DOI:
10.1039/D1RA04277G
(Paper)
RSC Adv., 2021,
11, 24410-24415
A ratiometric fluorescence probe for the selective detection of H2S in serum using a pyrene-DPA–Cd2+ complex†
Received
2nd June 2021
, Accepted 5th July 2021
First published on 12th July 2021
Abstract
A ratiometric and selective hydrogen sulfide (H2S) detection probe was proposed based on the pyrene-DPA–Cd2+ complex through the metal ion displacement approach (MDA) mechanism. While most MDA-based fluorescence probes with paramagnetic Cu2+ have focused on the development of a simple turn-on sensor using the broad spectral range of fluorescence enhancement, this ratiometric probe exhibited unchanged monomer emission as a built-in internal reference with an increase in excimer emission with added H2S. The demonstrated probe showed a rapid response (within 1 min) and a high sensitivity, with 70 nM as the limit of detection. The selectivity for H2S over cysteine, homocysteine and glutathione was confirmed, and reliable fluorescence enhancement, which could be monitored by the naked eye, was observed upon irradiation with handheld UV light. In addition, this detection system was successfully applied to detect H2S in human serum without interference from biological molecules.
Introduction
Hydrogen sulfide (H2S) is a gaseous signaling molecule that has received attention because of its toxicity for physiological functions in humans.1,2 Endogenous H2S, which is generated mainly from cysteine (Cys), homocysteine (Hcy), and 3-mercaptopyruvate by enzymatic reactions of cystathionine β-synthase, cystathioine-γ-lyase, and 3-mercaptopyruvate sufurtransferase/cysteine aminotransferase, is involved in various biological processes such as blood pressure regulation, metabolic disorders, neurodegeneration, and inflammation.3–8 Therefore, the imbalanced synthesis of endogenous H2S is related to some human diseases such as lung infection, Alzheimer's disease, and diabetes.9–11 Thus, abnormal levels of endogenous H2S in human serum can be used as evidence for such diseases. Considering the importance of H2S as a biomarker, various detection methods have been developed for the accurate measurement of changed endogenous H2S based on chromatography, electrochemical techniques, colorimetric, and fluorescent chemosensors.12–15 Among them, fluorescence detection methods have shown favorable properties such as rapid response, easy sample preparation, and high sensitivity, and various examples have been reported recently.
The currently known methods for fluorescent H2S detection were designed by using the chemical properties of H2S.16,17 These detection methods typically employ four types of probes depending on the recognition mechanism used by them; they may be based on nucleophilicity, reduction, metal coordination, or the metal displacement approach (MDA).18–21 MDA is a strategy that exploits fluorescence changes caused by the removal of metal ions, which coordinate to a fluorophore through metal ions and H2S complex formation. Owing to the coordination with charged metal ions and the high binding affinity between metal ions and H2S, MDA has several advantages over other mechanisms, including good water solubility, rapid response time, and high sensitivity.22 Therefore, MDA is a detection mechanism that is appropriate for application in various environments. Although some metal ions, including Cd2+, Zn2+, Hg2+, and Cu2+, show very low solubility products (Ksp) when bound to H2S, most proposed examples have focused on paramagnetic Cu2+ because fluorescence recovery through CuS complex formation promotes turn-on fluorescence detection after fluorophores are quenched by Cu2+ binding; this turn-on detection method displays fewer false positive signals and good distinguishability from the background compared to the turn-off method.23,24 Consequently, examples of MDA-based H2S detection using other metal ions have been limited to date.
Ratiometric fluorescence probes emit two or more at different wavelengths, and the ratio between the emissions is used to detect the target molecules. Ratiometric probes have unique features that enable self-calibration for the quantification of the target, and they also have built-in correction systems that reduce the environmental interference and enable them to have high signal-to-noise ratios.25,26 Therefore, numerous applications of these probes for various analytes have been developed, including H2S detection.27,28 However, despite the advantages that make MDA among the four typical H2S detection mechanisms, this mechanism has not garnered attention as a strategy for the development of ratiometric detection methods except for a little example.29,30 This is because quenching the entire spectral range of fluorescence by paramagnetic Cu2+ induces only simple fluorescence enhancement instead of ratiometric changes upon addition of H2S. In addition, the excessively strong interaction between thiol and Cu2+ causes other biothiols such as Cys, Hcy, and glutathione (GSH) to interfere with the reactions and reduce the selectivity of H2S detection, as shown in several previous studies.22 Thus, it is crucial to develop ratiometric fluorescent probes for the detection of H2S using other metal ions that retain the advantages of the MDA-based mechanism and also display great selectivity of H2S over other biothiols.
