DOI:
10.1039/D1RA00802A
(Paper)
RSC Adv., 2021,
11, 13521-13529
Mesoporous adsorbent for competitive adsorption of fluoride ions in zinc sulfate solution
Received
30th January 2021
, Accepted 31st March 2021
First published on 12th April 2021
Abstract
Al–La hybrid gel was constructed using an innovative acid-catalyzed and calcination free sol–gel formation process which only included a sol–gel process lasting for 30 min and a drying procedure at 150 °C. This novel material was used as an adsorbent for competitive adsorption of fluoride ions in zinc sulfate solution. The properties, optimal adsorption conditions, synthetic principle and adsorption mechanism of the material were systemically investigated. The results showed that γ-AlO(OH) composed the skeleton of the Al–La hybrid gel and La(CH3COO)3 was embedded in the framework, which formed large amounts of ink-bottle type mesopores. A high fluoride ion adsorption rate with the removal rate reaching 50.88% within 1 min at 50 °C, 3 g L−1 was obtained. Analysis of the adsorption data has demonstrated that the adsorption of fluoride ions by the Al–La hybrid gel followed pseudo-second-order kinetics. Moreover, both Langmuir and Freundlich models can describe the adsorption process well. The maximum adsorption capacity of the Al–La hybrid adsorbent was 28.383 mg g−1. Furthermore, the mechanism analysis results indicated that the fluoride ions were mainly removed by the electrostatic adsorption on the AlO(OH), and a small amount of fluoride ions was also adsorbed by the complexation of lanthanum and fluoride ions. Since both AlO(OH) and La(CH3COO)3 had a large number of fluoride ion adsorption sites, the Al–La hybrid gel obtained an ideal adsorption capacity. In addition, the adsorption rate was greatly enhanced by the capillary action existing from the initial to the final stage of adsorption.
1. Introduction
Fluoride ions often cause adverse effects on industrial production and the environment.1–4 Currently, over 80% of zinc is produced through a hydrometallurgy process which is regarded as more eco-friendly.5,6 In the aforementioned practice, high concentrations of fluoride ions can cause multiple problems. Fluoride ions corrode the oxide film on the surface of the aluminum plate during the electrolytic deposition stage, which makes the cathode aluminum plate and the precipitated zinc bond together, increasing the difficulty of zinc stripping and consumption of the aluminum plate. Besides that, the corrosion of fluoride ions on the anode plate shortens the service time of the anode, and the presence of large amounts of fluoride ions with high toxicity worsens the working environment.7,8 Therefore, the realization of efficient defluoridation from zinc sulfate solution is significant. In numerous defluoridation technologies, adsorption has received extensive attention for high efficiency and low cost.9–11 Aluminum-based adsorbents are the most widely used fluoride ion sorbents with exceptional adsorption effects and low prices.12–14 However, the adsorption capacity and speed of traditional aluminum adsorbents are relatively low, so they need to be modified to get better adsorption properties. Rare earth compounds have a large adsorption capacity, low toxicity, and high selectivity to fluoride ions, but are expensive because of scarcity.15–18 Therefore, it is considered meaningful to modify aluminum sorbents with rare earth elements, which could combine the advantages of both materials.
There has been some progress in the research of rare earth modified aluminum-based materials used as fluoride ion adsorbents. Cheng et al. synthesized alumina (La-AA) adsorbent through dipping method which included a procedure of mixing reagents for two days.12 Wei et al. prepared Ca–Al–La composite sorbent by a one-step hydrothermal process which included an 8 h high temperature and pressure heating technique.19 However, the previous studies were aimed at the removal of fluoride ions in aqueous solutions rather than zinc sulfate electrolytes with much more complicated solution composition and ions form. In addition, the simpler preparation processing of this kind of adsorbent needs to be explored to reduce the consumption of time and energy.
In this study, Al–La hybrid gel prepared by innovative sol–gel method were used as fluoride ion sorbent. This method only included a rapid sol–gel processing lasting for 30 min and a drying procedure at 150 °C, which eliminated the high-temperature roasting process of typical sol–gel technique and quite differed from other preparation technologies with long flow or large time consuming. The material was used to adsorb fluoride ions in zinc sulfate solution and has been evaluated under different conditions including the amount of adsorbent, adsorption temperature and contact time. The properties of gel were characterized via using a variety of techniques, and the adsorption kinetics and isotherms were investigated. Additionally, the synthetic principle and adsorption mechanism of the adsorbent were explored.
2. Experimental
2.1. Chemicals
Pseudo-boehmite was the type of high purity and low sodium, which was purchased from Jinghuo Company (Shandong, China). Other reagents (La(NO3)3·6H2O, C2H4O2, ZnSO4·7H2O, CuSO4·5H2O, NaF, NaCl) were analytical grade. The simulated zinc sulfate solution was prepared referring to the actual solution composition of a smelter. 56.169 g ZnSO4·7H2O, 0.0785 g CuSO4·5H2O, 0.0329 g NaCl and 0.0221 g NaF were weighed and dissolved in 80 mL deionized water, preparing 100 mL simulated zinc sulfate solution. The main elemental contents of the solution are listed in Table 1.
