurofulvalene was synthesized in 1982, it has turned out to be very difficult to obtain any crystalline molecular solids allowing for comparison of properties. Tetraoxafulvalene itself is still unknown and the closest derivatives are the dibenzo- and dinaphtotetraoxafulvalenes,9 dibenzotrioxathio-,10the unsymmetrical dibenzodithiafulvalene11 and dibenzodiselenadioxafulvalene.12 A crystalline 1
:
1 charge transfer complex between trans-dibenzodithiadioxafulvalene and TCNQ has been reported as being an insulator but no crystal structure was described.11
The groups of Bechgaard13–15 and Nakasuji16–29 have reported conducting molecular solids based on 1,6-dithiapyrene (1), which was originally synthesized by Tilak and coworkers.30,31 This led to the question if it would be possible to use the pyrene π-frame as a test tube for investigating the effect of the heteroatom on the conducting materials properties. Since it is not possible to synthesize 1,6-dioxapyrenes by the same methodology32 (Scheme 1), different methodologies have been developed based either on 2,6-dialkyl-1,5-naphthalenediols as starting materials33,34 or on using 1,4,5,8-tetrasubstituted naphthalenes as intermediates35–38 but both strategies have their limitations.
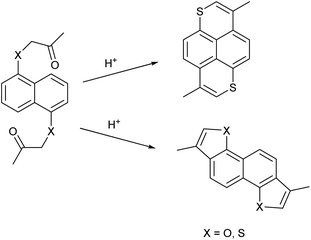 |
| Scheme 1 The different regioselectivities in acid catalyzed cyclizations of 1,1′-(naphthalene-1,5-diylbis(oxy))bis(propan-2-one) and 1,1′-(naphthalene-1,5-diylbis(sulfanediyl))bis(propan-2-one). | |
So far only a few 1,6-dioxapyrenes have given well-defined charge transfer complexes or radical cations salts, where it has been possible to get a crystal structure. The complex between 2,7-dimethyl-4,9-diethyl-1,6-dioxapyrene and TCNQ is a mixed stack and therefore insulating.39,40 Conversely, 2,4,7,9-tetramethyl-1,6-dioxapyrene (TMDOP) was found to form a series of isostructural salts of stoichiometry (TMDOP)2X,33 where X− is BF4−, PF6− and AsF6− with homogeneous donor stacking (Fig. 2).
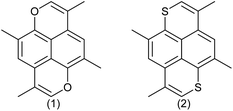 |
| Fig. 2 Tetramethyldioxapyrene (TMDOP) (1) and tetramethyldithiapyrene (TMDTP) (2). | |
The crystal structure of these compounds reveal the donor molecules packing in a centrosymmetric arrangement with regular stacking in the z-direction, but unfortunately with the TMDOP-molecules rotated in the x–y plane minimizing the molecular overlap in the stacks40,41 and the salts are semiconducting.42 The discovery of the (TMDOP)2X series led us to synthesize the corresponding sulphur analogue 2,4,7,9-tetramethyl-1,6-dithiapyrene (TMDTP) hoping that it would be possible to obtain an isostructural radical cation salt in order to compare the influence of the heteroatom on the properties. A previous communication42 reported metallic conductivity of the salt (TMDTP)2AsF6 down to 75 K, where charge localization accompanied a metal to insulator transition. The crystals were unfortunately too disordered to obtain a crystal structure.42 The present work reports on the synthesis and properties of TMDTP, the structure of a 1
:
1 TCNQ-complex (8) and the new radical cation salt (TMDTP)2AsF6 (9) as well as a comparison of the physical properties with the corresponding oxygen analogue TMDOP.
Results and discussion
Synthesis
The synthesis is based on our previous work on 2,6-disubstituted-1,6-dioxapyrenes,33,34 where the common intermediates are 2,6-disubstituted-1,5-naphthalenediols. Here the analogous intermediate would be 2,6-dimethyl-1,5-napthalenedithiol (6), which is convenient for synthesis of other derivatives for a more thorough comparison between 1,6-dithia- and 1,6-dioxapyrenes. 3,5,8,10-Tetramethyl-1,6-dithiapyrene (2) was synthesized as shown in Scheme 2 from a mixture of 2,6-dimethylnaphthalene and 2,7-dimethylnaphthalene (easily obtainable by crystallization of commercial dimethylnaphthalene/ethylnaphtalene mixture). Reaction with bromine and a catalytic amount of AlCl3 in CH2Cl2 gave pure 1,5-dibromo-2,6-dimethylnaphthalene (4) crystallizing from the reaction mixture. Transmetallation with n-BuLi in THF at −78 °C gave 1,5-dilithio-2,6-dimethylnaphthalene, which was trapped with dimethyldisulfide to give the sulphide (5). Reductive cleavage with sodium in pyridine gave the dithiol (6), which was alkylated with chloropropanone in DMF with K2CO3 as base. The ketosulfide (7) was cyclized to yield TMDTP (2) with CH3SO3H in CH2Cl2.
