DOI:
10.1039/D1RA02828F
(Paper)
RSC Adv., 2021,
11, 19630-19638
Heterostructure Ni3S4–MoS2 with interfacial electron redistribution used for enhancing hydrogen evolution†
Received
12th April 2021
, Accepted 17th May 2021
First published on 1st June 2021
Abstract
Developing highly effective and inexpensive electrocatalysts for hydrogen evolution reaction (HER), particularly in a water-alkaline electrolyzer, are crucial to large-scale industrialization. The earth-abundant molybdenum disulfide (MoS2) is an ideal electrocatalyst in acidic media but suffers from a high overpotential in alkaline solution. Herein, nanospherical heterostructure Ni3S4–MoS2 was obtained via a one-pot synthesis method, in which Ni3S4 was uniformly integrated with MoS2 ultrathin nanosheets. There were abundant heterojunctions in the as-synthesized catalyst, which were verified by X-ray photoelectron spectroscopy (XPS) and high-resolution transmission electron microscopy (HRTEM). The structure features with interfacial electron redistribution was proved by XPS and density functional theory (DFT) calculations, which offered several advantages to promote the HER activity of MoS2, including increased specific surface area, exposed abundant active edge sites and improved electron transfer. Ni3S4–MoS2 exhibited a low overpotential of 116 mV at 10 mA cm−2 in an alkaline solution with a corresponding Tafel slope of 81 mV dec−1 and long-term stability of over 20 h. DFT simulations indicated that the synergistic effects in the system with the chemisorption of H on the (002) plane of MoS2 and OH on the (311) plane of Ni3S4 accelerated the rate-determining water dissociation steps of HER. This study provides a valuable route for the design and synthesis of inexpensive and efficient HER electrocatalyst, heterostructure Ni3S4–MoS2.
Introduction
With the increasing environmental protection demands, developing sustainable and fossil-free renewable energy plays a major role.1 Due to its environmentally friendly, zero-emission, high-energy capacity and sustainable merits, hydrogen (H2) has received extensive attention.2 The most efficient method to generate H2 (2H2O → 2H2 + O2) is water splitting by electric power generated from renewable energy sources.3–5 Currently, some noble metals, such as Pt, Rh and Ir, have been proved to possess excellent catalytic performance as HER electrocatalysts, including low overpotential, low Tafel slope and low impedance.6,7 However, the exorbitant cost and limited earth abundance of these noble metal materials have hindered their industrialization and commercialization.8 Therefore, developing electrocatalysts that are low cost, highly active and stable from nonprecious and earth-abundant metal materials is urgent.
In recent years, earth-abundant 2D MoS2 has been considered as an ideal alternative to the precious Pt-based catalysts as the next-generation electrocatalytic material due to its unique structure and chemical properties.9–11 DFT calculations indicated that MoS2 exhibits excellent HER performance in acidic solutions since its edge sites permit a near-optimal hydrogen adsorption free energy (ΔGH* = 0.08 eV).12 Moreover, the tremendous amount of S sites in the basal plane of pure MoS2 are quite inert and not sufficiently utilized.13 Unfortunately, MoS2 has also been found to have poor activity in alkaline media,14 even though alkaline catalysis is a more widespread application. Numerous strategies have been employed to improve the catalytic activity of 2D MoS2, such as generating the sulfur vacancies,15 introducing heteroatoms,16 changing conductive supports.17 It has been both experimentally and theoretically identified that the fabrication of heterogeneous nanostructures with abundant and accessible exposed active sites is a very effective way for improving the catalytic activity.18
MoS2 decorated with transition metals, such as Fe, Co and Ni, by heterostructure engineering has shown excellent electrocatalytic performance.19 Because of the versatile electronic structure of these metals and the ability to fill d orbitals with electrons from another transition metal, they provide distinctive catalytic properties. For example, nickel-based catalysts have been shown to have impressive potential for HER electrocatalysts due to their conductivity and low cost.20,21 Some reports have indicated that constructing heterostructure for MoS2 via introducing nickel sulfides (e.g., Ni3S2,22 NiS2 (ref. 23) and NiS24) could provide superior HER activity. However, the intrinsic motivation of the enhanced catalytic performance is not clear. Moreover, the impact of constructing a heterogeneous structure via introducing Ni3S4 into MoS2 on HER performance remains to be studied. Moreover, an in-depth understanding of the interfacial electron redistribution for the improved HER performance is important for its wide application.
In this study, we fabricated the heterostructure Ni3S4–MoS2 with a nanospherical morphology via a one-step hydrothermal strategy and applied it as an HER catalyst. To improve the electronic conductivity and expose abundant active edge sites of MoS2, we constructed the heterostructure via introducing Ni3S4 into MoS2 ultra-thin nanosheets, which significantly enhanced the HER activity. The heterostructure of Ni3S4–MoS2 was investigated via XPS, SEM and HRTEM techniques. For researching the electron redistribution on the interface of Ni3S4–MoS2 and the mechanism of electro-catalysis during HER, we also applied the DFT simulation. It is indicated that the heterostructure Ni3S4–MoS2 optimized water dissociation energies and H* absorption free energy.
Experimental section
Materials
Sodium molybdate (VI) dihydrate (Na2MoO4·2H2O), nickel(II) chloride hexahydrate (NiCl2·6H2O), urea, sodium sulfide nonahydrate (Na2S·9H2O) and thiourea (CH4N2S) were obtained from Beijing Chemical Works. A 5 wt% Nafion solution was obtained from Du Pont China Holding Co., Ltd. A nickel foam (NF) having a thickness of 0.5 mm was bought from Changsha Lyrun New Materials Co., Ltd., and it (cut as 1.0 cm × 1.0 cm in size) was washed with hydrochloric acid, ethanol and deionized water. All other chemicals were of analytical grade and were purchased from Sinopharm Chemical Reagents Co. All chemicals were used without further purification.