Table 1 Main ingredients of the simulated zinc sulfate solution
Composition |
Zn |
Cu |
Cl |
F |
Concentration (g L−1) |
130 |
0.2 |
0.36 |
0.07 |
2.2. Preparation
The Al–La hybrid adsorbent was prepared by acid-catalyzed and calcination free sol–gel method: 0.374 g La(NO3)3·6H2O was weighed and dissolved in deionized water. After the solute dissolved, 1.621 g pseudo-boehmite was added into the solution under stirring. Until the mixing procedure continued for 30 min, the acetic acid solution was added to turn the turbid liquid into a sol. Finally, powdery dry gel was obtained through low-temperature drying (150 °C) and grinding steps. Schematic diagram of Al–La hybrid adsorbent preparation is displayed as Fig. 1.
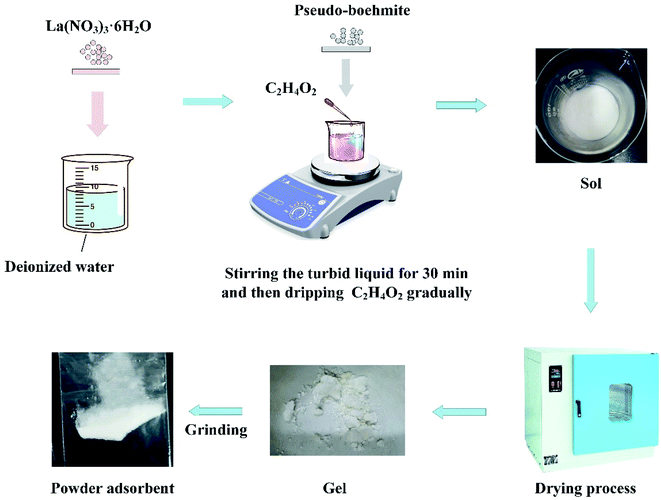 |
| Fig. 1 Schematic diagram of innovative sol–gel method. | |
2.3. Characterization
The phase of the adsorbent was confirmed by X-ray diffraction (XRD, X′ Pert PRO) with Cu Kα radiation, the test conditions were: scanning angle range 5–90°, scanning speed 5 (°) min−1, accelerating voltage 45 kV, applied current 40 mA. The morphology of the material was tested by Scanning Electron Microscope (SEM, SU8220) and Transmission Electron Microscope (TEM, FEI Talos-S). The specific surface area and pore size distribution of the adsorbent were measured by BET analysis (ASAP2460).
2.4. Batch experiments
15 mL simulated zinc sulfate solution was added to 25 mL centrifuge tube. The calculated amount of powdered gel was added into the centrifuge tube. And the mixture was shaken in a reciprocating constant temperature water bath oscillator at 120 rpm within a certain contact time. After adsorption, adsorbent and zinc sulfate solution were separated by filtration. The concentration of fluoride ions in solution before and after adsorption were measured by the fluoride ion selective electrode (BPX-932, Bell, China), and the defluoridation performance of the adsorbent was assessed by the calculation of eqn (1).where qe is the adsorption capacity of fluoride ions per unit weight of adsorbent (mg g−1) at equilibrium, C0 is the initial fluoride concentration (mg L−1), Ce is the equilibrium fluoride concentration (mg L−1), V is the volume (mL) of the zinc sulfate solution and m is the amount of adsorbent (g).
To further explore the optimal conditions for adsorption, the experiments based on variable factors were operated. To study the effect of adsorption temperature, the adsorption temperatures were controlled from 30 to 70 °C, respectively. The adsorbent dose was 10 g L−1 and the contact time was limited to 65 min. In the investigation of the effect of amount of adsorbent, the doses were adjusted to 1 g L−1, 3 g L−1, 5 g L−1, 10 g L−1 or 15 g L−1. The adsorption temperature was 50 °C and the contact time was controlled under 65 min.
To study the kinetic on the adsorption process, the fluoride ion content was measured in different time points from 1 to 64 min. The adsorption temperature was 50 °C and the adsorbent dose was 3 g L−1.
To obtain the adsorption isotherm on the process, simulated solutions with initial fluoride ion concentrations from 10 to 250 mg L−1 were prepared. The adsorption temperature was 50 °C; the adsorbent dose was 3 g L−1 and the contact time was 64 min.
3. Results and discussion
3.1. Characterization results
3.1.1. XRD pattern. The XRD pattern of the Al–La hybrid sample is exhibited in Fig. 2. It could be seen that most of the diffraction peaks were the characteristic peaks of γ-AlO(OH), especially those at 2θ = 13.9990°, 28.2015°, 38.3883°, 49.3223°. Thus, the principal phase of the sample was γ-AlO(OH).