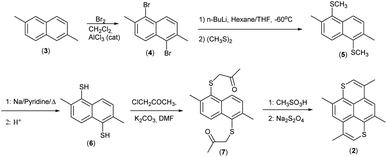 |
| Scheme 2 Synthesis of 2. | |
Crystals of the TCNQ-complex (8) were grown by diffusion in a H-tube using acetonitrile as the solvent. The stoichiometry was determined by elemental analysis and confirmed by X-ray crystallography.
Crystals of the (TMDTP)3(PF6)2·2THF (9) were grown by electrocrystallization of a solution of TMDTP in THF with Bu4NPF6 as electrolyte.
Comparison between TMDOP and TMDTP
The oxidation potentials for the two compounds were measured by cyclic voltammetry and are shown in Table 1.
Table 1 Electrochemical dataa
Compound |
E11/2, V |
E21/2, V |
ΔE, V |
Potentials were measured at a scan rate of 100 mV s−1 using Pt versus SCE in 0.1 M Bu4NPF6/CH2Cl2. |
TMDOP33 |
0.32 |
1.00 |
0.68 |
TMDTP |
0.27 |
0.79 |
0.52 |
The first oxidation potential of TMDTP is slightly lower than that for TMDOP, but the second oxidation potential is significantly lower for TMDTP than for TMDOP and the difference ΔE suggests that the on-site electron repulsion (U in the Hubbard model) is lower for TMDP than for TMDOP.
Structural characterization
Crystal structures of TMDTP, TMDTP–TCNQ (8), and (TMDTP)3(PF6)2·2THF (9) were determined by single crystal X-ray diffraction at 100 K. TMDTP crystallizes in the triclinic group P
with TMDTP at inversion centers. The normal to the TMDTP planes makes an angle of ca. 21.8° with the crystallographic a-axis and a TMDTP interplane distance at 3.515 Å, accordingly shorter than the unit cell a-axis (3.962 Å). The molecular structure including bond length metrics is shown in Fig. 3.
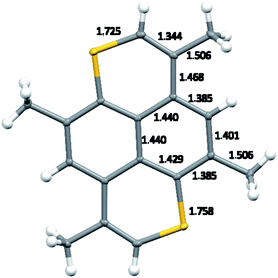 |
| Fig. 3 Molecular structure of TMDTP including bond lengths in the asymmetric unit. Thermal ellipsoid probabilities are drawn at 50%. | |
Crystals of TMDTP–TCNQ (8) also belong to P
with TMDTP at inversion centers. In addition TCNQ units are at general positions. The packing is shown in Fig. 4 and features a stack of TCNQ and two different stacks of TMDTP. One stack (a) is close to aligned with the TCNQ stack: ∠TCNQ–TMDTPa = 13.94° whereas the second TMDTP stack (b) is significantly inclined with respect to the TCNQ stack: ∠TCNQ–TMDTPb = 50.92°. The angle between the two TMDTP stacks amounts to 36.98°. The interlayer distances in the three stacks are TCNQ: 3.132 Å; TMDTPa: 3.538 Å; TMDTPb: 3.531 Å. From the metrics of the TCNQ moiety, an analysis of the extent of charge transfer can be made based on the modeling by Coppens et.al.43 For TCNQ–TMDTP the distance based model of ref. 45, based on the full dataset yield q = −0.69. This is close to the calculated charge transfer, q = −0.70(3), based on the same model for monomethylmorpholinium (TCNQ)2, but significantly larger the charge transfer for tetramethyltetraselenofulvalene (red form, q = −0.27(6)), testifying to the donor capabilities of TMDTP.