Synthesis of MoS2, Ni4S3–MoS2 and Ni4S3 nanosheets
3.5 mmol (0.847 g) of Na2MoO4·2H2O and 15 mmol (1.14 g) of thiourea were dissolved in 30 mL of deionized water and dispersed by ultrasonication for 10 min to form a uniform solution. The mixture was transferred into a 50 mL Teflon-lined stainless-steel autoclave and maintained at 180 °C for 24 h. The as-prepared MoS2 was washed with deionized water and ethanol several times and then dried in a vacuum at 60 °C. Ni3S4–MoS2 was synthesized by a simple one-pot step method that was the same as the above process for MoS2. However, in the Na2MoO4·2H2O and thiourea mixed solution, different amounts of NiCl2·6H2O were added. Then, the Ni3S4–MoS2 nanosheets were obtained after the same hydrothermal treatment, washing and drying.
Ni3S4 was prepared according to the method in the reported literature.25 Briefly, 1.5 mmol (0.357 g) of NiCl2·6H2O and 10 mmol (0.60 g) of urea were dissolved in 30 mL deionized water, and the mixture was dispersed by ultrasonication for 10 min to form a uniform solution. The solution was then transferred into a 50 mL Teflon-lined stainless-steel autoclave and maintained at 130 °C for 2 h. The as-prepared Ni(OH)2 was washed with deionized water and ethanol several times and then dried in a vacuum at 60 °C. Then, the as-prepared Ni(OH)2 precursor together with 30 mL of 15 mmol (3.6 g) sodium sulfide (Na2S·9H2O) aqueous solution was placed in the Teflon-lined stainless-steel autoclave and maintained at 90 °C for 9 h. The as-prepared Ni3S4 was washed with deionized water and ethanol several times and then dried in a vacuum at 60 °C for 12 h.
Characterization
X-ray diffraction (XRD) patterns were collected on a Rigaku XRD-6000 diffractometer using Cu Kα radiation from 3° to 80° at the scan rate of 10° min−1. The morphologies were investigated via SEM (Zeiss SUPRA 55) at an accelerating voltage of 20 kV. A Brunauer–Emmett–Teller (BET, ASAP 2460) apparatus was used to measure the surface area. HRTEM images were recorded using a JEOL JEM-2010 field-emission transmission electron microscope at an accelerating voltage of 200 kV, combined with energy-dispersive X-ray spectroscopy (EDS). XPS measurements were performed on a Thermo VG ESCALAB 250 X-ray photoelectron spectrometer with Al Kα radiation at a pressure of about 2 × 10−9 Pa. Inductively coupled plasma-optical emission spectrometry (ICP-OES) was adopted to analyze the chemical components of the catalysts.
Electrochemical measurements
Electrochemical measurements were performed on an electrochemical workstation (CHI 660E, CH Instruments Inc., Chenhua, Shanghai) using a three-electrode mode in an Ar-saturated 1 mol L−1 KOH aqueous solution. A platinum electrode was used as the counter electrode, a sliver/sliver chloride (Ag/AgCl) electrode was used as the reference electrode, and the as-fabricated materials were used as the working electrodes. The potentials were converted to the RHE scale using the following Nernst equation: (E(RHE) = E(Ag/AgCl) + 0.059 pH + 0.197). To accelerate the electrochemical performance tests, 5 mg of the as-prepared catalysts, 2 mg conductive carbon, 35 μL of the 5 wt% Nafion solution and 1 mL anhydrous ethanol were mixed and ultrasonicated for 10 min to form homogeneous catalyst inks. The catalyst inks were dripped respectively onto the as-prepared NF to obtain the working electrodes with a loading of ∼5 mg cm−2, which were dried at 60 °C for 1 h. The electrochemical impedance spectroscopy (EIS) tests were performed in the frequency range from 100 kHz to 0.1 Hz at an overpotential of 180 mV. The cyclic voltammograms (CV) were obtained between 0.1 and −0.3 V vs. RHE at 100 mV s−1 to investigate the cycling stability. The long-term stability tests were recorded by taking a chronoamperometric curve current density that reached 10 mA cm−2. All data were presented without IR compensation, and all the electrochemical tests were tested at room temperature.
Computational methods
All first principles calculations were performed via DFT in the Cambridge Sequential Total Energy Package (CASTEP) module in the Materials Studio. The MoS2 (002) plane consisting of six layers of Mo and S, and the Ni3S4 (311) slab composed of six layers of atoms were constructed as our models, because the (002) plane of MoS2 and the (311) plane of Ni3S4 were dominant crystal faces from the HRTEM images. The exchange–correlation interactions were treated within the generalized gradient approximation of the Perdew–Burke–Ernzerhof (PBE) type. The plane-wave cutoff energy was 400 eV, and a k-mesh of 3 × 3 × 1 was adopted to sample the Brillouin zone. The convergence threshold for energy and Hellmann–Feynman forces on each atom were set to 10−5 eV and 0.01 eV Å−1. Vacuum layers of 15 Å were introduced to minimize interactions between adjacent layers in all supercells.15 All the atom positions in the model were optimized by the conjugate-gradient optimization procedure.
Gibbs free-energy of the adsorption atomic hydrogen was calculated as the following formula:
where Δ
E is the adsorption energy of adsorbed species on the given unit cell. Δ
ZPE and
TΔ
S are the zero-point energy and entropy difference of hydrogen in the adsorbed state and the gas phase, respectively. The value of
ZPE and
TS for the adsorbed species were calculated from the vibration frequencies, as shown in the previous literature.