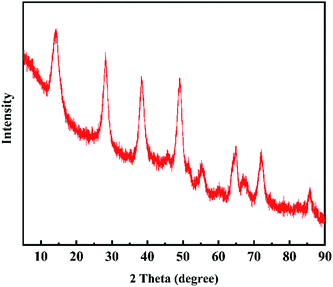 |
| Fig. 2 XRD pattern of the Al–La hybrid adsorbent. | |
3.1.2. Electron microscope images. The SEM images of the as-prepared sample are showed in Fig. 3A. It could be observed that the sample had a relatively rough surface. The TEM images of the adsorbent (Fig. 3B) show that the host phase with low crystallinity was of a similar structure to the traditional γ-AlO(OH), confirming that the host phase of the gel was γ-AlO(OH). The lanthanum complex loaded on hydroxy alumina had fine crystallinity, and the areas where the compound was concentrated contained a large number of mesopores.
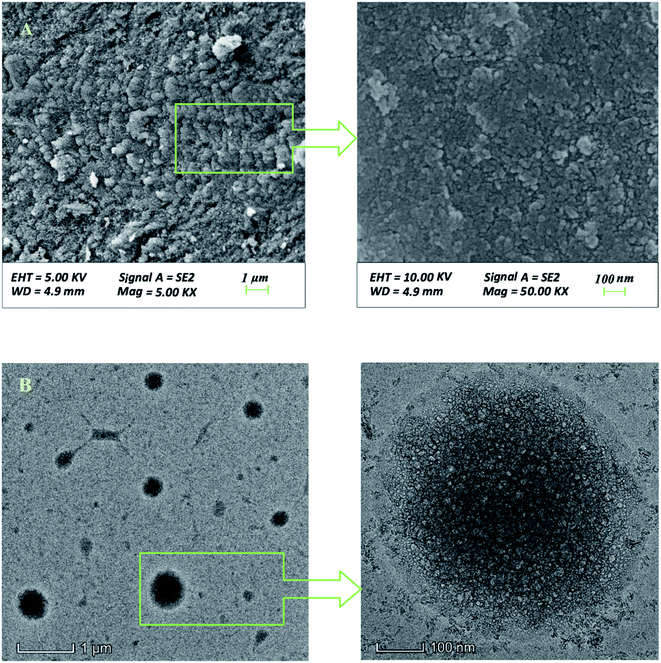 |
| Fig. 3 SEM images (A) and TEM images (B) of the Al–La hybrid adsorbent. | |
3.1.3. BET analysis. The N2 adsorption–desorption isotherms of the Al–La hybrid gel is displayed in Fig. 4. According to the classification of IUPAC,20 the isotherm linear plot of hybrid adsorbent could be classified as type IV isotherms type-H2 hysteresis loop, which indicated the mesoporous nature of synthesized materials. At lower pressures, the adsorption capacity increased slowly. H2 hysteresis loop were presented with the increase of relative pressures, revealing the presence of pores with narrow neck and wide body (often in the form of ink bottle pores).21 The pore distributed plot (Fig. 4B) reflected the average pore size of 3.358 nm, confirming the existence of mesopores. In addition, the specific surface area of the material was measured as 183.537 m2 g−1. The decrease in surface area can be attributed to the filling of rare earth compound on hydroxy alumina.22,23
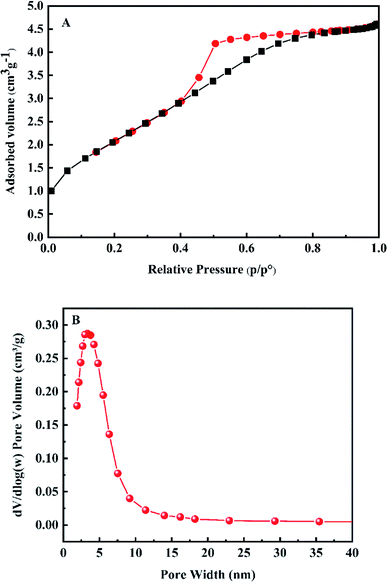 |
| Fig. 4 N2 adsorption–desorption isotherms (A) and BJH pore size distribution curve (B) of the Al–La hybrid adsorbent. | |
3.2. Optimization of application conditions
The effect of adsorption temperature on adsorbed capacity of the material is shown in Fig. 5. It could be observed that the adsorption capacity increased firstly and then decreased with the adsorption temperature from 30 °C to 70 °C, and the best adsorption efficiency could be obtained at 50 °C. Therefore, the temperature of the adsorption process was designated as 50 °C.