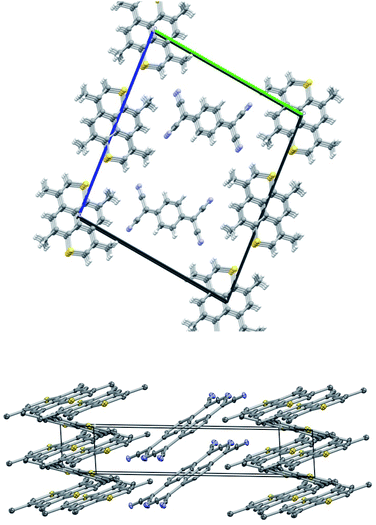 |
| Fig. 4 Crystal packing of TMDTP·TCNQ. Top view approx. along a-axis. Bottom view approx. along c-axis. Thermal ellipsoid probabilities are drawn at 50%. The asymmetric unit comprises half a molecule of TMDTP from each of the two differently inclined stacks and a full TCNQ unit. Hydrogens are omitted for clarity. Color coding: carbon: grey; sulfur: yellow nitrogen: blue. | |
A needle-shaped crystal of TMDTP electrocrystallized with PF6− as counter ion in a 3
:
2 stoichiometry was investigated by single crystal X-ray diffraction. The compound crystallizes in space group P
with 1/3's of TMDTP cations at inversion centers and all other moieties at general positions. The packing consists of TMDTP cation stacks, with interlayer spacing of 3.608 Å running along the crystallographic a-direction (cf. Fig. 5 top panel). Separating the TMDTP stacks are columns of alternating THF molecules and PF6− anions (Fig. 5 bottom panel). By symmetry the closest spacings between the TMDTP stacks equals the crystallographic b = 11.925(4) Å and c = 12.194(4) Å dimensions.
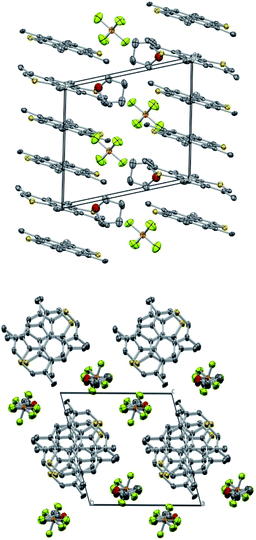 |
| Fig. 5 Crystal packing of (TMDTP)3(PF6)2·2THF. Top view approx. along c-axis. Bottom view approx. along a-axis. Thermal ellipsoid probabilities are drawn at 40%. The asymmetric unit comprises 1.5 molecule of TMDTP, one PF6− ion and one THF molecule. Hydrogens are omitted for clarity. Color coding: carbon: grey; phosphorous: orange; oxygen: red; fluorine: lime. | |
Along the TMDTP stacks the molecular orientation alternate between layers, but neighbouring sulfur atoms remain in quite close proximity (3.841 Å/3.912 Å). Both the stoichiometry and the metrics of the TMDTPs indicate that the two moieties with fractional coordinates a = 1/3 and a = 2/3 are oxidized with average C–S bond lengths 1.710 Å while moieties with a = 0 are neutral (average C–S bond length = 1.747 Å). The difference upon oxidation matches quite closely the computed average change of −0.030 Å upon oxidation (vide infra).
Discussion
The degree of charge transfer in TCNQ–TMDTP inferred by analysis of the TCNQ geometry (vide supra) according to Coppens and coworkers,43 is further corroborated by comparison of the structures of the TMDTP entities between the TCNQ–TMDTP and the (TMDTP)3(PF6)2·2THF structures. The stoichiometry of the latter compound together with the model derived charge of q = +0.69 for TMDTP in TCNQ–TMDTP suggest very similar average oxidation states of TMDTP in the two structures. Indeed, a structure overlay of TMDTP from both compounds reveal almost perfectly superimposable geometries (cf. Fig. 6) with only very minor differences (<0.02 Å) in bond lengths and planarity (<0.14 Å) mainly around the sulfur atoms.
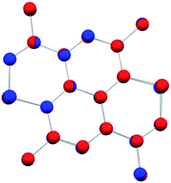 |
| Fig. 6 Overlay of TMDTP moieties from TCNQ–TMDTP (blue) and (TMDTP)3(PF6)2·2THF (red). The rms deviation on atom positions is 0.016 Å. | |
The changes in geometric and electronic structure associated with the oxidation of TMDTP was evaluated computationally using DFT. Frontier orbitals for the neutral molecule are shown in Fig. 7. As for the parent hydrocarbon,44 the computed HOMO is located at the periphery of the molecule with approximately equal amplitude at carbon atoms and sulphur atoms. Conversely, the LUMO extends over also the central carbon atoms in the pyrene framework.
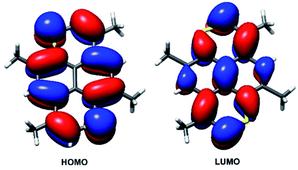 |
| Fig. 7 DFT computed frontier orbitals for neutral TMDTP. Restricted computation employing the B3LYP functional and a TZVPP basis on all atoms. Contours are draw at (±0.027). | |
Upon oxidation the resulting SOMO resembles the HOMO of the neutral molecule as witnessed by the spin density distribution (Fig. 8).
