DOI:
10.1039/D1RA04877E
(Paper)
RSC Adv., 2021,
11, 26963-26973
A nano-sized Cu-MOF with high peroxidase-like activity and its potential application in colorimetric detection of H2O2 and glucose†
Received
23rd June 2021
, Accepted 30th July 2021
First published on 9th August 2021
Abstract
Peroxidase widely exists in nature and can be applied for the diagnosis and detection of H2O2, glucose, ascorbic acid and other aspects. However, the natural peroxidase has low stability and its catalytic efficiency is easily affected by external conditions. In this work, a copper-based metal–organic framework (Cu-MOF) was prepared by hydrothermal method, and characterized by means of XRD, SEM, FT-IR and EDS. The synthesized Cu-MOF material showed high peroxidase-like activity and could be utilized to catalyze the oxidation of o-phenylenediamine (OPDA) and 3,3′,5,5′-tetramethylbenzidine (TMB) in the presence of H2O2. The steady-state kinetics experiments of the oxidation of OPDA and TMB catalyzed by Cu-MOF were performed, and the kinetic parameters were obtained by linear least-squares fitting to Lineweaver–Burk plot. The results indicated that the affinity of Cu-MOF towards TMB and OPDA was close to that of the natural horseradish peroxidase (HRP). The as-prepared Cu-MOF can be applied for colorimetric detection of H2O2 and glucose with wide linear ranges of 5 to 300 μM and 50 to 500 μM for H2O2 and glucose, respectively. Furthermore, the specificity of detection of glucose was compared with other sugar species interference such as sucrose, lactose and maltose. In addition, the detection of ascorbic acid and sodium thiosulfate was also performed upon the inhibition of TMB oxidation. Based on the high catalytic activity, affinity and wide linear range, the as-prepared Cu-MOF may be used for artificial enzyme mimics in the fields of catalysis, biosensors, medicines and food industry.
1. Introduction
Metal–organic frameworks (MOFs) are crystalline porous framework materials that are formed with organic ligands and metal ions through coordination bonds with a periodic network structure.1 MOFs have been widely used in catalysis,2 sensing,3 gas adsorption and separation,4 luminescence5 and other fields due to their large specific surface area, unsaturated sites, and structural and functional diversity.6–8 MOFs materials have been shown to have great application prospects with more and more kinds of MOFs and their composite materials having been discovered. In the field of catalysis, MOFs with high catalytic efficiency have been reported. Qin et al. found that hollow mesoporous MOF exhibited superior catalytic performance when loading Pd nanoparticles toward benzyl alcohol oxidation.9 Furthermore, Muhammad Fiaz et al. synthesized high efficient oxygen evolution reaction (OER) catalyst NiS@MOF-5, which can be coated on Ni-foam to form NiS@MOF-5/NF and showed OER catalytic activity and excellent stability.10 Besides, Nguyenet et al. found that Ni-MOF-74 possessed ultrahigh catalytic activity for the arylation of azoles.11
Enzyme, also called biocatalyst, has the characteristics of high efficiency, specificity and mild reaction conditions.12,13 Peroxidase is a kind of enzyme, which is widely existed in nature and can participate in the metabolism of organisms,14 it can also be used for the diagnosis and detection of H2O2, glucose, ascorbic acid.15,16 However, natural enzymes usually have drawbacks of the high cost of preparation and purification, special storage conditions and low stability.15,17–20 The enzyme activity can only be best performed under suitable conditions, such as optimal temperature and acid-alkali conditions.13,21,22 Therefore, people begin to pay attention to the research of artificial mimic peroxidase.23–27
It has been reported that Fe3O4 could catalyze the oxidation of TMB and OPDA to generate blue and orange color reactions, respectively.28 Since then, many researchers have carried out research on the application of Fe3O4 mimic peroxidase in the field of glucose detection.29,30 Later, it has been discovered that Co3O4 nanoparticles,31 V2O5 nanowires,32 CuO nanoparticles,33 MnO2 microspheres34 and Ag2O,21 etc. also have intrinsic catalytic activity on classic peroxidase substrates in the presence of H2O2. At present, colorimetry is the most widely used method for glucose detection. The principle is that glucose oxidase (GOX) catalyzes the oxidation of glucose and the reduction of O2 to H2O2, then the simulated peroxidase catalyzes H2O2 to produce hydroxyl free groups to oxidize substrates (TMB, DAB, OPDA, etc.) to produce color reaction.35
As a peroxidase mimetic enzyme, MOFs have substantive applications in colorimetric detection of some substances such as H2O2 and glucose. It has been reported that some iron-containing MOFs, such as MIL-53(Fe),36 MIL-88(Fe)37 and MIL-68(Fe)38 possess the properties of peroxidase mimics. In the presence of H2O2, the hydrothermally synthesized MIL-53(Fe) can catalyze the oxidation of TMB and OPDA, which has been applied to the detection of actual samples such as glucose and serum with a good linear range and selectivity. Later, it was found that precious metals such as Au and other metals,39 and composite metals (such as bimetallic) also have the catalytic activity of mimetic enzymes,40 which makes MOFs as a popular new material in the simulation of peroxidase.