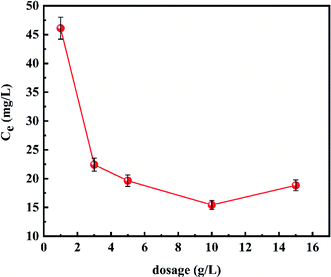 |
| Fig. 5 Effect of adsorption temperature (where qe (mg g−1) is the equilibrium adsorption capacity of the material, amount of adsorbent: 10 g L−1, initial fluoride ion concentration: 70.0 mg L−1, contact time: 65 min, pH: 4.5). | |
The effect of the amount of adsorbent on defluoridation ability of the material is depicted in Fig. 6. It was found that when the amount of adsorbent from 1 g L−1 to 3 g L−1, the concentrations of residual fluoride ions decreased from 46.110 mg L−1 to 22.425 mg L−1 rapidly. As the amount of adsorbent increased from 3 g L−1 to 10 g L−1, the decrease in the fluoride ion equilibrium concentration was greatly reduced. When the amount of adsorbent was further increased to 15 g L−1, the residual fluoride ion concentration showed an upward trend unexpectedly, which may be due to the flocculation phenomenon in the case of excessive powdered adsorbent. Thus, 3 g L−1 was selected as the optimal amount of adsorbent.
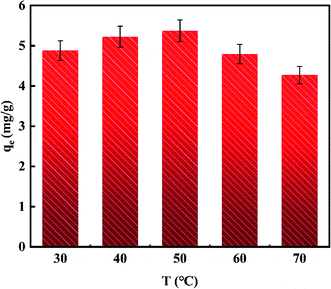 |
| Fig. 6 Effect of amount of adsorbent (where Ce (mg L−1) is the equilibrium concentration of fluoride ions, temperature: 50 °C, initial fluoride ion concentration: 70.0 mg L−1, contact time: 65 min, pH: 4.5). | |
The effect of the contact time of the adsorption process is presented in Fig. 7. It was obvious that the concentration of remaining fluoride ions in the solution dropped sharply from 32.934 mg L−1 to 23.857 mg L−1 in the first 16 min, then the downward trend became slow. Eventually, fluoride ion equilibrium concentration in the simulated solution remained unchanged at 20.685 mg L−1 after 64 min. Therefore, it can be considered that when the adsorption temperature and amount of adsorbent were 50 °C and 3 g L−1 respectively, the adsorption equilibrium time after modification was shortened to 64 min. Besides, the high fluoride ions adsorption rate with the removal rate reaching 50.88% within 1 min at 50 °C, 3 g L−1 was obtained.
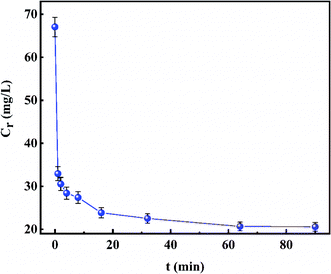 |
| Fig. 7 Effect of contact time (where Cr (mg L−1) is the residual concentration of fluoride ions, temperature: 50 °C, initial fluoride ion concentration: 70.0 mg L−1, amount of adsorbent: 3 g L−1, pH: 4.5). | |
3.3. Adsorption kinetics
To further evaluate the adsorption kinetics, the above data was further analyzed by kinetic equations including the pseudo-first-order and pseudo-second-order models.24–27
The pseudo-first-order rate equation can be given as eqn (2).
|
ln(qe − qt) = ln qe − k1t
| (2) |
The pseudo-second-order rate equation is expressed as eqn (3).
|
t/qt = 1/k2qe2 + t/qe
| (3) |
where
qe (mg g
−1) and
qt (mg g
−1) are the adsorbed capacities of fluoride ions on the Al–La hybrid adsorbent at equilibrium and at time
t respectively.
k1 and
k2 are the rate constants of the pseudo-first-order and pseudo-second-order models. The value of
qe,
k1 and
k2 could be obtained from the fitting plots (
Fig. 8) and are shown in
Table 2.
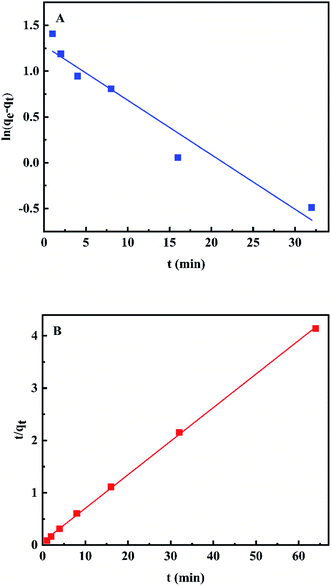 |
| Fig. 8 Pseudo-first-order (A) and pseudo-second-order (B) kinetic model fitting plots for fluoride ion adsorption on the Al–La hybrid adsorbent. | |
Table 2 Kinetic parameters for fluoride ion adsorption on the Al–La hybrid adsorbent
qe.exp (mg g−1) |
Pseudo-first-order model |
Pseudo-second-order model |
K1 (min−1) |
qe.cal (mg g−1) |
R2 |
K2 [g (min−1 g−1)] |
qe.cal (mg g−1) |
R2 |
15.460 |
0.05949 |
15.354 |
0.94766 |
0.06419 |
15.373 |
0.99954 |
As shown in Table 2, the goodness of fit of the pseudo-second-order model was better than that of the pseudo-first-order model with the correlation coefficient (R2) 0.99954 and 0.94766, respectively. In addition, the qe.cal value from the pseudo-second-order (15.373 mg g−1) was closed to the experimental value (15.460 mg g−1). Therefore, the pseudo-second-order kinetic model can explain the adsorption process well, indicating that the rate-limiting step was the surface adsorption involving chemisorption.28 The high rate constant k2 of Al–La hybrid adsorbent indicated the fast adsorption kinetics of fluoride ions on Al–La hybrid adsorbent. Meanwhile, the initial adsorption rate of gel was 11.372 mg g−1 min−1.