In this study, the pyrene-DPA–Cd2+ complex was proposed for use in a ratiometric fluorescence probe for H2S detection that is selective to other biothiols (Scheme 1). Previous studies performed in our group revealed that coordination of pyrene-DPA with Zn2+ decreased excimer emission without changing the monomer emission.31 Inspired by this result, it is expected that a complex of pyrene-DPA with a metal ion that has high binding affinity to H2S and quenches an excimer emission can be applied to develop ratiometric detection methods based on the MDA using the unchanged monomer as a built-in internal standard. Screening the response of the pyrene-DPA–metal ion complex in the presence of various biothiols, it was confirmed that Cd2+, which is in the same group as Zn2+, reduced only the excimer emission of pyrene-DPA; in particular, other biothiols apart from H2S did not influence any emissions in the pyrene-DPA–Cd2+ complex. Thus, the pyrene-DPA–Cd2+ complex could be used as a ratiometric probe for selective H2S detection over biothiols with high sensitivity and applicability in real serum samples, which were derived from the features of the MDA mechanism.
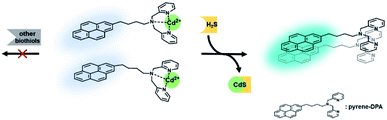 |
| Scheme 1 Schematic illustration of ratiometric and selective detection of H2S using Cd2+ displacement in a pyrene-DPA–Cd2+ complex. | |
Experimental
Materials and instrumentations
Chemical reagents were purchased from commercial sources (Sigma Aldrich, Alfa Aesar, Duksan, and Tokyo Chemical Industry) and used without further purification. Human serum was purchased from Sigma Aldrich (catalog number: H6914). 1H NMR spectra were recorded on a JEOL 400 MHz NMR spectrometer. Fluorescence spectra were measured on Agilent Cary Eclipse fluorescence spectrometer.
Synthesis of pyrene-DPA and in situ generation of pyrene-DPA–metal ion complex
The pyrene-DPA compound was prepared from previous literature of our group.31
For in situ pyrene-DPA–Cd2+ complex, same volume of pyrene-DPA and metal ion solution with same concentration were added to the buffer solution for each measurements and incubated for 5 min. After the incubation, various analytes were introduced and fluorescence or NMR spectra were obtained.
Screening for selection of an optimal metal ion and pH conditions
To pyrene-DPA–metal ion complex (Cd2+, Zn2+, Cu2+, and Hg2+, 20 μM) in buffer solution (20 mM, HEPES, pH 7.4), H2S (100 μM) and other biothiols solution (Cys, Hcy, and GSH, 100 μM) was added and emission spectra (λex = 341 nm) were recorded using fluorescence spectrometer. Pyrene-DPA–Cd2+ or Zn2+ complex (20 μM) was conducted by pyrophosphate (PPi) solution (50 μM) and fluorescence spectra were measured in the same procedure.
For selection of optimal pH condition, H2S and Cys (60 μM) were added to buffer solution (20 mM, pH 5.0 acetate, pH 6.0 MES, pH 7.0, 7.4, and 8.0 HEPES, pH 9.0 Tris) containing pyrene-DPA–Cd2+ complex (20 μM) and fluorescence spectra was recorded with excitation at 341 nm.