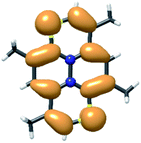 |
| Fig. 8 Spin density distribution from unrestricted DFT (ochre = majority; blue = minority). Contours are draw at (±0.001). | |
Geometry optimized bond length differences of the TMDTP and its cation are depicted in Fig. 9. The computed changes in geometry reflect the peripheral and very delocalized nature of the MO involved in the redox process. Accordingly, the charge distribution is also very even with the highest computed positive atomic charges being as low as +0.14 (on hydrogens) and only +0.11 on the sulphur atoms, which harbours the highest spin density (+0.18).
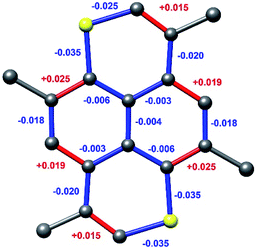 |
| Fig. 9 Changes in bond lengths between geometry optimized structures of TMDTP/TMDTP+. Differences are changes upon oxidation. | |
Experimental
TMDTP (2)
1,1′-((2,6-Dimethylnaphthalene-1,5-diyl)bis(sulfanediyl))-bis(propan-2-one) (7) (1.4 g; 4.2 mmol) was dissolved in CH2Cl2 (10 mL). After addition of CH3SO3H (4 mL), the reaction mixture was stirred overnight and poured into a cold solution of NaS2O4 (10 g; 57 mmol) in 2 M NaOH (100 mL). The crude product was extracted with CH2Cl2 (500 mL), dried over Na2SO4 and filtered. The solvent was removed and the product purified by column chromatography on silica gel 60 (0.040–0.063 mm) with toluene as eluent. Yield: 0.28 g (19%) after crystallization from toluene. Mp 206–208 °C
1H-NMR (500 MHz, CS2, CDCl3-lock tube): δ: 6.51 (s, 2H); 5.85 (s, 2H); 2.05 (s, 6H); 1.99 (s, 6H).
13C-NMR (125 MHz, CS2, CDCl3-lock tube): δ: 132.05; 129.10; 128.99; 128.66; 127.33; 122.76; 117.64; 22.64; 20.25.
EI-MS: m/z: 296.07 (100.0%), 297.07 (19.5%), 298.07 (9.0%), 298.08 (1.8%), 297.07 (1.6%)
Elemental analysis: calcd for C18H16S2: C, 72.93%; H, 5.44%; S, 21.63%.
Found: C, 73.14%; H, 5.50%; S: 21.66%.
Cyclic voltammetry (0.1 M Bu4N+PF6− in CH2Cl2, rate: 100 mV s−1, Pt vs. SCE): E11/2: 0.27 V; E21/2: 0.79 V.
2,6-/2,7-Dimethylnapthalene mixture (3)
Dimethylnapthalene, mixture of isomers and ethylnaphthalenes (2.5 L) was mixed with EtOH (2.5 L) and cooled to −20 °C for 24 h. The crystalline material was isolated by filtration and air dried to give 476 g of crude material, which was recrystallized from EtOH (3.5 L), cooling to −20 °C, filtered and air dried to give 235 g of 2,6-/2,7-dimethylnaphthalene eutectic mixture. The mixture consists of 58% 2,6-dimethylnaphthalene and 42% 2,7-dimethylnaphthalene as determined by 1H-NMR (see ESI†).
2,6-Dimethyl-1,5-dibromonapthalene (4)
Bromine (6.6 mL; 20.5 g; 0.26 mol) dissolved in CH2Cl2 (50 mL) was added over 25 minutes to a stirred solution of (3) (10.0 g; 64 mmol) in CH2Cl2 (50 mL) containing a catalytic amount of AlCl3. Stirring overnight followed by removal of the solvent in vacuo. The residue was dissolved in ligroin (bp 100–140 °C, 100 mL), treated with activated carbon and cooled to +5 °C overnight. Yield: 6.7 g (57% based on 2,6-dimethylnaphhalene). Mp 151–153 °C (151–153 °C (ref. 45)).
1H-NMR (500 MHz, CDCl3): δ 8.09 (d, J = 5 Hz, 2H); 7.31 (d, J = 5 Hz, 2H); 2.52 (s, 6H).
13C-NMR (126 MHz, CDCl3): δ 135.88; 131.94; 129.78; 126.35; 123.95; 24.01.
GC-MS: 314 (M+).