15
Results and discussion
Morphology and structure of Ni3S4–MoS2
A series of Ni3S4–MoS2 with different Ni contents were synthesized via a one-pot hydrothermal method. The ICP-OES measurements provided the contents of Ni in Ni3S4–MoS2, as shown in Table S1.† The XRD patterns of the Ni3S4–MoS2 composite, pure Ni3S4 and MoS2 are shown in Fig. 1a. For the Ni3S4 and MoS2 samples, all of their peaks were well matching with the Ni3S4 (JCPDS no. 47-1739) and MoS2 phases (JCPDS no. 37-1492), respectively. For the XRD pattern of Ni3S4–MoS2, the diffraction peaks at 14.5°, 32.7° and 58.3° corresponded to the (002), (100) and (110) lattice planes of the MoS2 phase (JCPDS Card no. 37-1492).26 There are six sharp peaks at 16.2°, 26.6°, 31.3°, 37.9°, 50.0° and 54.7°, which can be indexed to the (111), (220), (311), (400), (511) and (440) planes of Ni3S4 (JCPDS no. 47-1739).27 The XRD diagram implied the hybrid of these two metal sulfides in Ni3S4–MoS2.
 |
| Fig. 1 (a) XRD patterns of Ni3S4–MoS2, Ni3S4 and MoS2. (b) SEM image of the Ni3S4–MoS2. (c) SEM image of the MoS2. (d) TEM image and (e) corresponding SAED pattern of Ni3S4–MoS2. (f) TEM image of Ni3S4–MoS2 and corresponding elemental mappings: Mo (g), S (h) and Ni (i). | |
The SEM image of Ni3S4–MoS2 identifies that the morphology of the sample is a hierarchical nanosphere composed of nanosheets with a size of about 1 μm and a thickness of about 30 nm (Fig. 1b). By comparison Fig. 1b with c, it is found that the nanosheets of Ni3S4–MoS2 are bigger and thinner than that of MoS2 (average size is about 0.6 μm and thickness is 40 nm, as shown in Fig. S1†). Besides, the heterostructure Ni3S4–MoS2 has a larger specific surface area (2.7 m2 g−1) than that of the pure MoS2 (0.6 m2 g−1). The information illustrates that the construction of the heterojunction increased the surface area of samples. The HRTEM images provide further details of the microstructure for the heterostructure Ni3S4–MoS2. The lattice spacing of 0.625 nm corresponds to the (002) plane of MoS2,28,29 and 0.28 nm corresponds to the (311) plane of Ni3S4,30 indicating that the sample consisted of Ni3S4 and MoS2. The HRTEM image suggests that the (002) plane of MoS2 and (311) plane of Ni3S4 constitute important heterointerfaces in the composite (Fig. 1d). The crystal structure of the Ni3S4–MoS2 composite was further verified by the select area electron diffraction (SAED) (Fig. 1e). It showed that the inner ring was strong and the outer ring pattern correspond to the (002) plane of the MoS2 crystal and the (311) plane of Ni3S4, respectively, matching well with the XRD spectra (Fig. 1a). The EDS elemental mapping of Ni3S4–MoS2 indicates that the Mo, S and Ni elements distributed uniformly in the entire nanosheets (Fig. 1f–i), and the contents of Mo, S and Ni of Ni3S4–MoS2 are consistent with the results of ICP-OES tests (Fig. S2 and Table S2†).
XPS spectra were obtained to confirm the chemical composition and valence state of atoms in Ni3S4–MoS2 and pure MoS2, as shown in Fig. 2. It shows the existence of C, Mo, S, Ni and O elements in Ni3S4–MoS2 and the presence of all the elements but no Ni signal in MoS2 (Fig. 2a). The O 1s signal was assigned to the absorbed O-containing species, such as O2, CO2 and H2O. The high-resolution Mo 3d spectra of Ni3S4–MoS2 directly evidence the concurrent presence of Mo3+ and Mo4+ (Fig. 2b) with four definite fitting peaks assigned to Mo3+ (3d3/2 at 231.8 eV and 3d5/2 at 228.6 eV) and Mo4+ (3d3/2 at 232.8 eV and 3d5/2 at 229.7 eV), respectively.17 The binding energies of Mo 3d5/2 and Mo 3d3/2 in Ni3S4–MoS2 are negative shifts (0.2 eV) compared with the corresponding peaks of pristine MoS2,31,32 indicating the lower valence state of Mo in Ni3S4–MoS2. Thus, the charge transfer behavior from Ni3S4 to MoS2 is determined. The change of Mo valence was ascribed to the electronegativity difference between Mo and Ni, where the introduced Ni3S4 led to the rearrangement of the electron cloud between Mo and S, thus, forming a new hybridized electronic state. The S 2p spectrum of Ni3S4–MoS2 (Fig. 2c) was deconvoluted into three peaks at 161.4 (S 2p3/2), 162.6 (S 2p1/2) and 164.8 eV (S2−), respectively, and the S 2p shifts to the lower binding energy (about 0.2 eV, S 2p3/2 and S 2p3/2) compared with the pristine MoS2 (Fig. 2c), indicating the lower valence state of S in Ni3S4–MoS2. The result was consistent with the previous reports for other transition metals decorated with MoS2.33,34 The lower valence state of S in Ni3S4–MoS2 than that in MoS2 indicated that S in Ni3S4–MoS2 had a higher electric charge density that was contributed to H adsorption, which helped to improve the HER properties. The Ni 2p spectrum of Ni3S4–MoS2, as shown in Fig. 2d, exhibits the apparent Ni 2p3/2 and 2p1/2 peaks at 854.8 and 872.1 eV, respectively, that are attributed to Ni2+, and the other peaks at 856.9 and 874.3 eV, attributed to Ni3+.21 Two satellite peaks at 862.1 and 879.6 eV attributed to Ni 2p3/2 and 2p1/2, respectively, were observed.35 It was further confirmed that the material contained compound Ni3S4,36 which is in agreement with the XRD and HRTEM measurements.