Sensitivity for detection of H2S
Various concentrations of H2S (0, 0.1, 0.2, 0.4, 0.6, 0.8, 1.0, 2.0, 4.0, 6.0, 8.0, 10.0, 12.0, 14.0, 16.0, 18.0, 20.0, 30.0, 40.0, 50.0, 60.0 μM) were added to buffer solution (20 mM, HEPES, pH 7.0) containing pyrene-DPA–Cd2+ complex (20 μM) and fluorescence spectra was obtained with excitation at 341 nm. Limit of detection (LOD) was estimated by 3σ/S using excimer enhancement against monomer emission from 0 to 1.0 μM H2S where σ is standard deviation of blank and S means slope from titration data for H2S detection.
Selectivity and interference test for detection of H2S
Biothiols (60 μM, H2S, Cys, Hcy, and GSH) and various anions (60 μM, F−, Cl−, Br−, I−, NO2−, NO3−, N3−, CN−, HSO4, CO32−, PO43−, and PPi) were added to buffer solution (20 mM, HEPES, pH 7.0) containing pyrene-DPA–Cd2+ complex (20 μM) and fluorescence spectra were recorded with excitation at 341 nm. For interference test, H2S (20 μM) was introduced to buffer solution (20 mM, HEPES, pH 7.0) containing pyrene-DPA–Cd2+ complex (20 μM) and other biothiol or anion (60 μM, Cys, Hcy, GSH, F−, Cl−, Br−, I−, NO2−, NO3−, N3−, CN−, HSO4, CO32−, PO43− and PPi), and fluorescence change was measured with excitation at 341 nm.
Mechanistic study for H2S detection based on metal ion displacement
To pyrene-DPA–Cd2+ complex (10 mM) in DMSO-d6, Cd2+ (10 mM) in D2O was added with or without subsequent addition of H2S (10 mM) in D2O and 1H-NMR was measured in DMSO-d6
:
D2O (8
:
2) with 400 MHz NMR spectrometer. The spectra of recorded 1H-NMR were compared in particular region.
Application of H2S detection in human serum samples
For preparation of deproteinized human serum sample, ammonium sulfate precipitation and subsequent centrifugal filtration were carried out. And the treated serum solution was spiked with various concentrations of H2S (6.0, 10.0, 12.0, 16.0, and 18.0 μM) and incubated for 5 min. The spiked serum sample was added to buffer solution (20 mM, HEPES, pH 7.0) containing pyrene-DPA–Cd2+ complex (20 μM) with final 5% serum and obtained fluorescence ratio at 476 and 376 nm was used to estimate found H2S concentrations.
Results and discussion
Screening for selection of an optimal metal ion and pH conditions
To develop a ratiometric probe for the selective detection of H2S over other biothiols, fluorescence responses through metal ion displacement of pyrene-DPA complexes were compared for various metal ions along with the addition of biothiols. In the case of Cu2+ and Hg2+, the entire fluorescence-containing monomer and excimer emission were reduced during complex formation between metal ions with pyrene-DPA (Fig. S1†). Therefore, they were not suitable for the development of ratiometric detection probes. Dramatic enhancement of excimer emission at 476 nm against monomer emission at 376 nm (F476/F376) was observed only in the pyrene-DPA–Zn2+ or Cd2+ complex with selectivity for H2S over Cys, Hcy, and GSH when excess of biothiols (5 equiv.) was added to the pyrene-DPA–metal ion complex (Fig. 1). Considering that the pyrene-DPA–Zn2+ complex was previously used as a probe for the detection of PPi, which is abundant in a biological environment, fluorescence changes under interference from PPi were measured (Fig. S2†).32 Unlike the pyrene-DPA–Cd2+ complex, the pyrene-DPA–Zn2+ complex displayed an increase in excimer emission in the presence of PPi. This property of the pyrene-DPA–Zn2+ complex would hinder its application in the detection of H2S in real samples. Therefore, the pyrene-DPA–Cd2+ complex was chosen as an optimal probe for selective and ratiometric H2S detection.
