DOI:
10.1039/D1RA02774C
(Paper)
RSC Adv., 2021,
11, 24787-24793
Identification and in situ removal of an inhibitory intermediate to develop an efficient phytosterol bioconversion process using a cyclodextrin-resting cell system†
Received
9th April 2021
, Accepted 30th June 2021
First published on 15th July 2021
Abstract
A classically versatile steroid intermediate, 9α-hydroxyandrost-4-ene-3,17-dione (9α-OH-AD), can be obtained by phytosterol (PS) bioconversion using Mycobacterium. In this study, a cyclodextrin-resting cell reaction system with a high concentration of PS (50 g L−1) was used to produce 9α-OH-AD. However, the inhibitory effect of metabolic intermediates is a key factor limiting production efficiency. After the separation and identification of a series of metabolic intermediates, it was found that 4-ene-3-keto steroids, which are the first metabolites of sterol side-chain degradation, accumulated at the beginning of the bioprocess and had a remarkable inhibitory effect on bioconversion. The bioconversion rate was greatly improved when 5 g L−1 of macroporous adsorbent resin D101 was added to the reaction system in the initial phase. A certain amount of resin acted as a reservoir to remove the inhibitory intermediate in situ and facilitated the bioconversion process, and the 9α-OH-AD space–time yield increased to 8.51 g L−1 d−1, which was 23.15% higher than that without resin addition (6.91 g L−1 d−1) after 72 h bioconversion. In summary, we identified an inhibitory intermediate that limits the bioconversion rate and provided a solution based on resin adsorption for improving 9α-OH-AD production efficiency in a commercial-scale process.
1. Introduction
In the past decades, researchers have focused on the microbial side-chain degradation of phytosterols (PS) or cholesterol to produce steroid intermediates.1,2 9α-Hydroxyandrost-4-ene-3,17-dione (9α-OH-AD) is one of the intermediates that can further be chemically modified to steroid drugs, such as hydrocortisone and eplerenone.3 However, the bioavailability of PS and the cytotoxicity of intermediate metabolites are the key factors that limit production efficiency, particularly at high substrate concentrations.4
Currently, the synthesis efficiency of steroid intermediates is enhanced by a variety of metabolic regulation methods. Genetic engineering modification of the production strains is a widely used method to improve bioconversion. Yao et al. reported an engineered Mycobacterium-transformed PS to 9α-OH-AD.5 In addition, diverse sterol-solubilizing reaction systems, including a cyclodextrin complexing system,4,6 biocompatible ionic liquid system,7 microdispersion/emulsification system, and surfactant addition,8,9 were established to improve the poor water solubility of PS. Among them, the cyclodextrin-resting cell system is an ideal method that has been successfully used for the production of a variety of steroid intermediates. It was observed that not only was the expression of genes related to PS metabolism influenced but the degradation of steroid intermediates was also inhibited in this reaction system.10,11 Nonetheless, an extended bioconversion period and a lower reaction rate still exist, especially at high PS concentrations. The inhibitory effect of metabolic intermediates is a key factor limiting production efficiency.4 Thus, many resin-based in situ removal methods have been used to alleviate the inhibition of intermediates and improve the bioconversion efficiency of many steroids, such as androsta-1,4-diene-3,17-dione and testosterone.12–14
In situ product removal (ISPR) is a common method used for the simultaneous separation of inhibitory products during fermentation and biotransformation. Macroporous resins are promising candidates for ISPR materials and have many advantages, such as alleviated feedback suppression, low cost, and high phase stability.15,16 In recent years, macroporous resins have been successfully used to produce various metabolites, such as diepoxin ζ, biogenic amines, botrallin and TMC-264.17–19 However, the study on the influence of adsorption resin on the performance of Mycobacterium to produce 9α-OH-AD from PS at high substrate concentrations has not been published and requires further investigation.
In this study, 9α-OH-AD was obtained from PS through biotransformation using the cyclodextrin-resting cell system at a high substrate concentration. We evaluated the effect of the adsorption resin on the reaction efficiency of 9α-OH-AD production. The ISPR system with different resin types, times, and amounts was measured to optimize the bioconversion process. Furthermore, we investigated the mechanism by which the adsorption resin improved phytosterol bioconversion efficiency, and we separated and identified the inhibitory intermediate that limits the bioconversion rate. Using the in situ removal strategy, we were able to provide a solution for improving 9α-OH-AD production efficiency at high substrate concentrations.