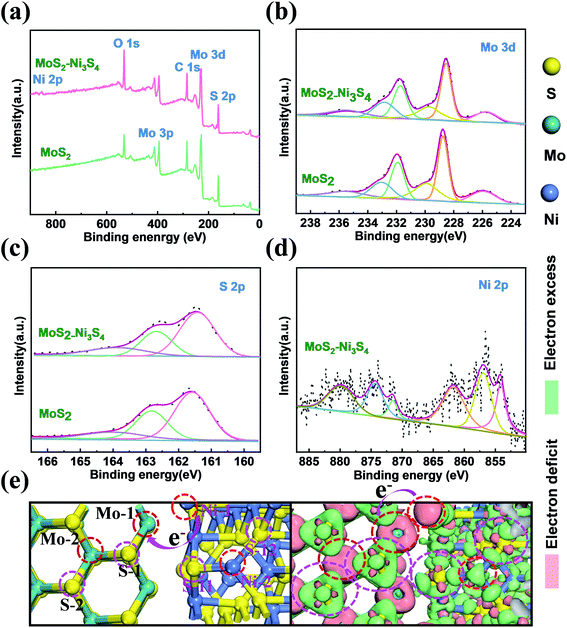 |
| Fig. 2 XPS spectra of Ni3S4–MoS2 and MoS2: (a) Survey spectra, (b) Mo 3d, (c) S 2p and (d) Ni 2p. (e) Top views of the most stable structure and charge density difference for the Ni3S4–MoS2. | |
To investigate the electron redistribution on the heterostructure of Ni3S4–MoS2, the electron density different diagram and the Bader charge analysis was performed by DFT calculations (Fig. 2e and Table S3†). The different electron density diagrams demonstrated that the electric charge densities of Mo and S increased on the interfaces Mo-1, S-1 and S-11 in Ni3S4–MoS2, compared with that on the base Mo-2, S-2 and S-12 in Ni3S4–MoS2, indicating that the binding energies of Mo and S in the heterojunction of Ni3S4–MoS2 reduced, which is in accordance with the XPS spectra and Bader charge analysis. The electric charge density of Ni on the interface Ni-1, compared with that on the base Ni-2 in Ni3S4–MoS2, was decreased, indicating that the electrons were transferred from Ni to Mo or S on the interfaces. These data confirmed the electron redistribution in the heterostructure Ni3S4–MoS2.
HER catalytic behavior
The HER activities of MoS2, Ni3S4–MoS2 and Ni3S4 on NF were measured in a 1 M KOH solution. There was a significant enhancement of the HER activity for Ni3S4–MoS2, as shown in Fig. 3a. The pure MoS2 exhibited an overpotential of 10 mA cm−2 (η10) at 235 mV, which is in agreement with the reported literature.37 The η10 of Ni3S4–MoS2 is 116 mV, which is much lower than that of the pure MoS2 (235 mV) and pristine Ni3S4 (318 mV). The heterostructure Ni3S4–MoS2 showed superior η10 in an alkaline solution and is comparable with the electrocatalysts reported in literature (see Table S4† for more details).38–40 The HER catalytic performance of the electrocatalysts with different Ni contents in Ni3S4–MoS2 was investigated, as shown in Fig. S3,† indicating that Ni3S4–MoS2 with 7.7 wt% Ni has the lowest overpotential (116 mV) at 10 mA cm−2 in the alkaline solution.
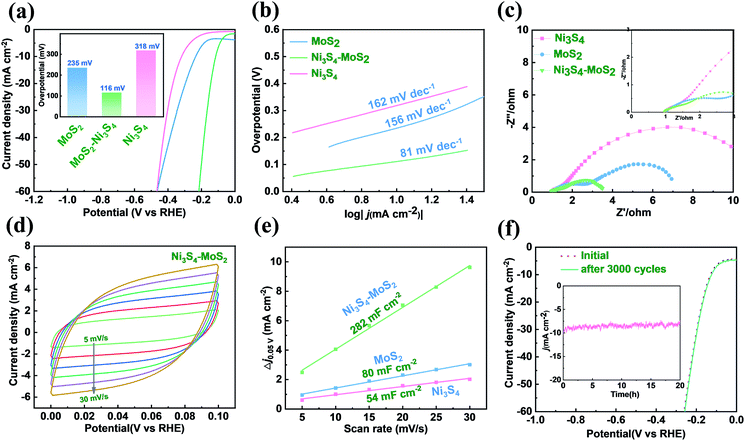 |
| Fig. 3 The HER behavior of Ni3S4–MoS2, Ni3S4 and MoS2 in 1 M KOH. (a) The LSV curves and overpotential (η10) without IR correction. (b) The Tafel slopes. (c) Nyquist plots collected at the overpotential of 180 mV. (d) CV curves of Ni3S4–MoS2 at the scan rates of 5, 10, 15, 20, 25 and 30 mV s−1, respectively. (e) Differences in current density variation (ΔJ = Ja − Jc) at 0.05 V vs. RHE plotted against scan rate fitted to linear regression for estimation of Cdl values of Ni3S4–MoS2, Ni3S4 and MoS2. (f) The initial and 3000th polarization curves of Ni3S4–MoS2. The inset is the chronoamperometric curve recorded at 10 mA for a continuous 20 h. | |
The Tafel curves of MoS2, Ni3S4–MoS2 and Ni3S4 on NF are shown in Fig. 3b. The Tafel slope of Ni3S4–MoS2 (81 mV dec−1) was much lower than that of MoS2 (156 mV dec−1), indicating that Ni3S4 played a key role in promoting the kinetics of HER. The EIS diagrams exhibited similar impedance characteristics, which implied similar electrochemical processes of these samples (Fig. 3c). Ni3S4–MoS2 showed a much lower charge-transfer-resistance (Rct) value when compared with the other catalysts, suggesting that Ni3S4–MoS2 had better charge-transfer property and HER kinetics. In addition, not only the electric conductivity but also the wettability of MoS2 was influenced by Ni3S4. The contact angle tests of the materials in 1 mol L−1 KOH electrolyte were carried out to explain such influence. The contact angle decreased from 21.36° for MoS2 to 15° for Ni3S4–MoS2 (Fig. S3†). It is shown that Ni3S4–MoS2 had better wettability in the KOH electrolyte than that of initial MoS2.