1,5-Dimethylmercapto-2,6-dimethylnaphthalene (5)
1,5-Dibromo-2,6-dimethylnaphthalene (4) (5.1 g; 16 mmol) was dissolved in dry THF (80 mL) and cooled to −60 °C. A solution of n-BuLi in hexane (2.5 M; 13 mL; 32.5 mmol) was added over 10 minutes with stirring. After further 20 min at −60 °C, dimethyldisulfide (3.5 mL; 39 mmol) was added dropwise (exothermic reaction). The cooling bath was removed and when the reaction mixture reached room temperature, it was poured into water (200 mL), extracted with ether, dried over MgSO4, filtered and concentrated in vacuo. The residual oil was crystallized from absolute ethanol to give pure 5. Yield: 2.5 g (62%). Mp 101–103 °C
1H-NMR (500 MHz, CDCl3) δ: 8.56 (d, J = 10 Hz, 2H); 7.40 (d, J = 10 Hz, 2H); 2.69 (s, 6H); 2.21 (s, 6H).
13C-NMR (126 MHz, CDCl3) δ: 140.25; 134.48; 132.43; 129.55; 127.02; 21.82; 19.21.
GC-MS: 248.2 (M+).
Elemental analysis:
Calcd for C14H16S2: C, 67.69%; H, 6.49%.
Found: C, 66.30%; H, 6.31%.
2,6-Dimethylnaphthalene-1,5-dithiol (6)
1,5-Dimethylmercapto-2,6-dimethylnaphthalene (5) (5.0 g; 20 mmol) was dissolved in pyridine (50 mL) under a N2-atmosphere. Sodium cut in small pieces (1.9 g; 83 mmol) was added with stirring. The reaction mixture was heated to +100 °C overnight. After cooling to room temperature, the residues of sodium were carefully destroyed with abs. EtOH and the reaction mixture was poured into 2 M H2SO4 (500 mL). The product was extracted with diethyl ether, dried over Na2SO4, filtered, concentrated in vacuo followed by crystallization from toluene (cooling to −20 °C) to give 1.85 g (42%). Pale yellow material. Mp: 136–138 °C
Elemental analysis:
Calcd for C12H12S2: C, 65.41; H, 5.49; S, 29.10.
Found: C, 65.70; H, 5.47; S, 28.65.
1H-NMR (500 MHz, CDCl3): δ: 8.015 (d, J = 5 Hz, 2H); 7.31 (d, J = 5 Hz, 2H); 3.32 (s, 2H); 2.51 (s, 6H).
13C-NMR (126 MHz, CDCl3): δ: 134.42; 132.03; 129.20; 127.26; 123.08; 22.42.
ESI-MS: 220 (M+).
1,1′-((2,6-Dimethylnaphthalene-1,5-diyl)bis(sulfanediyl))bis(propan-2-one) (7)
2,6-Dimethylnaphthalene-1,5-dithiol (6) (1.1 g; 5 mmol) was dissolved in a stirred, degassed mixture of K2CO3 (2 g; 15 mmol) and DMF (20 mL). 1-Chloro-2-propanone (1.5 mL; 1.7 g; 18 mmol) was added. The reaction mixture was stirred for 2 hours at room temperature, poured into water (200 mL) and extracted with diethyl ether. Drying over Na2SO4, filtration and removal of the solvent followed by column chromatography on Silicagel 60 (0.040–0.063 mm) with ethyl acetate/heptane (1
:
1). After crystallization from ethyl acetate, pale yellow crystals 2.0 g (66%). Mp 139–141 °C.
1H-NMR (500 MHz, CDCl3) δ: 8.50 (d, J = 10 Hz, 2H); 7.40 (d, J = 10 Hz, 2H); 3.43 (s, 4H); 2.67 (s, 6H); 2.09 (s, 6H).
13C-NMR (126 MHz, CDCl3) δ: 202.90; 141.47; 134.55; 129.88; 129.36; 127.39; 45.93; 28.74; 22.07.
EI-MS: m/z: 332.09 (100.0%), 333.09 (19.5%), 334.09 (9.0%), 334.10 (1.8%), 335.09 (1.8%), 333.09 (1.6%)
Elemental analysis: calcd For C18H20O2S2: C, 65.03; H, 6.06. Found: C, 65.05; H, 6.20.
TMDTP–TCNQ (8)
Was grown by diffusion in a H-cell from saturated solutions of TMDTP (2) and TCNQ.
(TMDTP)3(PF6)2·2THF (9)
A solution of TMDTP (50 mg; 0.17 mmol) in dry THF (50 mL) containing Bu4NPF6 (0.5 g; 1,3 mmol) was electrocrystallized at room temperature with Pt-electrodes at a constant current of 2 μA for 7 days.