2. Materials and methods
2.1 Microorganisms, medium and reagents
Mycobacterium neoaurum NwIB-HK86 (devoid of 3-ketosteroid-1,2-dehydrogenase activity; 3-ketosteroid-9-hydroxylase and cholesterol oxidases overexpressed) can bioconvert PS to produce 9α-OH-AD as the main product. MYC/01 seed medium was used as an amplification medium for microorganisms, as previously described by Gao et al.4 The seeds were then inoculated at 5% (v/v) into 500 mL shake flasks containing 100 mL of MYC/04 broth and cultured at 30 °C for 72 h on a 200 rpm shaker. MYC/04 were composed of (g L−1) K2HPO4·3H2O, 0.5; MgSO4·7H2O, 0.5; glucose, 30; sodium citrate, 2.8; ammonium ferric citrate, 0.05; starch, 2; corn steep powder, 3; and PS, 0.1. The initial pH was controlled at 8.0.
The reagents described in the above medium composition were purchased from Shanghai Titan Scientific Co., Ltd. (Shanghai, China) or Sinopharm Chemical Reagent Co., Ltd. (Shanghai, China). 9α-OH-AD, AD standards were obtained from Sigma (Shanghai, China). PS and HP-β-CD were purchased from Shanxi Sciphar Natural Products Co., Ltd. (Shangluo, China) and Shandong Binzhou Zhiyuan Biotechnology Co., Ltd. (Binzhou, China), respectively. The organic solvents used in the mobile phase, e.g., methanol, acetonitrile, isopropanol, were all HPLC grade.
2.2 Preparation of resting cells and macroporous resins
Resting cells were prepared according to the method described by Gao et al.4 Ten types of macroporous adsorbent resins were employed as carriers for the in situ adsorption technique during the production of 9α-OH-AD, and were purchased from Shanghai Huazhen Technology Co., Ltd. (Shanghai, China). The physical and chemical properties of the resins are listed in Table S1.† The resins were pre-treated by soaking in ethanol at 30 °C for 48 h, then washed three times with deionised water for 24 h each, followed by drying at 55 °C to a constant weight.
2.3 Bioconversion with ISPR by addition of macroporous resins
Precisely, weighed resin was added to the reaction solution at the beginning of the bioconversion (except for experiments exploring the optimum time to add resin). The transformation of PS was performed under non-sterile conditions with a 10 mL PB (20 mM, pH = 8.0) system consisting of 100 g L−1 wet cells, 50 g L−1 PS, and 200 g L−1 HP-β-CD, placed on a shaker at a rotation speed of 200 rpm and 30 °C for the reaction. All experiments were repeated three times.
2.4 Metabolites analytical methods
The resins were separated from the reaction system using a single layer gauze. The samples from the reaction solution and resins were extracted with ten volumes of ethyl acetate (before desorption, the resin was washed twice with deionized water to remove non-adsorbed products). After centrifugation, an appropriate amount of the upper organic phase was withdrawn and allowed to evaporate completely before detection by high-performance liquid chromatography (HPLC) with Hypersil ODS2-column. Methanol–water (80
:
20, v/v) was used as the mobile phase at a flow rate of 1 mL min−1 and UV detector at 254 nm was used to investigate the main steroidal metabolites, 9α-OH-AD et al. For the detection of phytosterols, the mobile phase and detection wavelength were changed to acetonitrile–isopropanol (70
:
30, v/v) and 210 nm, respectively.
3. Results and discussion
3.1 Transformation of PS by Mycobacterium neoaurum NwIB-HK86 under resting cell system
In our previous study, Mycobacterium neoaurum NwIB-HK86 efficiently produced the main product 9α-OH-AD from phytosterols in HP-β-CD-resting cell system, accompanied by more than 20% of by-products such as androstenedione (AD), 9,22-dihydroxy-23,24-bisnorchol-4-en-3-one (DHBC), and 9,24-dihydroxychol-4-en-3-one (DHC).20 Furthermore, the results demonstrated that the reaction was remarkably inhibited by high levels of substrates and metabolites. As shown in Fig. 1, 9α-OH-AD production increased slowly during the first 24 h. Subsequently, the accumulated products entered a rapid growth stage and reached the maximum level (25.1 g L−1) at 96 h. The PS was also depleted after 96 h of bioconversion, following the accumulation of 9α-OH-AD. After further incubation, 9α-OH-AD entered the degradation stage, and the yield began to decrease.