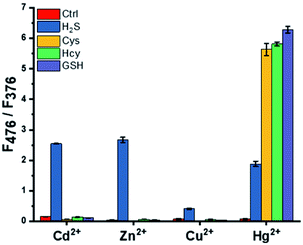 |
| Fig. 1 Fluorescence change (F476/F376) in the excimer at 476 nm against monomer emission at 376 nm of pyrene-DPA–metal ion complex with excess of biothiols (100 μM) in the buffer solution (HEPES, 20 mM, pH 7.4). [pyrene-DPA–metal ion complex] = 20 μM, λex = 341 nm. | |
The optimal pH conditions were determined by evaluating the fluorescence responses of the pyrene-DPA–Cd2+ complex for H2S and Cys because in human serum, the approximate Cys concentration is higher than that of H2S, which makes it difficult to distinguish them (Fig. S3†).33 At pH values above 7.4, interference of Cys was observed, and this interference increased with the pH. A more drastic change in fluorescence induced by Cys was observed at pH 9.0. At neutral pH, the fluorescence enhancement by H2S was still similar to that at basic pH, and interference by Cys was inexistent. As a result, a pH of 7.0 was used in subsequent experiments.
Sensitivity for detection of H2S
The fluorescence change of the pyrene-DPA–Cd2+ complex with various concentrations of H2S was monitored to verify the sensitivity and the available detection range of the developed probe. The excimer emission at 476 nm upon addition of H2S with unchanged monomer emission at 376 nm increased gradually with increasing the H2S concentrations (Fig. 2a). The extent of fluorescence change in the excimer against monomer emission as an internal reference exhibited an almost linear relationship with the concentration of H2S (Fig. 2b). The largest enhancement was observed with 20 μM of H2S because the upper limit of detection was dependent on the concentration (20 μM) of pyrene-DPA–Cd2+ complex (Fig. 2b). As expected, the MDA-based mechanism enables rapid detection and high sensitivity; the fluorescence change was observed within 1 min, and LOD was estimated to be 70 nM from titration data (Fig. S4 and S5†).
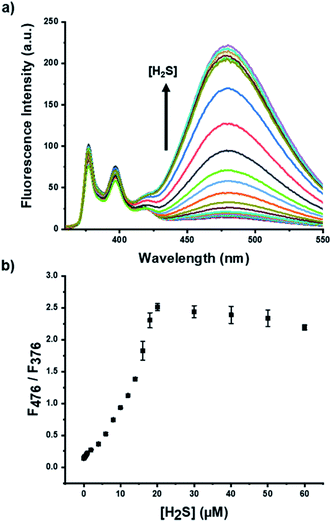 |
| Fig. 2 (a) Fluorescence spectra of pyrene-DPA–Cd2+ complex obtained 1 min after the addition of various concentrations of H2S in the buffer solution (HEPES, 20 mM, pH 7.0); (b) plot of the fluorescence intensity ratio at 476 and 376 nm of pyrene-DPA–Cd2+ complex along with the increased H2S concentrations. [pyrene-DPA–Cd2+ complex] = 20 μM, [H2S] = 0, 0.1, 0.2, 0.4, 0.6, 0.8, 1.0, 2.0, 4.0, 6.0, 8.0, 10.0, 12.0, 14.0, 16.0, 18.0, 20.0, 30.0, 40.0, 50.0, and 60 μM, λex = 341 nm. | |
Selectivity and interference test for detection of H2S
In a biological environment, biothiols and various anions can disturb the detection of H2S. To investigate the selectivity of the suggested probe, spectral changes of the pyrene-DPA–Cd2+ complex in the presence of these compounds were examined. The biothiols, except for H2S, did not affect the fluorescence intensity of the pyrene-DPA–Cd2+ complex, and the entire fluorescence spectra of the complex did not change upon the addition of various anions (Fig. 3a). In particular, the fluorescence responses with biothiols were photographed under irradiation with handheld UV light, and only H2S showed a reliable fluorescence enhancement that can be observed by the naked eye (Fig. 3a). To explore the interference for the probe from biothiols and anions, the detection of H2S in the presence of excess biothiols and various anions (3 equiv.) was performed. The coexistence of other biothiols or most anions did not induce a notable interference in the detection of H2S, while a slight additional increase in excimer emission with PPi was observed (Fig. 3b). Thus, it is expected that this great selectivity and unimpeded features to detect H2S would allow its application in a real serum sample.