Crystallography
The X-ray crystallographic studies. The X-ray crystallographic studies were carried out on single crystals, which were coated with mineral oil, mounted on kapton loops, and transferred to the nitrogen cold stream of the diffractometer. The single-crystal X-ray diffraction studies were performed at 100(2) K on a Bruker D8 VENTURE diffractometer equipped with a Mo Kα high-brilliance IμS radiation source (λ = 0.71073 Å), a multilayer X-ray mirror and a PHOTON 100 CMOS detector, and an Oxford Cryosystems low temperature device. The instrument was controlled with the APEX3 software package using SAINT.46 Final cell constants were obtained from least squares fits of several thousand strong reflections. Intensity data were corrected for absorption using intensities of redundant reflections with the program SADABS.47 The structures were solved in Olex2 using SHELXT and refined using SHELXL.48 The crystals of (TMDTP)3(PF6)2·2THF were all poorly diffracting and the reported resolution of 0.95 Å was the best achievable. Crystallographic details are listed in Table 2. CCDC entries 2051961, 2051922, and 2051918 contain the crystallographic data reported herein.†
Table 2 Crystallographic data
Compound |
TMTDP |
TMDTP·TCNQ |
(TMDTP)3(PF6)2·2THF |
CCDC entry |
2051961 |
2051922 |
2051918 |
Empirical formula |
C18H16S2 |
C18H16S·C12H4N4 |
(C18H16S2)3·(PF6)2·(C4H8O)2 |
Formula weight |
296.42 |
500.62 |
1323.43 |
T/K |
100(2) |
100(2) |
100(2) |
Crystal system |
Triclinic |
Triclinic |
Triclinic |
Space group |
P![[1 with combining macron]](https://www.rsc.org/images/entities/char_0031_0304.gif) |
P![[1 with combining macron]](https://www.rsc.org/images/entities/char_0031_0304.gif) |
P![[1 with combining macron]](https://www.rsc.org/images/entities/char_0031_0304.gif) |
a/Å |
3.9618(4) |
3.7098(3) |
10.825(4) |
b/Å |
9.2110(8) |
17.1976(18) |
11.925(4) |
c/Å |
9.8478(8) |
20.181(2) |
12.194(4) |
α/° |
105.537(3) |
82.379(4) |
71.857(10) |
β/° |
90.439(3) |
89.939(3) |
77.095(10) |
γ/° |
96.653(3) |
86.046(4) |
72.995(10) |
V/Å3 |
343.62(5) |
1273.1(2) |
1415.2(9) |
Z |
1 |
2 |
1 |
ρcalc/g cm−3 |
1.432 |
1.306 |
1.553 |
μ/mm−1 |
0.373 |
0.24 |
0.385 |
2θ range/° |
4.3–50.5 |
4.8–56.4 |
4.7–43.7 |
Reflections collected |
14 877 |
69 129 |
13 127 |
Independent reflections |
2736 [Rint = 0.050] |
5812 [Rint = 0.071] |
3278 [Rint = 0.084] |
Data/restraints/parameters |
2736/0/93 |
5812/0/330 |
3278/138/385 |
Goodness-of-fit on F2 |
1.078 |
1.130 |
1.209 |
Final R indexes [I ≥ 2σ(I)] |
R1 = 0.033, wR2 = 0.0838 |
R1 = 0.096, wR2 = 0.245 |
R1 = 0.139, wR2 = 0.382 |
Largest diff. Peak/hole/e Å−3 |
0.55/–0.31 |
1.34/−0.58 |
1.03/−0.66 |
Computations
DFT calculations were carried out with the ORCA 4.2.1 program Tight convergence criteria were chosen for all calculations (Keywords TightSCF and TightOpt). The B3LYP functional was employed in conjunction with the def2-TZVPP basis set for both geometry optimizations and single point calculations. Orbital and spin density visualizations were done using USCF Chimera ver. 1.13.1.49
Conclusions
A synthesis of 2,4,7,9-tetramethyl-1,6-dithiapyrene (TMDTP) has been developed in order to compare the properties with the corresponding 2,4,7,9-tetramethyl-1,6-dioxapyrene (TMDOP), which is known to form a series of isostructural radical cations salts of the type (TMDOP)2X. Replacement of oxygen with sulphur leads to a more promiscuous donor molecule, which forms radical cation salts including crystal solvent molecules that tend to be disordered. The new salt (TMDTP)3(PF6)2·2THF and a 1
:
1 TCNQ-complex were prepared and their structures determined. Structural and computational characterization suggests a closely similar average oxidation state of ∼+2/3 for the TMDTP moieties. The TCNQ-complex consists of segregated stacks in contrast to the known TCNQ-complexes with 1,6-dioxapyrenes. The electrochemical data on TMDTP compared to TMDOP suggests that substitution of two CH-groups in pyrene with sulphur instead of oxygen leads to a better donor-molecule and could lead to new interesting materials.