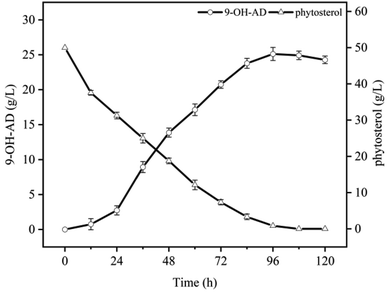 |
| Fig. 1 Time profiles of 9α-OH-AD production by M. neoaurum NwIB-HK86 transforming phytosterols under cyclodextrin-resting cells system. The reaction system consisted of 50 g L−1 of phytosterols, 100 g L−1 of wet biomass and 200 g L−1 of HP-β-CD, were carried out in 250 mL shake flasks containing 10 mL of reaction solution for 5 d (error bars for standard deviations, n = 3). | |
3.2 Application of ISPR in the process
3.2.1 Selection of different adsorption resins in bioconversion. To further improve the efficiency of 9α-OH-AD bioconversion, the ISPR method was used in the system to alleviate the inhibition of metabolites. The influence of ten types of adsorption resins on 9α-OH-AD production was evaluated in this study. The production of 9α-OH-AD and the consumption of PS were remarkably influenced by the different adsorption resins (Table 1). Among them, the D101 resin significantly increased the space–time yield of 9α-OH-AD (from 6.91 to 7.76 g L−1 d−1), leading to a 12.25% increase in the total yield of 9α-OH-AD (23.28 g L−1) compared to the control group after 72 h of bioconversion by Mycobacterium, which was most suitable for further exploration. In addition, the strains showed strong bioconversion activity of PS after the addition of D101 resin. The PS in the D101 group was consumed by 92.78% after 72 h, with a 7.48% increase compared to the control group, which could contribute to production cost savings by reducing the reaction period. However, all the other nine resins exerted negative effects on PS conversion and 9α-OH-AD production. Consequently, D101 is the most effective resin for in situ adsorption. Furthermore, the distribution of 9α-OH-AD in the reaction solution and resins was investigated. Initially, we thought that the feasibility of using D101 as an absorbent may be due to, in part, its ability to adsorb the main product, 9α-OH-AD. However, only 2.23 g L−1 of 9α-OH-AD converged at the resins, accounted for the 9.58% of the total 9α-OH-AD yield, suggesting that the adsorption of 9α-OH-AD may not be a key factor in improving the reaction rate.
Table 1 Effects of different resins on 9α-OH-AD production, space–time yield and phytosterols consumptiona
Resin |
9α-OH-AD in supernatant (g L−1) |
9α-OH-AD in resins (g L−1) |
Total 9α-OH-AD (g L−1) |
Conversion rate of PS (%) |
Space–time yield (g L−1 d−1) |
Except for the control group with no resin addition (CK), all the resins were added at a concentration of 20 g L−1 at 0 h. — means not detected. All experiments contained 50 g L−1 of phytosterols, 100 g L−1 of wet biomass, 200 g L−1 of HP-β-CD, and 10 mL of reaction solution bioconverted in 250 mL shake flasks for 3 d (error bars for standard deviations, n = 3). |
CK |
20.74 ± 0.54 |
— |
20.74 ± 0.74 |
85.30 ± 0.39 |
6.91 ± 0.24 |
D101 |
21.05 ± 0.38 |
2.23 ± 0.04 |
23.28 ± 0.86 |
92.78 ± 0.30 |
7.76 ± 0.19 |
SP 207 |
17.90 ± 1.12 |
1.16 ± 0.01 |
19.06 ± 0.49 |
82.72 ± 0.73 |
6.35 ± 0.18 |
HZ 820 |
12.24 ± 0.24 |
0.72 ± 0.09 |
12.96 ± 0.38 |
66.67 ± 0.65 |
4.32 ± 0.27 |
HZ 818 |
15.94 ± 0.78 |
1.33 ± 0.03 |
17.27 ± 0.51 |
78.22 ± 0.71 |
5.76 ± 0.31 |
HZ 806 |
6.36 ± 0.01 |
0.54 ± 0.02 |
6.90 ± 0.25 |
57.89 ± 0.57 |
2.30 ± 0.22 |
HZ 801 |
16.24 ± 0.13 |
0.76 ± 0.05 |
17.00 ± 0.53 |
75.41 ± 0.81 |
5.67 ± 0.27 |
HPD 826 |
3.63 ± 0.15 |
0.14 ± 0.01 |
3.77 ± 0.19 |
42.80 ± 0.55 |
1.22 ± 0.11 |
HPD 500 |
11.83 ± 0.70 |
0.69 ± 0.04 |
12.52 ± 0.45 |
70.89 ± 1.25 |
4.17 ± 0.24 |
HPD 300 |
7.04 ± 0.51 |
0.48 ± 0.04 |
7.52 ± 0.47 |
55.09 ± 0.68 |
2.51 ± 0.13 |
HPD 100 |
5.71 ± 0.59 |
0.29 ± 0.01 |
6.00 ± 0.32 |
52.26 ± 0.53 |
2.00 ± 0.21 |