Electrochemical active surface area (ECSA) is a standard parameter applied in the evaluation of electrochemical catalysts. To investigate the exposed active sites, the ECSA of Ni3S4–MoS2, MoS2 and Ni3S4 were calculated by the double-layer capacitance (Cdl) through plotting CV curves. The CV curves of the samples were tested in the potential range of 0.0–0.1 V at the scan rates of 5, 10, 15, 20, 25 and 30 mV s−1 in 1.0 M KOH, respectively (Fig. 3d and S5†). The Cdl value of Ni3S4–MoS2 was 282 mF cm−2, which is much higher than that of MoS2 (80 mF cm−2) and Ni3S4 (54 mF cm−2) (Fig. 3e), indicating that the additional electrochemical active sites were generated after Ni3S4 was introduced. The Cdl value of Ni3S4–MoS2 was much more than that of MoS2 and Ni3S4, indicating there are more active sites exposed for HER.
To evaluate the long-term stability of the heterostructure Ni3S4–MoS2, it was subjected to 3000 continuous CV cycles in an alkaline environment from 0 to −0.3 V vs. RHE. The LSV curves had no clear changes before and after 3000 CV cycles (Fig. 3f), indicating that Ni3S4–MoS2 has excellent catalytic stability during the electrochemical process. Besides, Ni3S4–MoS2 has a stable HER current at a constant current of 10 mA versus time over a 20 h period in 1 M KOH (Fig. 3f). Simultaneously, the morphology of Ni3S4–MoS2 was well preserved (Fig. S6†), demonstrating excellent catalytic stability during the alkaline HER process.
Mechanism of Ni3S4–MoS2 for HER
According to literature,41,42 DFT calculations were also carried out to gain insight into the underlying mechanism of Ni3S4–MoS2 towards the HER activity. In an alkaline medium, the HER reaction mainly includes three steps: water adsorbed on the catalyst, water dissociation, H* formation and H2 generation.43,44 The water dissociation step is considered as the important step for the HER catalytic property in an alkaline solution. The chemisorption free energies of OH (ΔEOH) and H (ΔEH) on the different sites of Ni3S4–MoS2 were calculated, respectively. To study the optimal chemisorption free energies (ΔE), several feasible positions were chosen for the adsorption of OH and H (Fig. S7†). The chemisorption free energy of H adsorbed on the (002) plane of MoS2 (ΔEH = −0.81 eV) was lower than that absorbed on the (311) plane of Ni3S4 (ΔEH = −0.027 eV), indicating that H was inclined to be adsorbed on the (002) plane of MoS2. Compared with the (002) plane of MoS2 (ΔEOH = −3.0 eV), the (311) plane of Ni3S4 showed a predominant binding energy towards OH (ΔEOH = −4.8 eV), which is attributed to the bonding ability between OH and Ni (Fig. 4a). Therefore, OH is intensely adsorbed on the (311) plane of Ni3S4 and H on the (002) plane of MoS2. The appropriate oxidation of Ni in Ni3S4–MoS2 contributes to the adsorption energy of OH. Simultaneously, the partial reduction of S in Ni3S4–MoS2 is beneficial for the adsorption of H. It demonstrated a synergistic effect of Ni3S4–MoS2 with chemisorption of H (on the (002) plane of MoS2) and OH (on the (311) plane of Ni3S4) accelerated the rate-determining water dissociation steps of HER.