Several Cu-MOFs were reported previously that different organic compounds were used as coordination ligands, such as uric acid,41 2-aminoterephthalic acid42 and 1,10-phenanthroline-2,9-dicarboxylic acid.43 In this work, we prepared a Cu-MOF by using a simple hydrothermal method with 1,3,5-benzenetricarboxylic acid (H3BTC) as ligand. The peroxidase-like activity of the as-prepared Cu-MOF was investigated by the oxidation reaction of TMB and OPDA with H2O2. The Cu-MOF showed high peroxidase-like activity, which can be used for catalyzing OPDA and TMB to generate colored products in the presence of H2O2. To investigate the possible oxidation mechanism of the Cu-MOF, typical Michaelis–Menten curves were obtained through steady-state kinetic experiments. Furthermore, the color reaction of TMB with H2O2 can be inhibited by some reductive substances. Based on these findings, we obtained satisfied results with the Cu-MOF for colorimetric detection of H2O2, ascorbic acid, sodium thiosulfate and glucose.
2. Experimental
2.1. Materials
1,3,5-Benzenetricarboxylic acid (H3BTC) and TMB were obtained from Shanghai Titan Scientific Co, Ltd (Shanghai, China). OPDA, hydrogen peroxide (H2O2, 30%), copper nitrate trihydrate (Cu(NO3)2·3H2O), sodium acetate (NaAc), acetic acid (HAc), ethanol absolute, phosphate buffered saline (PBS), sodium hydroxide (NaOH), hydrochloric acid (HCl), GOX, glucose, maltose, lactose, sucrose were obtained from Chendu Kelong Chemical Reagent Company. All the reagents were of analytical reagent grade and all the aqueous solutions were prepared with deionized water.
2.2. Characterizations
X-ray powder diffraction (XRD) spectroscopy was performed on Rigaku Dmax/Ultima LV X-ray powder diffractometer. Fourier transform infrared (FT-IR) spectrometer (Nicolet-6700) was applied to record FTIR spectroscopy. Scanning electron microscopy (SEM) image and energy dispersive X-ray spectroscopy (EDS) image were taken by a Hitachi S4800 scanning electron microscope.
2.3. Cu-MOF preparation
The Cu-MOF was prepared by using hydrothermal method. Briefly, 0.45 g (2.14 mmol) H3BTC was dissolved in 48 mL absolute ethanol and stirred for 10 min. Next, addition of 0.75 g (3.1 mmol) of Cu(NO3)2·3H2O to the above solution and continued stirring for 10 min. Then, transferred the mixed solution into a Teflon-lined stainless autoclave. After heated at 120 °C for 12 h and cooled down to the room temperature, collected the Cu-MOF by centrifugation and washed 3 times with absolute ethanol, then dried in a vacuum drying-oven for 24 h at 60 °C to obtain the target product.44
2.4. Peroxidase-like activity
The catalytic oxidation of TMB by H2O2 was performed in 100 mM acetate buffer (pH 4.0) in the presence of Cu-MOF catalyst. Briefly, 2.4 mL of 0.06 mg mL−1 Cu-MOF (Cu-MOF solid was dispersed in acetate buffer by ultrasonication), 300 μL TMB (0.3 mM, dissolve the DMF) and 300 μL H2O2 (0.6 mM) were mixed and reacted for 20 min at 30 °C. The maximum absorption wavelength of TMB oxidized product (652 nm)45 was measured with a UV-Vis spectrophotometer. For OPDA oxidation, 1 mL of 0.06 mg mL−1 Cu-MOF solution (Cu-MOF solid was dispersed in deionized water), 1 mL OPDA (0.4 mM, dissolve the deionized water) and 1 mL H2O2 (0.6 mM) were reacted for 20 min at 30 °C. The maximum absorption wavelength of OPDA oxidized product (416 nm)15 was measured by a UV-Vis spectrophotometer.