3.2.2 Optimization of the concentration of D101 adsorbent resin in bioconversion. A set of experiments was conducted with different concentrations of resin D101, ranging from 0 to 50 g L−1, to determine the optimum resin concentration for the 9α-OH-AD production process, as shown in Table 2. As the adsorbed resin concentration increased from 0 to 5 g L−1, D101 exhibited an increased effect on the production of 9α-OH-AD and the bioconversion of PS. The space–time yield of 9α-OH-AD increased from 6.91 to 8.51 g L−1 d−1 in a concentration-dependent manner. And the PS was completely exhausted at 72 h, which was 14.7% higher than that of the control. Unexpectedly, PS bioconversion and 9α-OH-AD production showed reverse trends as the D101 concentration continuously increased from 5 g L−1 to 50 g L−1, even though the proportion of 9α-OH-AD adsorbed in the resin continued to increase and reached a maximum of 20.23%, which further demonstrates that 9α-OH-AD adsorption is not an essential factor in increasing the reaction rate. After resin desorption, it was found that the higher the dose of resin D101 added during the bioconversion process, the more metabolic intermediates adsorbed in the resin, which may be the reason for lower production rate of 9α-OH-AD. Furthermore, the high concentration of resin in the system could restrict mass transfer and thus affect the space–time yield of 9α-OH-AD. These results indicate that the addition of 5 g L−1 of D101 is sufficient to facilitate the production of 9α-OH-AD and that an overdose of resin inhibits the conversion of PS.
Table 2 Effects of different concentrations of resin D101 on 9α-OH-AD production, space–time yield and phytosterol consumptiona
D101 concentrations (g L−1) |
9α-OH-AD in supernatant (g L−1) |
9α-OH-AD in resins (g L−1) |
Total 9α-OH-AD (g L−1) |
Conversion rate of PS (%) |
Space–time yield (g L−1 d−1) |
D101 resin was added at the beginning of the reaction. All experiments contained 50 g L−1 phytosterols, 100 g L−1 wet biomass, 200 g L−1 HP-β-CD, 10 mL reaction solution bioconverted in 250 mL shake flasks for 3 d (error bars for standard deviations, n = 3). |
0 |
20.74 ± 0.54 |
— |
20.74 ± 0.74 |
85.30 ± 0.39 |
6.91 ± 0.24 |
3 |
22.02 ± 0.34 |
0.23 ± 0.02 |
22.25 ± 0.51 |
89.16 ± 0.34 |
7.42 ± 0.10 |
5 |
24.75 ± 0.43 |
0.78 ± 0.18 |
25.53 ± 0.23 |
100 ± 0.00 |
8.51 ± 0.15 |
10 |
22.45 ± 0.18 |
1.26 ± 0.09 |
23.71 ± 0.50 |
94.68 ± 0.12 |
7.90 ± 0.44 |
20 |
21.05 ± 0.38 |
2.23 ± 0.04 |
23.28 ± 0.86 |
92.78 ± 0.30 |
7.76 ± 0.19 |
30 |
17.96 ± 0.58 |
2.74 ± 0.31 |
20.70 ± 0.38 |
82.98 ± 0.83 |
6.90 ± 0.15 |
40 |
15.88 ± 0.17 |
3.32 ± 0.29 |
19.20 ± 0.73 |
79.44 ± 0.15 |
6.40 ± 0.25 |
50 |
14.63 ± 0.58 |
3.71 ± 0.47 |
18.34 ± 0.49 |
76.28 ± 0.96 |
6.11 ± 0.17 |
3.2.3 Optimization of resin D101 addition time in the bioconversion process. The addition time of the adsorbent resin is another key factor in maximizing resin utilization efficiency during PS bioconversion by Mycobacterium in the resting cell systems. The influence of different D101 addition times, ranging from 0 to 60 h, on 9α-OH-AD production process was investigated. As shown in Table 3, the results showed that resin D101 was optimally added at 0 h, allowing PS to be entirely depleted, and the 9α-OH-AD space–time yield increased by 23.15% to 8.51 g L−1 d−1 compared to the control group at 72 h. Apart from this, the PS bioconversion efficiency decreased gradually when the addition of D101 was later than 0 h because a short incubation time for the resin D101 might not necessarily alleviate the inhibitory effect of the toxic products. These results are contrary to the conclusions of a previously published study on the timing of resin addition for the production of ADD, diepoxin ζ, botrallin, and TMC-264 under the growing cells system,12,17,19 mainly because premature resin addition can adsorb nutrients and thus lead to a decrease in biomass. However, this situation did not exist in this resting cell system and early resin addition provided an excellent protective microenvironment for bioconversion by adsorbing toxic products.