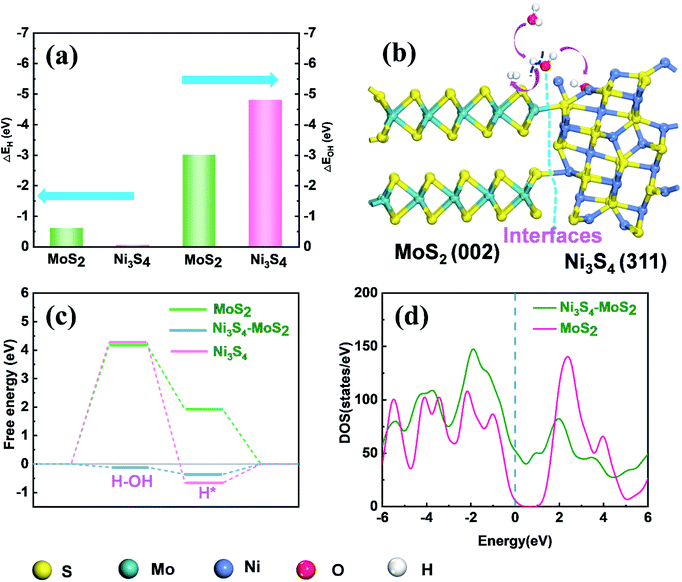 |
| Fig. 4 (a) DFT-calculated adsorption energies of H and OH at different sites on the surfaces of Ni3S4–MoS2, respectively. (b) The illustration of a mechanism for the electrocatalytic HER under alkaline conditions. (c) Free energy diagrams on the surface of MoS2, Ni3S4 and Ni3S4–MoS2 in alkaline solution. (d) DOSs of pristine MoS2 and Ni3S4–MoS2. | |
The free energy diagrams on the surfaces of MoS2, Ni3S4 and Ni3S4–MoS2 are shown in Fig. S8.† The ΔGH* of Ni3S4–MoS2 was −0.36 eV, which was much lower than that of pure MoS2 (1.92 eV), indicating the superior capacity of Ni3S4–MoS2 for H* adsorption (Table S4†), which benefited from the electron redistribution between Ni3S4 and MoS2. For pure MoS2, the free energy barrier of water dissociation ΔGH2O is as high as 4.2 eV, which distinctly hindered the dissociation of H2O to H*. Moreover, the ΔGH2O of Ni3S4–MoS2 was only −0.10 eV, which was much lower than that of MoS2 (4.2 eV) and Ni3S4 (4.3 eV). It is indicated that the ΔGH2O of Ni3S4–MoS2 efficiently decreased because of the existence of a heterostructure. Hence, the HER process on Ni3S4–MoS2 is highly accelerated and in accordance with our experimental results.
Moreover, the heterostructure improved the electrical transport efficiency of Ni3S4–MoS2. It is found that the total density of state (DOS) curve of MoS2 shows a clear band gap at the region around 0 eV, confirming the typical semiconductor characteristic. The peak of the valence band of the heterostructure Ni3S4–MoS2 is close to 0 eV (Fig. 4d), leading to the enhanced excitation of charge carriers to the conduction band and showing better electric conductivity, which is consistent with the EIS tests.
Conclusions
In summary, we fabricated the sphere-shaped heterostructure Ni3S4–MoS2 by a one-pot hydrothermal method. The as-synthesized catalyst with the activated interfaces generated abundant active sites and improved the electrical transport efficiency. Benefiting from engineering the heterostructure, the Ni3S4–MoS2 demonstrated the low overpotential of 116 mV with the corresponding Tafel slope of 81 mV dec−1 and long-term stability of over 20 h. DFT calculations proved that the heterostructure Ni3S4–MoS2 resulted in electron redistribution, which indicated the presence of a synergistic effect with MoS2 as the hydrogen acceptor and Ni3S4 as the hydroxyl acceptor, and effectively reduced the intermediate energy barrier of the water dissociation. Hence showing outstanding HER performance in alkaline solution. This work opens the door to develop low-cost and high-activity HER electrocatalyst Ni3S4–MoS2 via heterostructure engineering.
Conflicts of interest
The authors declare no competing financial interest.
Acknowledgements
This work was supported by the National Natural Science Foundation of China (nos 2177060378, 21978023, 21627813 and 21521005), the Program for Changjiang Scholars, Innovative Research Teams in Universities (No. IRT1205), and the Fundamental Research Funds for the Central Universities (No. 12060093063 and XK1803-05).
References
- Z. W. Seh, J. Kibsgaard, C. F. Dickens, I. Chorkendorff, J. K. Norskov and T. F. Jaramillo, Combining theory and experiment in electrocatalysis: Insights into materials design, Science, 2017, 355, eaad4998 CrossRef PubMed.
- M. S. Dresselhaus and I. L. Thomas, Alternative energy technologies, Nature, 2001, 414, 332–337 CrossRef CAS PubMed.
- J. A. Turner, Sustainable hydrogen production, Science, 2004, 305, 972–974 CrossRef CAS PubMed.
- V. R. Stamenkovic, D. Strmcnik, P. P. Lopes and N. M. Markovic, Energy and fuels from electrochemical interfaces, Nat. Mater., 2017, 16, 57–69 CrossRef CAS PubMed.
- Y. Zheng, Y. Jiao, A. Vasileff and S.-Z. Qiao, The Hydrogen Evolution Reaction in Alkaline Solution: From Theory, Single Crystal Models, to Practical Electrocatalysts, Angew. Chem., Int. Ed., 2018, 57, 7568–7579 CrossRef CAS PubMed.
- M. Zeng and Y. Li, Recent advances in heterogeneous electrocatalysts for the hydrogen evolution reaction, J. Mater. Chem. A, 2015, 3, 14942–14962 RSC.
- Y. Xu and B. Zhang, Recent advances in porous Pt-based nanostructures: synthesis and electrochemical applications, Chem. Soc. Rev., 2014, 43, 2439–2450 RSC.
- H. Zhang, P. An, W. Zhou, B. Y. Guan, P. Zhang, J. Dong and X. W. D. Lou, Dynamic traction of lattice-confined platinum atoms into mesoporous carbon matrix for hydrogen evolution reaction, Sci. Adv., 2018, 4, eaao6657 CrossRef PubMed.
- J. K. Norskov and C. H. Christensen, Chemistry - Toward efficient hydrogen production at surfaces, Science, 2006, 312, 1322–1323 CrossRef CAS PubMed.
- X. Zhang, Z. Lai, C. Tan and H. Zhang, Solution-Processed Two-Dimensional MoS2 Nanosheets: Preparation, Hybridization, and Applications, Angew. Chem., Int. Ed., 2016, 55, 8816–8838 CrossRef CAS PubMed.
- S. Shi, Z. Sun and Y. H. Hu, Synthesis, stabilization and applications of 2-dimensional 1T metallic MoS2, J. Mater. Chem. A, 2018, 6, 23932–23977 RSC.