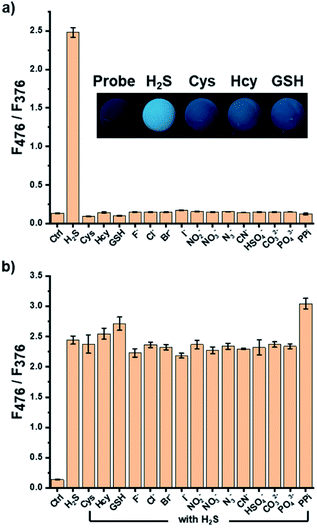 |
| Fig. 3 (a) Fluorescence change in the excimer against monomer emission of pyrene-DPA–Cd2+ complex in the presence of various biothiols and anions (60 μM), and the photograph of the complex with biothiols under irradiation of handheld UV light (365 nm); (b) fluorescence change of the pyrene-DPA–Cd2+ complex by the addition of H2S (20 μM) in the presence of biothiol or anion in the buffer solution (HEPES, 20 mM, pH 7.0). [pyrene-DPA–Cd2+ complex] = 20 μM, λex = 341 nm. | |
Mechanistic study for H2S detection based on metal ion displacement
To evaluate the mechanism for the detection of H2S based on the pyrene-DPA–Cd2+ complex, 1H-NMR spectra along with Cd2+ and subsequent H2S addition to pyrene-DPA were compared (Fig. 4). The assignment of the proton signal in pyrene-DPA was proposed in a previous report.34 The aromatic proton signal (Ha, Hb, Hc, and Hd) in the DPA group, which is a well-known metal ion binding moiety, was shifted downfield following the addition of Cd2+, suggesting that the strong electron-withdrawing Cd2+ was coordinated to the DPA group and reduced the electron shielding effect. In succession, the introduction of H2S in the pyrene-DPA–Cd2+ complex facilitated the recovery of the downfield protons to the initial signal similar to that of pyrene-DPA, regarded as a displacement of Cd2+ through the formation of a CdS complex.35 In addition, the aliphatic proton (He) near the pyrene unit was moved downfield while coordinating with Cd2+ and recovered again with subsequent H2S addition, indicating that effective π–π stacking between pyrene was removed by Cd2+ addition and recovered by H2S addition.36 Thus, it could be inferred that the fluorescence excimer emission recovery after quenching on the coordination of Cd2+ was caused by the change in distance between pyrene groups due to Cd2+ displacement with H2S through the MDA mechanism.
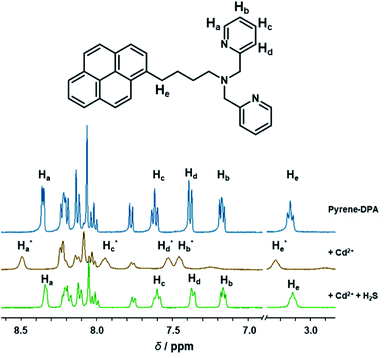 |
| Fig. 4 Change of partial 1H-NMR spectra (400 MHz) of pyrene-DPA–Cd2+ complex (10 mM) in the addition of Cd2+ (10 mM) and subsequent H2S (10 mM) in DMSO-d6 : D2O (8 : 2). | |
Application of H2S detection in human serum samples
The utility of the developed system in a practical sample was confirmed using spiked H2S human serum samples. Human serum samples with various concentrations of spiked H2S were prepared through a pretreatment process to remove proteins. The fluorescence change caused by the spiked H2S was used to estimate the concentrations, and the results were compared with the known values. As summarized in Table 1, the obtained H2S concentration from the probe showed recoveries ranging from 94.8% to 110.1%. Moreover, H2S detection by the demonstrated detection system was unaffected by most molecules present in human serum, and these results show its potential for accurately and precisely detecting H2S in human serum.