Table 3 Effects of different addition times of resin D101 on 9α-OH-AD production, space–time yield and phytosterol consumptiona
D101 addition times (h) |
9α-OH-AD in supernatant (g L−1) |
9α-OH-AD in resins (g L−1) |
Total 9α-OH-AD (g L−1) |
Conversion rate of PS (%) |
Space–time yield (g L−1 d−1) |
The D101 resin was added at a concentration of 5 g L−1. All experiments contained 50 g L−1 phytosterols, 100 g L−1 wet biomass, 200 g L−1 HP-β-CD, 10 mL reaction solution bioconverted in 250 mL shake flasks for 3 d (error bars for standard deviations, n = 3). |
CK |
20.74 ± 0.54 |
— |
20.74 ± 0.74 |
85.30 ± 0.39 |
6.91 ± 0.24 |
0 |
24.75 ± 0.43 |
0.78 ± 0.18 |
25.53 ± 0.23 |
100 ± 0.00 |
8.51 ± 0.15 |
12 |
23.72 ± 0.54 |
0.55 ± 0.08 |
24.27 ± 0.23 |
95.2 ± 0.37 |
8.09 ± 0.11 |
24 |
22.24 ± 0.64 |
0.57 ± 0.02 |
22.81 ± 0.50 |
92.02 ± 0.49 |
7.60 ± 0.07 |
36 |
21.25 ± 0.17 |
0.55 ± 0.07 |
21.80 ± 0.67 |
86.98 ± 0.43 |
7.27 ± 0.12 |
48 |
20.44 ± 0.65 |
0.31 ± 0.04 |
20.75 ± 0.38 |
84.16 ± 0.55 |
6.92 ± 0.10 |
60 |
20.39 ± 0.52 |
0.22 ± 0.01 |
20.61 ± 0.73 |
82.30 ± 0.83 |
6.87 ± 0.39 |
In this section, macroporous adsorbent resin D101, which favourably produced 9α-OH-AD, was selected and the conditions for its use were optimized. The results showed that the optimum time for the addition of D101 was at the beginning of the reaction, and the most suitable concentration was 5 g L−1.
3.3 Analysis of the mechanism of D101 in improving the bioconversion process
3.3.1 Separation and identification of putative inhibitory intermediates. The aforementioned results indicated that the adsorption of 9α-OH-AD by D101 was not a key factor in improving the space–time yield, since only 3.1% of the total 9α-OH-AD yield was adsorbed by the resin when 5 g L−1 D101 was added. Multiple types of metabolites produced during bioconversion were adsorbed by the resins. To better understand the effect of resin adsorption on space–time yield, the putative inhibitor was separated and purified by silic gel chromatograph, and identified using ultra-performance liquid chromatography-tandem mass spectrometry (UPLC-MS/MS) to determine the molecular weight and fragmentation pattern of these compounds (Fig. S1†). As described in Table 4, these compounds are all intermediates of PS bioconversion, and 4-ene-3-keto steroids, which were the first intermediate of PS conversion through an oxidation reaction catalysed by cholesterol oxidases (ChoMs) (Fig. 2), showed the largest adsorption rate in the intermediates. Moreover, 4-ene-3-keto steroids was detected only in the resin and not in the supernatant when the process was treated with resin. Other intermediates, such as AD and DHC, mostly remained in the reaction system. Therefore, we speculated that D101 enhanced the space–time yield of 9-OH-AD mainly by the adsorption of 4-ene-3-keto steroids and alleviated its toxic effect on Mycobacterium cells.