3.4. Adsorption isotherms
To further explore the adsorption mechanism, the adsorption isotherm was investigated. In recent research, Langmuir and Freundlich adsorption isotherm models are usually used to describe the adsorption isotherms.29–34
The Langmuir isotherm model describes the monolayer adsorption process in an ideal uniform sorption state. The primitive equation of the Langmuir model is given as eqn (4).
|
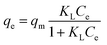 | (4) |
where
qe (mg g
−1) is the equilibrium adsorption capacity of the material,
Ce (mg L
−1) is the equilibrium concentration of fluoride ions,
qm (mg g
−1) is the maximum adsorption capacity and
KL (L mg
−1) is the Langmuir constant.
The Freundlich isotherm model describes the multi-layer adsorption process in a heterogeneous adsorption state. The primitive equation of the Freundlich model is expressed as eqn (5).
where
kF is the Freundlich constant and
n is the empirical constant.
Fig. 9 shows the modeling result and Table 3 lists corresponding parameters for the plots. By comparing the correlation coefficients (R2) of the Langmuir model (R2 = 0.965) and Freundlich model (R2 = 0.986), the experimental values are suitable for both models especially the latter, demonstrating a favorable non-uniform adsorption condition on the heterogeneous surface. For the Langmuir isotherm model, the KL (KL = 0.024) was indicative of the binding propensity of fluoride ions unto Al–La hybrid adsorbent under the experiment conditions. Based on the Freundlich model, the value of 1/n was 0.496 within 0.1–1, also revealing the favorable of Al–La hybrid adsorbent in defluoridation. The maximum adsorption capacity could be analyzed as 28.383 mg g−1. Moreover, the first inflection point in the curve was the result of competitive adsorption of fluoride ions between adsorbent and zinc ions in solution. Furthermore, the isotherm showed a trend almost perpendicular to the X axis in the dilute solution area, which should be attributed to capillary action appearing from the initial stage of adsorption.35,36
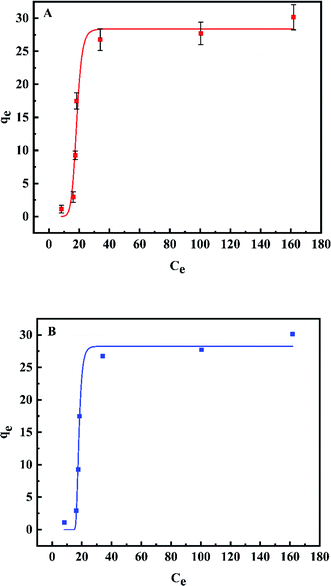 |
| Fig. 9 Fitting plots of the Langmuir adsorption isotherm (A) and Freundlich adsorption isotherm (B). (Temperature: 50 °C, amount of adsorbent: 3 g L−1, contact time: 64 min, pH: 4.5). | |
Table 3 Langmuir and Freundlich adsorption isotherm parameters
Langmuir |
Freundlich |
Parameter |
Value |
Parameter |
Value |
KL |
0.024 |
KF |
3.042 |
qm |
28.383 |
n |
2.016 |
R2 |
0.965 |
R2 |
0.986 |
3.5. Synthesis and adsorption mechanisms
The FTIR spectra of the material are depicted in Fig. 10. According to the peaks between 400–800 in FTIR curve (a) and the crystalline state of lanthanum complex in TEM images, it can be inferred that lanthanum should exist in the form of lanthanum acetate. Hydrosol was obtained via mixing pseudo boehmite, lanthanum nitrate and acetic acid in deionized water under stirring. A large number of hydroxyl groups on the surface of the pseudo-boehmite crystallites adsorbed hydrogen ions as bridge to connect the hydroxyl groups of other pseudo-boehmite particles, which formed a network framework constituted by particles losing fluidity. Nitrate ions on the surface of the colloidal particles further constructed a stable electric double layer structure. Besides that, the generated lanthanum acetate entered the gaps of the network structure under the action of hydrogen bonding.