Conflicts of interest
There are no conflicts to declare.
Notes and references
- H. Inokuchi, Int. Rev. Phys. Chem., 1989, 8, 95–124 Search PubMed.
- D. Shukla, T. R. Welter, D. R. Robello, D. J. Giesen, J. R. Lenhard, W. G. Ahearn, D. M. Meyer and M. Rajeswaran, J. Phys. Chem. C, 2009, 113, 14482–14486 CrossRef CAS.
- D. Shukla, T. R. Welter, A. L. Carroll-Lee, W. G. Ahearn and D. R. Robello, US pat., 20090001353A1, 2009 Search PubMed.
- Y. Kiyota, T. Kawamoto and T. Mori, Synth. Met., 2020, 259, 116217 CrossRef CAS.
- R. Hoffmann, Angew. Chem., Int. Ed. Engl., 1987, 26, 846–878 CrossRef.
- D. Jerome, A. Mazaud, M. Ribault and K. Bechgaard, J. Phys., Lett., 1980, 41, L95–L98 CrossRef.
- S. S. P. Parkin, M. Ribault, D. Jerome and K. Bechgaard, J. Phys. C: Solid State Phys., 1981, 14, 5305–5326 CrossRef CAS.
- W. Kang, S. T. Hannahs and P. M. Chaikin, Phys. Rev. Lett., 1993, 70, 3091–3094 CrossRef CAS PubMed.
- K. Tanaka, K. Yoshida, T. Ishida, A. Kobayashi and T. Nogami, Adv. Mater., 2000, 12, 661–664 CrossRef CAS.
- S. T. Darcangelis and D. O. Cowan, Tetrahedron Lett., 1996, 37, 2931–2934 CrossRef CAS.
- T. Nogami, K. Tanaka, T. Ishida and A. Kobayashi, Synth. Met., 2001, 120, 755–756 CrossRef CAS.
- T. Kojima, K. Tanaka, T. Ishida and T. Nogami, J. Org. Chem., 2004, 69, 9319–9322 CrossRef CAS PubMed.
- K. Bechgaard, Mol. Cryst. Liq. Cryst., 1985, 125, 81–89 CrossRef CAS.
- N. Thorup, G. Rindorf, C. S. Jacobsen, K. Bechgaard, I. Johannsen and K. Mortensen, Mol. Cryst. Liq. Cryst., 1985, 120, 349–352 CrossRef CAS.
- K. Bechgaard, I. Johannsen, M. Joergensen, C. S. Jacobsen, G. Rindorf, N. Thorup and K. Mortensen, Stud. Surf. Sci. Catal., 1986, 25, 391–402 Search PubMed.
- I. Murata and K. Nakasuji, Stud. Org. Chem., 1986, 25, 335–348 CAS.
- K. Nakasuji, H. Kubota, T. Kotani, I. Murata, G. Saito, T. Enoki, K. Imaeda, H. Inokuchi, M. Honda and a. et, J. Am. Chem. Soc., 1986, 108, 3460–3466 CrossRef CAS.
- F. Gerson, G. Gescheidt, J. Knoebel, I. Murata and K. Nakasuji, Helv. Chim. Acta, 1987, 70, 2065–2072 CrossRef CAS.
- K. Nakasuji, M. Sasaki, T. Kotani, I. Murata, T. Enoki, K. Imaeda, H. Inokuchi, A. Kawamoto and J. Tanaka, J. Am. Chem. Soc., 1987, 109, 6970–6975 CrossRef CAS.
- K. Nakasuji, T. Kotani, I. Murata, A. Kawamoto, J. Tanaka, T. Enoki, K. Imaeda and H. Inokuchi, Synth. Met., 1987, 19, 385–388 CrossRef CAS.
- A. Kawamoto, J. Tanaka, A. Oda, H. Mizumura, I. Murata and K. Nakasuji, Bull. Chem. Soc. Jpn., 1990, 63, 2137–2145 CrossRef CAS.
- K. Nakasuji, J. Toyoda, K. Imaeda, H. Inokuchi, I. Murata, A. Oda, A. Kawamoto and J. Tanaka, Synth. Met., 1991, 42, 2529–2534 CrossRef CAS.
- K. Yamaguchi, M. Okumura, T. Fueno and K. Nakasuji, Synth. Met., 1991, 43, 3631–3634 CrossRef CAS.
- Y. Morita, E. Miyazaki, S. Maki, J. Toyoda, H. Yamochi, G. Saito and K. Nakasuji, Mol. Cryst. Liq. Cryst. Sci. Technol., Sect. A, 2002, 379, 77–82 CrossRef CAS.