Table 4 Proportion of phytosterol metabolites adsorbed by resin D101 after 3 d of bioconversion
Metabolites |
In supernatant (g L−1) |
In resins (g L−1) |
Absorption ratio (%) |
9α-OH-AD |
24.75 ± 0.33 |
0.78 ± 0.02 |
3.05 ± 0.04 |
AD |
0.46 ± 0.04 |
0.06 ± 0.01 |
11.54 ± 0.11 |
DHBC |
2.99 ± 0.03 |
0.14 ± 0.02 |
4.47 ± 0.09 |
DHC |
2.17 ± 0.17 |
0.29 ± 0.02 |
11.79 ± 0.23 |
4-Ene-3-keto steroids |
— |
0.05 ± 0.01 |
100 ± 0.00 |
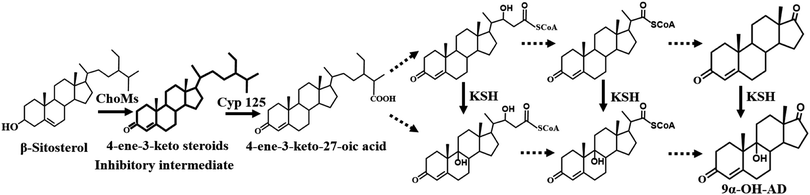 |
| Fig. 2 Degradation pathway of M. neoaurum HK86 for sitosterol, according to ref. 5 and 21. ChoMs: cholesterol oxidase, Cyp125: steroid C27-monooxygenase, KSH: 3-ketosteroid-9α-hydroxylase. The black dashed arrows indicate multi-step enzyme-catalyzed side chain degradation reactions. | |
3.3.2 Effect of 4-ene-3-keto steroids on bioconversion process. The quantitative characterization of 4-ene-3-keto steroids using the relative concentrations method (referred Fig. S2†) is shown in Fig. 3A. In the control group, the accumulation of 4-ene-3-keto steroids was observed only at 12 h and was not detectable after 24 h of reaction, indicating that 4-ene-3-keto steroids only accumulated at the beginning of the bioprocess and did not have a clear effect in the middle and late stages of bioconversion. Notably, there was no 4-ene-3-keto steroids detected in the supernatant after treatment with D101 at 0 h, and all of them were adsorbed by the resin. Moreover, the amount of 4-ene-3-keto steroids adsorption increased with an increase in the amount of resin, and when the system was treated with 30 g L−1 of D101, the amount of 4-ene-3-keto steroids adsorption reached 3 times that attained with 5 g L−1 of D101. However, the excess adsorption of 4-ene-3-keto steroids reduced the 9α-OH-AD yield and the conversion rate of PS (Table 2). The initial reaction rate after the addition of resin was evaluated (Fig. 3B). When 5 g L−1 D101 was added at 0 h, the initial reaction rate was enhanced by 23.44% compared to the control group (17.28 g L−1 d−1), reaching 21.33 g L−1 d−1. Interestingly, there were no significant differences in the initial reaction rates at different resin concentrations. These data demonstrated that the accumulation of 4-ene-3-keto steroids was closely related to the production efficiency of 9α-OH-AD, and the removal of 4-ene-3-keto steroids by resin adsorption markedly increased the initial reaction rate of the bioprocess. However, the excess adsorption of 4-ene-3-keto steroids by the resin in the process was detrimental to further degradation of sterol side chains as an intermediate metabolite, and 5 g L−1 of D101 resin was optimum for 9α-OH-AD production in this system.