- B. Hinnemann, P. G. Moses, J. Bonde, K. P. Jorgensen, J. H. Nielsen, S. Horch, I. Chorkendorff and J. K. Norskov, Biomimetic hydrogen evolution: MoS2 nanoparticles as catalyst for hydrogen evolution, J. Am. Chem. Soc., 2005, 127, 5308–5309 CrossRef CAS PubMed.
- T. F. Jaramillo, K. P. Jorgensen, J. Bonde, J. H. Nielsen, S. Horch and I. Chorkendorff, Identification of active edge sites for electrochemical H-2 evolution from MoS2 nanocatalysts, Science, 2007, 317, 100–102 CrossRef CAS PubMed.
- J. Hu, C. Zhang, L. Jiang, H. Lin, Y. An, D. Zhou, M. K. H. Leung and S. Yang, Nanohybridization of MoS2 with Layered Double Hydroxides Efficiently Synergizes the Hydrogen Evolution in Alkaline Media, Joule, 2017, 1, 383–393 CrossRef CAS.
- J. M. Zhang, X. P. Xu, L. Yang, D. J. Cheng and D. P. Cao, Single-Atom Ru Doping Induced Phase Transition of MoS2 and S Vacancy for Hydrogen Evolution Reaction, Small Methods, 2019, 3, 1900653 CrossRef CAS.
- Y. Huang, Y. Sun, X. Zheng, T. Aoki, B. Pattengale, J. Huang, X. He, W. Bian, S. Younan, N. Williams, J. Hu, J. Ge, N. Pu, X. Yan, X. Pan, L. Zhang, Y. Wei and J. Gu, Atomically engineering activation sites onto metallic 1T-MoS2 catalysts for enhanced electrochemical hydrogen evolution, Nat. Commun., 2019, 10, 982 CrossRef CAS PubMed.
- Y. Wang, F. Lu, K. Su, N. Zhang, Y. Zhang, M. Wang and X. Wang, Engineering Mo-O-C interface in MoS2@rGO via charge transfer boosts hydrogen evolution, Chem. Eng. J., 2020, 399, 126018 CrossRef CAS.
- Y. Liu, S. Jiang, S. Li, L. Zhou, Z. Li, J. Li and M. Shao, Interface engineering of (Ni, Fe)S2@MoS2 heterostructures for synergetic electrochemical water splitting, Appl. Catal., B, 2019, 247, 107–114 CrossRef CAS.
- Y. Li, Z. Yin, M. Cui, X. Liu, J. Xiong, S. Chen and T. Ma, Interface engineering of transitional metal sulfide–MoS2 heterostructure composites as effective electrocatalysts for water-splitting, J. Mater. Chem. A, 2021, 9, 2070–2092 RSC.
- M. Gong, D. Y. Wang, C. C. Chen, B. J. Hwang and H. J. Dai, A mini review on nickel-based electrocatalysts for alkaline hydrogen evolution reaction, Nano Res., 2016, 9, 28–46 CrossRef CAS.
- J. Bao, Y. Zhou, Y. Zhang, X. Sheng, Y. Wang, S. Liang, C. Guo, W. Yang, T. Zhuang and Y. Hu, Engineering water splitting sites in three-dimensional flower-like Co–Ni–P/MoS2 heterostructural hybrid spheres for accelerating electrocatalytic oxygen and hydrogen evolution, J. Mater. Chem. A, 2020, 8, 22181–22190 RSC.
- J. Cao, J. Zhou, Y. Zhang, Y. Wang and X. Liu, Dominating Role of Aligned MoS2/Ni3S2 Nanoarrays Supported on Three-Dimensional Ni Foam with Hydrophilic Interface for Highly Enhanced Hydrogen Evolution Reaction, ACS Appl. Mater. Interfaces, 2018, 10, 1752–1760 CrossRef CAS PubMed.
- X. Wang, L. Li, Z. Wang, L. Tan, Z. Wu, Z. Liu, S. Gai and P. Yang, NiS2/MoS2 on carbon cloth as a bifunctional electrocatalyst for overall water splitting, Electrochim. Acta, 2019, 326, 134983 CrossRef CAS.
- H. Jiang, K. Zhang, W. Li, Z. Cui, S.-A. He, S. Zhao, J. Li, G. He, P. R. Shearing and D. J. L. Brett, MoS2/NiS core-shell structures for improved electrocatalytic process of hydrogen evolution, J. Power Sources, 2020, 472, 228497 CrossRef CAS.
- H. Wang, M. Liang, D. Duan, W. Shi, Y. Song and Z. Sun, Rose-like Ni3S4 as battery-type electrode for hybrid supercapacitor with excellent charge storage performance, Chem. Eng. J., 2018, 350, 523–533 CrossRef CAS.
- C. Li, M. Liu, H. Ding, L. He, E. Wang, B. Wang, S. Fan and K. Liu, A lightly Fe-doped (NiS2/MoS2)/carbon nanotube hybrid electrocatalyst film with laser-drilled micropores for stabilized overall water splitting and pH-universal hydrogen evolution reaction, J. Mater. Chem. A, 2020, 8, 17527–17536 RSC.
- Y. Zhang, W. Sun, X. Rui, B. Li, H. T. Tan, G. Guo, S. Madhavi, Y. Zong and Q. Yan, One-Pot Synthesis of Tunable Crystalline Ni3S4@Amorphous MoS2 Core/Shell Nanospheres for High-Performance Supercapacitors, Small, 2015, 11, 3694–3702 CrossRef CAS PubMed.