Table 1 Determination of H2S in human serum samples
Samples |
Spiked (μM) |
Pyrene-DPA–Cd2+ complex |
Found (μM) |
Recovery (%) |
RSD (%) |
Human serum |
6.0 |
5.7 |
94.8 |
18.5 |
10.0 |
10.9 |
109.3 |
14.4 |
12.0 |
12.6 |
105.0 |
16.9 |
16.0 |
17.1 |
107.1 |
10.0 |
18.0 |
19.8 |
110.1 |
6.4 |
Conclusions
We developed a ratiometric fluorescence probe for selective H2S detection based on the pyrene-DPA–Cd2+ complex, which works based on the MDA mechanism. The increase in excimer emission against a fixed monomer emission, which was used an internal reference, facilitated ratiometric detection of H2S, which was previously difficult using the MDA strategy. Using the MDA, rapid response (within 1 min) and high sensitivity (LOD = 70 nM) for the detection of analytes were confirmed. The selectivity for H2S over Cys, Hcy, and GSH was exhibited without interference from these molecules, and the selectivity over other biothiols was evaluated through the significant fluorescence enhancement monitored by the naked eye upon irradiation with handheld UV light. Moreover, H2S detection in human serum was achieved without interference from other components. Thus, the demonstrated system shows potential for use in practical applications.
Conflicts of interest
There are no conflicts to declare.
Acknowledgements
This work was supported by the National Research Foundation of Korea (NRF) grant funded by the Korea government (MSIT) (NRF-2020R1A2B5B01002392).
References
- D. J. Polhemus and D. J. Lefer, Circ. Res., 2014, 114, 730–737 CrossRef CAS.
- L. Li, P. Rose and P. K. Moore, Annu. Rev. Pharmacol. Toxicol., 2011, 51, 169–187 CrossRef CAS PubMed.
- K. Abe and H. Kimura, J. Neurosci., 1996, 16, 1066–1071 CrossRef CAS PubMed.
- R. Hosoki, N. Matsuki and H. Kimura, Biochem. Biophys. Res. Commun., 1997, 237, 527–531 CrossRef CAS.
- M. Whiteman and P. K. Moore, J. Cell. Mol. Med., 2009, 13, 488–507 CrossRef CAS PubMed.
- M. Lavu, S. Bhushan and D. J. Lefer, Clin. Sci., 2011, 120, 219–229 CrossRef CAS.
- Q. H. Gong, X. R. Shi, Z. Y. Hong, L. L. Pan, X. H. Liu and Y. Z. Zhu, J. Alzheimer's Dis., 2011, 24, 173–182 CAS.
- M. Whiteman and P. G. Winyard, Expert Rev. Clin. Pharmacol., 2011, 4, 13–32 CrossRef CAS PubMed.
- Y.-H. Chen, W.-Z. Yao, J.-Z. Gao, B. Geng, P.-P. Wang and C.-S. Tang, Respirology, 2009, 14, 746–752 CrossRef.
- X. Q. Liu, P. Jiang, H. Huang and Y. Yan, Natl. Med. J. China, 2008, 88, 2246–2249 CAS.
- M. Whiteman, K. M. Gooding, J. L. Whatmore, C. I. Ball, D. Mawson, K. Skinner, J. E. Tooke and A. C. Shore, Diabetologica, 2010, 53, 1722–1726 CrossRef CAS PubMed.
- X. Shen, C. B. Pattillo, S. Pardue, S. C. Bir, R. Wang and C. G. Kevil, Free Radical Biol. Med., 2011, 50, 1021–1031 CrossRef CAS.
- J. E. Doeller, T. S. Isbell, G. Benavides, J. Koenitzer, H. Patel, R. P. Patel, L. J. R. Lancaster, V. M. Darley-Usmar and D. W. Kraus, Anal. Biochem., 2005, 341, 40–51 CrossRef CAS.
- S. Kang, J. Oh and M. S. Han, Dyes Pigm., 2017, 139, 187–192 CrossRef CAS.
- S. Lee, D.-B. Sung, J. S. Lee and M. S. Han, ACS Omega, 2020, 5, 32507–32514 CrossRef CAS PubMed.
- V. S. Lin and C. J. Chang, Curr. Opin. Chem. Biol., 2012, 16, 595–601 CrossRef CAS PubMed.