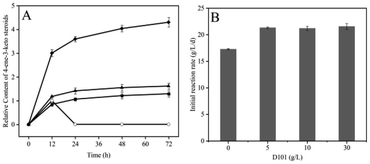 |
| Fig. 3 Quantitative characterization of 4-ene-3-keto steroids in the absence or presence of resin D101 (A), and effect of resin adsorption of 4-ene-3-keto steroids on initial reaction rate (B). The 4-ene-3-keto steroids content in the 12 hour sample in the absence of resin was set to 1, and the initial reaction rate indicates the consumption of PS at 1 d. ○, control group, no resin added; ■, 4-ene-3-keto steroids in 5 g L−1 D101; ▲, 4-ene-3-keto steroids in 10 g L−1 D101; ◆, 4-ene-3-keto steroids in 30 g L−1 D101. 4-Ene-3-keto steroids was not detected in the supernatant ingredients after the addition of resin and is not depicted in the figure. All experiments contained 50 g L−1 phytosterols, 100 g L−1 wet biomass, 200 g L−1 HP-β-CD, 10 mL reaction solution bioconverted in 250 mL shake flasks for 3 d (error bars for standard deviations, n = 3). | |
To verify if 4-ene-3-keto steroids accumulation could have an inhibitory effect on bioconversion, we exogenously supplemented 4-ene-3-keto steroids and detected its inhibitory effect on 9α-OH-AD production. The initial reaction rate decreased significantly in the presence of exogenous 4-ene-3-keto steroids (Fig. 4), and the higher the concentration of 4-ene-3-keto steroids, the lower the initial reaction rate. The initial reaction rate decreased by 19.39% to 13.93 g L−1 d−1 after adding the 4-ene-3-keto steroids at a relative concentration of 1 (4-ene-3-keto steroids content of the control group at 12 h was set to a relative concentration of 1). These results suggested that the accumulation of 4-ene-3-keto steroids had an inhibitory effect on the biotransformation process, thus reducing the space–time yield of 9α-OH-AD.
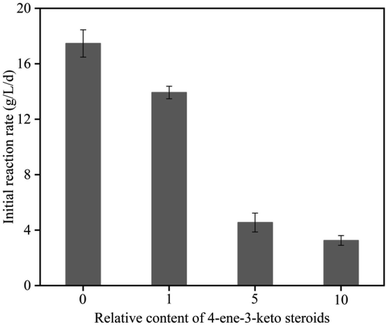 |
| Fig. 4 Effect of exogenous addition of 4-ene-3-keto steroids on initial reaction rate. The 4-ene-3-keto steroids content in the 12 hour sample was set to a relative concentration of 1 in the absence of resin, and the initial reaction rate indicates the consumption of PS at 1 d. Different amounts of 4-ene-3-keto steroids were added in reaction solution at the beginning of bioconversion. All experiments contained 50 g L−1 phytosterols, 100 g L−1 wet biomass, 200 g L−1 HP-β-CD, 10 mL reaction solution bioconverted in 250 mL shake flasks for 1 d (error bars for standard deviations, n = 3). | |
The generation of 4-ene-3-keto steroids is the initial step in the catabolism of PS by Mycobacterium neoaurum and has been demonstrated to be the rate-limiting step in PS bioconversion.22 The augmentation of cholesterol oxidase expression levels is a commonly used method to enhance the formation of 4-ene-3-keto steroids, but the increased response rate was in turn inhibited by feedback from the excessive accumulation of 4-ene-3-keto steroids. Therefore, enhancing cholesterol oxidase activity alone did not result in a sustained improvement in PS bioconversion. In this study, we demonstrated that the adsorption of 4-ene-3-keto steroids by resin accompanied with the reaction progress was a key method to help mitigate 4-ene-3-keto steroids toxicity, and achieving a balance between 4-ene-3-keto steroids production and consumption was a key factor affecting PS bioconversion.
4. Conclusions
PS bioconversion to 9α-OH-AD proceeded in a series of bioreactions and produced many intermediates that putatively inhibited cell activity. Mechanistic probing revealed that the increased space–time yield of 9α-OH-AD was mainly achieved by in situ removal of 4-ene-3-keto steroids, which is the first intermediate metabolite of sterol degradation, to alleviate its feedback inhibition rather than the adsorption of the final product 9α-OH-AD. A method of in situ adsorption of the toxic intermediate metabolite using D101 resin as a carrier in a cyclodextrin-resting cell system was first used to promote the production efficiency of 9α-OH-AD. We evaluated the types and amounts of resins added to the reaction system and optimized the addition time of the resins. The results revealed that D101 resin was the most suitable adsorption carrier for this ISPR system, and the space–time yield of 9α-OH-AD was markedly improved when it was added at a concentration of 5 g L−1 at the beginning of PS bioconversion. This study indicated that the in situ removal of the inhibitory intermediate is a potential candidate for producing 9α-OH-AD under the cyclodextrin-resting cell system at high substrate concentrations.
Conflicts of interest
The authors declare no conflict of interest.
Acknowledgements
This work was supported by the National Natural Science Foundation of China (31570079, 21706072).
References
- W. Y. Tong and X. Dong, Recent Pat. Biotechnol., 2009, 3, 141–153 CrossRef CAS.