- J. Deng, H. Li, J. Xiao, Y. Tu, D. Deng, H. Yang, H. Tian, J. Li, P. Ren and X. Bao, Triggering the electrocatalytic hydrogen evolution activity of the inert two-dimensional MoS2 surface via single-atom metal doping, Energy Environ. Sci., 2015, 8, 1594–1601 RSC.
- H. B. Zhang, L. Yu, T. Chen, W. Zhou and X. W. Lou, Surface Modulation of Hierarchical MoS2 Nanosheets by Ni Single Atoms for Enhanced Electrocatalytic Hydrogen Evolution, Adv. Funct. Mater., 2018, 28, 1807086 CrossRef.
- X. Hu, T. Li, Y. Tang, Y. Wang, A. Wang, G. Fu, X. Li and Y. Tang, Hydrogel-Derived Honeycomb Ni3S4/N, P-C as an Efficient Oxygen Evolution Catalyst, Chem. - Eur. J., 2019, 25, 7561–7568 CrossRef CAS PubMed.
- Y. Feng, T. Zhang, J. Zhang, H. Fan, C. He and J. Song, 3D 1T-MoS2/CoS2 Heterostructure via Interface Engineering for Ultrafast Hydrogen Evolution Reaction, Small, 2020, 16, 2002850 CrossRef CAS PubMed.
- S. Qin, T. Yao, X. Guo, Q. Chen, D. Liu, Q. Liu, Y. Li, J. Li and D. He, MoS2/Ni3S4 composite nanosheets on interconnected carbon shells as an excellent supercapacitor electrode architecture for long term cycling at high current densities, Appl. Surf. Sci., 2018, 440, 741–747 CrossRef CAS.
- Y. Zhao, S. Wei, F. Wang, L. Xu, Y. Liu, J. Lin, K. Pan and H. Pang, Hatted 1T/2H-Phase MoS2 on Ni3S2 Nanorods for Efficient Overall Water Splitting in Alkaline Media, Chem. - Eur. J., 2020, 26, 2034–2040 CrossRef CAS PubMed.
- A. Muthurasu, G. P. Ojha, M. Lee and H. Y. Kim, Zeolitic imidazolate framework derived Co3S4 hybridized MoS2–Ni3S2 heterointerface for electrochemical overall water splitting reactions, Electrochim. Acta, 2020, 334, 135537 CrossRef CAS.
- Y. Yang, M.-L. Li, J.-N. Lin, M.-Y. Zou, S.-T. Gu, X.-J. Hong, L.-P. Si and Y.-P. Cai, MOF-derived Ni3S4 Encapsulated in 3D Conductive Network for High-Performance Supercapacitor, Inorg. Chem., 2020, 59, 2406–2412 CrossRef CAS PubMed.
- K. Wan, J. Luo, C. Zhou, T. Zhang, J. Arbiol, X. Lu, B.-W. Mao, X. Zhang and J. Fransaer, Hierarchical Porous Ni3S4 with Enriched High-Valence Ni Sites as a Robust Electrocatalyst for Efficient Oxygen Evolution Reaction, Adv. Funct. Mater., 2019, 29, 1900315 CrossRef.
- J. Kibsgaard, Z. Chen, B. N. Reinecke and T. F. Jaramillo, Engineering the surface structure of MoS2 to preferentially expose active edge sites for electrocatalysis, Nat. Mater., 2012, 11, 963–969 CrossRef CAS PubMed.
- X. Hou, A. Mensah, M. Zhao, Y. Cai and Q. Wei, Facile controlled synthesis of monodispersed MoO3-MoS2 hybrid nanospheres for efficient hydrogen evolution reaction, Appl. Surf. Sci., 2020, 529, 147115 CrossRef CAS.
- P. Kuang, T. Tong, K. Fan and J. Yu, In Situ Fabrication of Ni–Mo Bimetal Sulfide Hybrid as an Efficient Electrocatalyst for Hydrogen Evolution over a Wide pH Range, ACS Catal., 2017, 7, 6179–6187 CrossRef CAS.
- Q. Liu, Z. Xue, B. Jia, Q. Liu, K. Liu, Y. Lin, M. Liu, Y. Li and G. Li, Hierarchical Nanorods of MoS2/MoP Heterojunction for Efficient Electrocatalytic Hydrogen Evolution Reaction, Small, 2020, 16, 2002482 CrossRef CAS PubMed.
- F. Lin, Z. Dong, Y. Yao, L. Yang, F. Fang and L. Jiao, Electrocatalytic Hydrogen Evolution of Ultrathin Co-Mo5N6 Heterojunction with Interfacial Electron Redistribution, Adv. Energy Mater., 2020, 10, 2002176 CrossRef CAS.
- J. Hu, J. Liu, Z. Chen, X. Ma, Y. Liu, S. Wang, Z. Liu and C. Huang, Insights into the mechanism of the enhanced visible-light photocatalytic activity of a MoS2/BiOI heterostructure with interfacial coupling, Phys. Chem. Chem. Phys., 2020, 22, 22349–22356 RSC.
- C. Hu, L. Zhang and J. Gong, Recent progress made in the mechanism comprehension and design of electrocatalysts for alkaline water splitting, Energy Environ. Sci., 2019, 12, 2620–2645 RSC.
- C. Zhu, S. Fu, Q. Shi, D. Du and Y. Lin, Single-Atom Electrocatalysts, Angew. Chem., Int. Ed., 2017, 56, 13944–13960 CrossRef CAS PubMed.
Footnote |
† Electronic supplementary information (ESI) available. See DOI: 10.1039/d1ra02828f |
|
This journal is © The Royal Society of Chemistry 2021 |
Click here to see how this site uses Cookies. View our privacy policy here.