- H. Li, Y. Fang, J. Yan, X. Ren, C. Zheng, B. Wu, S. Wang, Z. Li, H. Hua, P. Wang and D. Li, TrAC, Trends Anal. Chem., 2021, 134, 116117–116144 CrossRef CAS.
- J. Zhang, Y. Gao, X. Kang, Z. Zhu, Z. Wang, Z. Xi and L. Yi, Org. Biomol. Chem., 2017, 15, 4212–4217 RSC.
- L. Chen, D. Wu, C. S. Lim, D. Kim, S.-J. Nam, W. Lee, G. Kim, H. M. Kim and J. Yoon, Chem. Commun., 2017, 53, 4791–4794 RSC.
- K. Watanabe, T. Suzuki, H. Kitagishi and K. Kano, Chem. Commun., 2015, 51, 4059–4061 RSC.
- L. E. Santos-Figueroa, C. de la Torre, S. El Sayed, F. Sancenon, R. Martinez-Manez, A. M. Costero, S. Gil and M. Parra, Eur. J. Inorg. Chem., 2014, 2014, 41–45 CrossRef CAS.
- R. Kaushik, A. Ghosh and D. milan Jose, Coord. Chem. Rev., 2017, 347, 141–157 CrossRef CAS.
- K. Rurack, M. Kollmannsberger, U. Retsch-Genger and J. Daub, J. Am. Chem. Soc., 2000, 122, 968–969 CrossRef CAS.
- X. Zhao, Y. Zhang, J. Han, H. Jing, Z. Gao, H. Huang, Y. Wang and C. Zhong, Microporous Mesoporous Mater., 2018, 268, 88–92 CrossRef CAS.
- Z. Xu, K. Baek, H. N. Kim, J. Cui, X. Qian, D. R. Spring, I. Shin and J. Yoon, J. Am. Chem. Soc., 2010, 132, 601–610 CrossRef CAS.
- Z. Xu, Y. Xiao, X. Qian, J. Cui and D. Cui, Org. Lett., 2005, 7, 889–892 CrossRef CAS PubMed.
- R. Gui, H. Jin, X. Bu, Y. Fu, Z. Wang and Q. Liu, Coord. Chem. Rev., 2019, 383, 82–103 CrossRef CAS.
- M. H. Lee, J. S. Kim and J. L. Sessler, Chem. Soc. Rev., 2015, 44, 4185–4191 RSC.
- I. Takashima, M. Kinoshita, R. Kawagoe, S. Nakagawa, M. Sugimoto, I. Hamachi and A. Ojida, Chem.–Eur. J., 2014, 20, 2184–2192 CrossRef CAS PubMed.
- R. Kawagoe, I. Takashima, K. Usui, A. Kanegae, Y. Ozawa and A. Ojida, ChemBioChem, 2015, 16, 1608–1615 CrossRef CAS PubMed.
- J. Kim, J. Oh and M. S. Han, RSC Adv., 2021, 11, 10375–10380 RSC.
- H. K. Cho, D. H. Lee and J.-I. Hong, Chem. Commun., 2005, 1690–1692 RSC.
- L. Turell, R. Radi and B. Alvarez, Free Radical Biol. Med., 2013, 65, 244–253 CrossRef CAS.
- Q.-C. Xu, H.-J. Lv, Z.-Q. Lv, M. Liu, Y.-J. Li, X.-F. Wang, Y. Zhang and G.-W. Xing, RSC Adv., 2014, 4, 47788–47792 RSC.
- L. Xue, G. Li, Q. Liu, H. Wang, C. Liu, X. Ding, S. He and H. Jiang, Inorg. Chem., 2011, 50, 3680–3690 CrossRef CAS.
- P. Xing, Z. Zhao, A. Hao and Y. Zhao, Chem. Commun., 2016, 52, 1246–1249 RSC.
Footnotes |
† Electronic supplementary information (ESI) available. See DOI: 10.1039/d1ra04277g |
‡ Both authors contributed equally. |
|
This journal is © The Royal Society of Chemistry 2021 |
Click here to see how this site uses Cookies. View our privacy policy here.