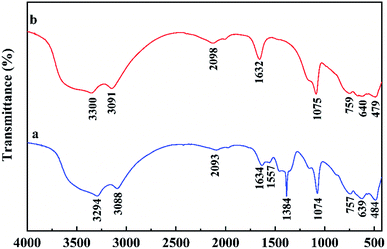 |
| Fig. 10 FTIR spectra of the Al–La hybrid adsorbent before (a) and after (b) adsorption. | |
The simultaneous TG-DSC curves used to explore the further reaction of hydrogel in drying process are shown in the Fig. 11. The curves showed mass loss of the hydrogel from room temperature to 300 °C and thermal events corresponding to the loss. The fast mass loss accompanied by endothermic peak between 25 °C and 75 °C should be attributed to the removal of adsorbed water in hydrogel, whilst the slow mass loss step corresponding to the exothermic event between 75 and 170 °C was related to the continuous decomposition of nitric acid. Therefore, the changes in the drying process can be inferred as follows: The solid–liquid interface was gradually replaced by the solid–gas due to the evaporation of the adsorbed water, which produced the capillary action. A large amount of lanthanum acetate entered the aluminum intensive areas attributed to higher solid–liquid interfacial tension and stronger capillary action here. These small rings where aluminum gathered would not collapse attributed to the support of lanthanum acetate, and the interconnected mesopores conductive to defluoridation were formed.
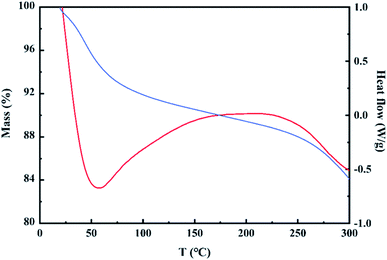 |
| Fig. 11 TG-DSC curves of Al–La hydrogel. | |
TEM, BET and synthesis mechanism analyses revealed the mesoporous structure of synthesized materials. Based on the mesoporous structure and isotherm curve almost perpendicular to the X axis in the dilute solution area, it can be deduced that the adsorption process was accompanied by capillary action appearing from the initial adsorption stage. Therefore, in the initial adsorption stage, fluoride ions can not only be adsorbed by the protonated hydroxyl groups on the surface, but also be readily accessible to the protonated hydroxyl groups and rare earth ions in the pores through capillary action, producing the high fluoride ions adsorption rate with the removal rate reaching 50.88% within 1 min at 50 °C, 3 g L−1 (Fig. 7).
FTIR spectra (Fig. 10) of the Al–La hybrid adsorbent before and after adsorption were compared to further explore the adsorption mechanism.37–39 The peak at 3294 cm−1 could be attributed to the tensile vibration of hydroxyl groups and the bands at 1634 cm−1 and 1557 cm−1 were assigned to the bending vibration of hydroxyl groups. These peaks were all shifted to higher wavelength positions in the composite after adsorption, which suggested that the hydroxyl groups adsorbed fluoride ions through electrostatic adsorption.11 The adsorption bands between 400–800 cm−1 were derived from the presence of La(CH3COO)3. In the material after adsorption, the changes in peak shape indicated that lanthanum was complexed with fluoride ions.18,19 Besides, the disappearance of the peak at 1384 cm−1 was due to the dissolution of nitrate ions in the sorbent during the adsorption process.
The element distribution of Al, La and F in the material after adsorption was investigated by EDS mapping method. As shown in Fig. 12, the element distribution of F and Al was similar, which indicated that the main mechanism of adsorption was the electrostatic adsorption of AlO(OH) to fluoride ions. It can be seen that La was mainly distributed in the gaps of AlO(OH), and a small amount of F was also present in the areas where La was dense. Therefore, it can be further confirmed that lanthanum had a complexing action on fluoride ions.
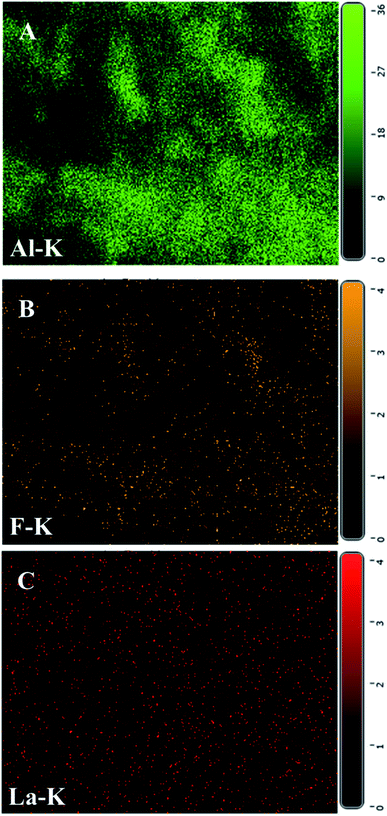 |
| Fig. 12 EDS mapping of Al (A), F (B) and La (C) of the Al–La hybrid adsorbent after adsorption. | |
Based on the above analyses, the adsorption process mechanism can be inferred as follows: The Al–La hybrid adsorbent was a mesoporous material. The main body of this composite was γ-AlO(OH) with large area distribution, and La(CH3COO)3 was embedded in the AlO(OH). Compared with the rare earth modified aluminum-based materials prepared by traditional methods, the rare earth was loaded in the interior rather than on the surface of the aluminum matrix, forming a more stable structure. Many ink-bottle type mesopores were formed attributed to this distribution state. When in contact with zinc sulfate solution, the liquid quickly entered the pores through capillary action. The fluoride ions in the solution were mainly adsorbed by the protonated hydroxyl groups through electrostatic adsorption, and a few fluoride ions were complexed by lanthanum.