- Y. Morita, E. Miyazaki, J. Kawai, K. Sato, D. Shiomi, T. Takui and K. Nakasuji, Polyhedron, 2003, 22, 2219–2225 CrossRef CAS.
- Y. Morita, E. Miyazaki, J. Toyoda and K. Nakasuji, Bull. Chem. Soc. Jpn., 2003, 76, 205–206 CrossRef CAS.
- E. Miyazaki, Y. Morita and K. Nakasuji, Polyhedron, 2005, 24, 2632–2638 CrossRef CAS.
- Y. Morita, Y. Yakiyama, T. Murata and K. Nakasuji, Solid State Sci., 2008, 10, 1720–1723 CrossRef CAS.
- T. Murata, E. Miyazaki, K. Nakasuji and Y. Morita, Cryst. Growth Des., 2012, 12, 5815–5822 CrossRef CAS.
- B. D. Tilak, Proc. – Indian Acad. Sci., Sect. A, 1951, 33, 71–77 CrossRef.
- H. S. Desai and B. D. Tilak, J. Sci. Ind. Res., Sect. B, 1961, 20, 22–30 Search PubMed.
- P. R. Dingankar, T. S. Gore and V. N. Gogte, Indian J. Chem., 1971, 9, 24–30 CAS.
- J. B. Christensen, I. Johannsen and K. Bechgaard, J. Org. Chem., 1991, 56, 7055–7058 CrossRef CAS.
- J. B. Christensen and K. Bechgaard, J. Heterocycl. Chem., 2003, 40, 757–761 CrossRef CAS.
- J. P. Buisson and P. Demerseman, J. Heterocycl. Chem., 1990, 27, 2213–2214 CrossRef CAS.
- M. B. Mortensen, A. Schluter, J. Soerensen and J. B. Christensen, Acta Chem. Scand., 1997, 51, 807–809 CrossRef CAS.
- D. S. Tyson, E. F. Fabrizio, M. J. Panzner, J. D. Kinder, J.-P. Buisson, J. B. Christensen and M. A. Meador, J. Photochem. Photobiol., A, 2005, 172, 97–107 CrossRef CAS.
- J. B. Christensen, J. N. Soerensen, K. Schaumburg and K. Bechgaard, Molbank, 2014, M812/811–M812/814, DOI:10.3390/m812.
- N. Thorup, M. Hjorth, J. B. Christensen and K. Bechgaard, Acta Crystallogr., Sect. C: Cryst. Struct. Commun., 1992, 48, 530–533 CrossRef.
- N. Thorup, M. Hjorth, J. B. Christensen and K. Bechgaard, Synth. Met., 1993, 56, 2069–2072 CrossRef CAS.
- M. Hjorth, N. Thorup, J. B. Christensen and K. Bechgaard, Z. Kristallogr., 1993, 207, 91–101 CAS.
- J. Morgado, J. B. Christensen, R. T. Henriques, L. Alcacer and M. Almeida, Synth. Met., 1997, 86, 1967–1970 CrossRef CAS.
- T. C. Umland, S. Allie, T. Kuhlmann and P. Coppens, J. Phys. Chem., 1988, 92, 6456–6460 CrossRef CAS.
- C. J. Lambert and S. X. Liu, Chem. –Eur. J., 2018, 24, 4193–4201 CrossRef CAS PubMed.
- D. Casarini, L. Lunazzi and P. Sgarabotto, J. Crystallogr. Spectrosc. Res., 1991, 21, 445–450 CrossRef CAS.
- Bruker, Bruker AXS, Inc. SAINT, Version 7.68A, Bruker AXS, Madison, WI, 2009 Search PubMed.
- S. G. Sheldrick, Version 2008/2, University of Göttingen, Germany, 2003 Search PubMed.
- G. Sheldrick, Acta Crystallogr., Sect. A, 2008, 64, 112–122 CrossRef CAS PubMed.
- E. F. Pettersen, T. D. Goddard, C. C. Huang, G. S. Couch, D. M. Greenblatt, E. C. Meng and T. E. Ferrin, J. Comput. Chem., 2004, 25, 1605–1612 CrossRef CAS PubMed.
Footnotes |
† Electronic supplementary information (ESI) available: NMR-spectra of the compounds. CCDC 2051961, 2051922 and 2051918. For ESI and crystallographic data in CIF or other electronic format see DOI: 10.1039/d1ra00587a |
‡ Deceased. |
|
This journal is © The Royal Society of Chemistry 2021 |
Click here to see how this site uses Cookies. View our privacy policy here.