- R. Batth, C. Nicolle, I. S. Cuciurean and H. T. Simonsen, Plants, 2020, 9, 1144 CrossRef CAS PubMed.
- M. V. Donova and O. V. Egorova, Appl. Microbiol. Biotechnol., 2012, 94, 1423–1447 CrossRef CAS.
- X. Q. Gao, J. X. Feng, Q. Hua, D. Z. Wei and X. D. Wang, Biocatal. Biotransform., 2014, 32, 343–347 CrossRef CAS.
- K. Yao, L. Q. Xu, F. Q. Wang and D. Z. Wei, Metab. Eng., 2014, 24, 181–191 CrossRef CAS.
- L. Su, S. Xu, Y. Shen, M. Xia, X. Ren, L. Wang, Z. Shang and M. Wang, Appl. Environ. Microbiol., 2020, 86, e00441-20 CrossRef PubMed.
- J. J. Yuan, Y. X. Guan and S. J. Yao, ACS Sustainable Chem. Eng., 2017, 5, 10702–10709 CrossRef CAS.
- R. A. Mancilla, C. Little and A. Amoroso, Appl. Biochem. Biotechnol., 2018, 185, 494–506 CrossRef CAS.
- L. Zhou, H. Li, Y. Xu, W. Liu, X. Zhang, J. Gong, Z. Xu and J. Shi, Process Biochem., 2019, 87, 89–94 CrossRef CAS.
- V. Y. Shtratnikova, M. I. Schelkunov, D. V. Dovbnya, E. Y. Bragin and M. V. Donova, Appl. Microbiol. Biotechnol., 2017, 101, 4659–4667 CrossRef CAS.
- S. M. Khomutov, G. V. Sukhodolskaya and M. V. Donova, Biocatal. Biotransform., 2009, 25, 386–392 CrossRef.
- M. A. Molchanova, V. A. Andryushina, T. S. Savinova, T. S. Stytsenko, N. V. Rodina and N. E. Voishvillo, Russ. J. Bioorg. Chem., 2007, 33, 354–358 CrossRef CAS PubMed.
- W. H. Liu, C. W. Kuo, K. L. Wu, C. Y. Lee and W. Y. Hsu, J. Ind. Microbiol. Biotechnol., 1994, 13, 167–171 CrossRef CAS.
- C. Y. Lee, C. D. Chen and W. H. Liu, Appl. Microbiol. Biotechnol., 1993, 38, 447–452 CrossRef CAS.
- J. T. Dafoe and A. J. Daugulis, Biotechnol. Lett., 2014, 36, 443–460 CrossRef CAS PubMed.
- A. G. Santos, T. L. Albuquerque, B. D. Ribeiro and M. A. Z. Coelho, Biotechnol. Appl. Biochem., 2020, 1–14 Search PubMed.
- J. Zhao, Y. Li, T. Shan, Y. Mou and L. Zhou, World J. Microbiol. Biotechnol., 2011, 27, 2753–2758 CrossRef CAS.
- S. W. Han, Y. R. Choi and J. S. Shin, Catal. Lett., 2021 DOI:10.1007/s10562-021-03535-6.
- H. Luo, H. Liu, Y. Cao, D. Xu, Z. Mao, Y. Mou, J. Meng, D. Lai, Y. Liu and L. Zhou, Molecules, 2014, 19, 14221–14234 CrossRef.
- X. Q. Gao, J. X. Feng, X. D. Wang, Q. Hua and D. Z. Wei, Chem. Biochem. Eng. Q., 2015, 29, 567–573 CrossRef CAS.
- J. K. Capyk, R. Kalscheuer, G. R. Stewart, J. Liu, H. Kwon, R. Zhao, S. Okamoto, W. R. Jacobs, L. D. Eltis and W. W. Mohn, J. Biol. Chem., 2009, 284, 35534–35542 CrossRef CAS.
- K. Yao, F. Q. Wang, H. C. Zhang and D. Z. Wei, Metab. Eng., 2013, 15, 75–87 CrossRef CAS PubMed.
Footnotes |
† Electronic supplementary information (ESI) available: Experimental details, Table S1, Fig. S1 and S2. See DOI: 10.1039/d1ra02774c |
‡ These authors contributed equally to this work. |
|
This journal is © The Royal Society of Chemistry 2021 |
Click here to see how this site uses Cookies. View our privacy policy here.