4. Conclusions
Al–La hybrid gel was prepared by a simple sol–gel method innovatively and used for the adsorption of fluoride ions in zinc sulfate solution. The body of the sorbent was γ-AlO(OH) and La(CH3COO)3 was embedded in the host material, which formed large amounts of ink-bottle type mesopores. It could be found that at 50 °C, the adsorbent with amount of adsorbent of 3 g L−1 was able to get an excellent adsorption effect, and the adsorption equilibrium time after modification was shortened to 64 min under the influence of capillary effect. The pseudo-second-order kinetic model can fit the adsorption process well. The initial adsorption rate and the kinetic rate constant k2 of Al–La hybrid gel were 11.372 mg g−1 min−1 and 0.06419 g min−1 g−1, respectively (at temperature: 50 °C, initial fluoride ion concentration: 70.0 mg L−1), showing an excellent kinetic performance compared with other aluminum-based adsorbents. For example, amorphous porous layered-Al2O3 derived from AlFu MOFs was used as an adsorbent for removing fluoride ions in industrial ZnSO4 solution, the initial adsorption rate and the kinetic rate constant k2 of it was 0.621 mg g−1 min−1 and 0.00633 g min−1 g−1, respectively (at temperature: 40 °C, initial fluoride ion concentration: 120 mg L−1).9 The kinetic rate constant k2 of a layered aluminum-based composite, which was used for adsorbing fluoride ions in industrial ZnSO4 solution, was 0.03381 g min−1 g−1 (at temperature: 50 °C).8 Additionally, the adsorption equilibrium time of Al–La hybrid was shortened to 64 min (at temperature: 50 °C, initial fluoride ion concentration: 70.0 mg L−1), confirming the excellent kinetic performance compared with other aluminum-based adsorbents. As an example, the sorption equilibrium of an Al–Ce hybrid adsorbent was achieved within 5 h when the initial fluoride concentration was 5 g L−1.14 Both Langmuir model and Freundlich model matched the adsorption isotherm of the material well, and the adsorbent had a relative high sorption capacity up to 28.383 mg g−1 for fluoride, higher than that of some aluminum based adsorbent adsorbents (e.g. The maximum adsorption capacity of a La3+-modified activated alumina for fluoride removal was 6.70 mg g−1.12). It can be concluded that the adsorption should be a process based on the electrostatic adsorption of hydroxy and lanthanum fluoride complexation, and accompanied by capillary action existed from the initial stage of adsorption. In conclusion, since both AlO(OH) and La(CH3COO)3 had a large number of fluoride ion adsorption sites, the Al–La hybrid gel possessed an ideal adsorption capacity. In addition, the adsorption rate was greatly enhanced by the filling load mode of the composite. The stable structure and the synthetic method with low time and energy consumption also revealed a large difference of Al–La hybrid gel from other aluminum-based adsorbents. Although the selectivity for fluoride ion needs to be improved, Al–La hybrid adsorbent suggests potential applications in defluoridation from zinc sulfate electrolytes as well as other solutions with complicated composition and ions form.
Conflicts of interest
There are no conflicts to declare.
Acknowledgements
This work was supported by the Key Laboratory Project of Shaanxi Provincial Department of Education (No. Z202001511) and the Punai Functional Materials Co., Ltd (Contract No. 20200216).
Notes and references
- A. Banihashemi, K. Zare, V. Javanbakht and H. Mohammadifard, Mater. Chem. Phys., 2021, 258, 123934 CrossRef CAS.
- A. Jeyaseelan, M. Naushad, T. Ahamad and N. Viswanathan, J. Environ. Chem. Eng., 2020, 104563 Search PubMed.
- N. Antuñano, J. F. Cambra and P. L. Arias, Hydrometallurgy, 2016, 161, 65–70 CrossRef.
- S. Mandal and S. Mayadevi, Chemosphere, 2008, 72, 995–998 CrossRef CAS PubMed.
- Q. Zhang and Y. Hua, Mater. Chem. Phys., 2012, 134, 333–339 CrossRef CAS.
- S. Tokunaga, M. J. Haron, S. A. Wasay, K. F. Wong, K. Laosangthum and A. Uchiumi, Int. J. Environ. Stud., 1995, 48, 17–28 CrossRef.
- X. Wu, Z. Liu and X. Liu, Hydrometallurgy, 2014, 141, 31–35 CrossRef CAS.
- Z. Tian, W. Guo, Z. Zhang, Y. Lai, S. Ye and J. Li, Hydrometallurgy, 2017, 171, 222–227 CrossRef CAS.
- K. Yang, Y. Li, Z. Zhao, Z. Tian and Y. Lai, Chem. Eng. Res. Des., 2020, 153, 562–571 CrossRef CAS.
- P. Loganathan, S. Vigneswaran, J. Kandasamy and R. Naidu, J. Hazard. Mater., 2013, 248–249, 1–19 CrossRef CAS PubMed.
- S. Deng, H. Liu, W. Zhou, J. Huang and G. Yu, J. Hazard. Mater., 2011, 186, 1360–1366 CrossRef CAS PubMed.
- J. Cheng, X. Meng, C. Jing and J. Hao, J. Hazard. Mater., 2014, 278, 343–349 CrossRef CAS PubMed.
- S. Jagtap, M. K. Yenkie, N. Labhsetwar and S. Rayalu, Chem. Rev., 2012, 112, 2454–2466 CrossRef CAS PubMed.
- H. Liu, S. Deng, Z. Li, G. Yu and J. Huang, J. Hazard. Mater., 2010, 179, 424–430 CrossRef CAS PubMed.
- S. Tokunaga, M. J. Haron, S. A. Wasay, K. F. Wong, K. Laosangthum and A. Uchiumi, Int. J. Environ. Stud., 1995, 48, 17–28 CrossRef.
- A. Ghosh, S. Chakrabarti, K. Biswas and U. C. Ghosh, Appl. Surf. Sci., 2014, 307, 665–676 CrossRef CAS.
- A. M. Raichur and M. Jyoti Basu, Purif. Technol., 2001, 24, 121–127 CrossRef CAS.
- Y. He, L. Zhang, X. An, G. Wan, W. Zhu and Y. Luo, Sci. Total Environ., 2019, 688, 184–198 CrossRef CAS PubMed.
- X. Wei, G. Zhang, Y. Zhang, D. Tang and J. Wang, Chem. Eng. J., 2014, 250, 423–430 CrossRef.
- S. W. K. Sing, Pure Appl. Chem., 1985, 57, 603–619 Search PubMed.
- D. Dayananda, V. R. Sarva, S. V. Prasad, J. Arunachalam and N. N. Ghosh, Chem. Eng. J., 2014, 248, 430–439 CrossRef CAS.
- E. C. Nnadozie and P. A. Ajibade, Microporous Mesoporous Mater., 2020, 309, 110573 CrossRef CAS.
- M. Li, D. Wei, T. Liu, Y. Liu, L. Yan, Q. Wei, B. Du and W. Xu, Sep. Purif. Technol., 2019, 227, 115696 CrossRef CAS.
- Y. S. Ho and G. McKay, Process Biochem., 1999, 34, 451–465 CrossRef CAS.
- G. E. J. Poinern, M. K. Ghosh, Y.-J. Ng, T. B. Issa, S. Anand and P. Singh, J. Hazard. Mater., 2011, 185, 29–37 CrossRef CAS PubMed.
- N. Chen, Z. Zhang, C. Feng, M. Li, D. Zhu, R. Chen and N. Sugiura, J. Hazard. Mater., 2010, 183, 460–465 CrossRef CAS PubMed.
- X. Fan, D. J. Parker and M. D. Smith, Water Res., 2003, 37, 4929–4937 CrossRef CAS PubMed.
- Y. S. Ho, J. Hazard. Mater., 2006, 136, 681–689 CrossRef CAS PubMed.
- A. Mittal, L. Kurup and J. Mittal, J. Hazard. Mater., 2007, 146, 243–248 CrossRef CAS PubMed.
- G. Sugihara, D. S. Shigematsu, S. Nagadome, S. Lee, Y. Sasaki and H. Igimi, Langmuir, 2000, 16, 1825–1833 CrossRef CAS.
- K. Y. Foo and B. H. Hameed, Chem. Eng. J., 2010, 156, 2–10 CrossRef CAS.
- F. Gimbert, N. Morin-Crini, F. Renault, P.-M. Badot and G. Crini, J. Hazard. Mater., 2008, 157, 34–46 CrossRef CAS PubMed.
- Y. Liu, H. Xu and J.-H. Tay, J. Environ. Eng., 2005, 131, 1466–1468 CrossRef CAS.
- C. K. Aslani and O. Amik, Appl. Radiat. Isot., 2020, 109474 Search PubMed.
- J. Van Brakel and P. M. Heertjes, Nature, 1977, 268, 44–45 CrossRef.
- G. Reichenauer and G. W. Scherer, Colloids Surf., A, 2001, 187–188, 41–50 CrossRef CAS.
- L. Li, H. Ren, Y. Liu, X. Liu, Y. Zhao, X. Zhou, W. Kang, X. Zhuang and B. Cheng, Microporous Mesoporous Mater., 2020, 308, 110544 CrossRef CAS.
- E. Santoso, R. Ediati, Z. Istiqomah, D. O. Sulistiono, R. E. Nugraha, Y. Kusumawati, H. Bahruji and D. Prasetyoko, Microporous Mesoporous Mater., 2021, 310, 110620 CrossRef CAS.
- R. Yu and Z. Wu, Microporous Mesoporous Mater., 2020, 308, 110494 CrossRef CAS.
|
This journal is © The Royal Society of Chemistry 2021 |
Click here to see how this site uses Cookies. View our privacy policy here.