2.5. Cu-MOF kinetics measurements
First, 6 mg Cu-MOF solid was dispersed in 100 mL acetate buffer (pH 4.0) to prepare 0.06 mg mL−1 Cu-MOF solution. The concentration of the TMB was fixed at 0.2 mM and a series of H2O2 with different concentrations (10 mM–90 mM) were prepared. During the reaction, 2.6 mL Cu-MOF solution, 0.1 mL H2O2 solution and 0.3 mL TMB were mixed with a total volume of 3 mL at 30 °C. The reaction product was sampled at regular intervals and spectroscopically detected at 652 nm by using a UV-Vis spectrophotometer. Similar experiments were performed by varying the concentration of TMB (0.1 mM–0.9 mM) with fixed concentration of H2O2 (3.5 mM).
For OPDA oxidation, 6 mg Cu-MOF solid was dispersed in 100 mL 0.1 M PBS buffer (pH 7.4) to prepare 0.06 mg mL−1 Cu-MOF solution. The concentration of OPDA was fixed at 0.4 mM with a series of concentration of H2O2 solutions (0.02 mM–0.6 mM). During the reaction, 1 mL Cu-MOF solution, 1 mL H2O2 and 1 mL OPDA were mixed with a total volume of 3 mL at 30 °C. The reaction product was sampled at regular intervals and spectroscopically detected at 416 nm by a UV-vis spectrophotometer. Similar measurements were carried out by varying the concentration of OPDA (0.05 mM–1 mM) with fixed concentration of H2O2 (0.6 mM). By monitoring the absorbance at 652 nm and 416 nm for TMB and OPDA oxidation, the steady-state reaction rates were obtained with the Lambert Beer's law: A = ε × b × C. A is absorbance which can be measured; ε is the molar extinction coefficient of OPDA oxidation product (ε = 16.7 mM−1 cm−1)46 and TMB oxidation product (ε = 39 mM−1 cm−1),47 b is the path length of light (b = 1 cm). The rate V can be calculated by the change of concentration with time: V = Δc/Δt. Michaelis–Menten equation, V = Vmax[S]/(Km + [S]), was used to obtain the apparent kinetic parameters by nonlinear least square fitting the absorbance data. The Vmax is the reaction velocity of an enzyme with saturated substrate. Km is so called Michaelis constant, which represents the substrate concentration when enzymatic reaction velocity V reaches the half of the Vmax. Usually, the Vmax and Km values can be determined by Lineweaver–Burk double reciprocal model (1/V = (Km/Vmax)·(1/[S]) + 1/Vmax). A straight line is formed by plotting 1/V as a function of 1/[S], and the intercept of this line on X axis represents −1/Km, and Y axis represents 1/Vmax.
The experimental procedures of the effects of pH, catalyst concentration and reaction temperature on the peroxidase-like activity of Cu-MOF, and the detection of H2O2, ascorbic acid, sodium thiosulfate and glucose were similar to the above-mentioned experimental process (see ESI† for details). The data presented in this work were the averages of at least two measurements with an error less than 5% unless otherwise stated.
3. Results and discussions
3.1. Characterizations
The XRD pattern of the Cu-MOF is shown in Fig. 1. The characteristic peaks at 2θ = 6.7°, 9.5°, 11.6°, 13.4°, 14.9°, 17.5°, 19.1°, 25.9° and 39.1° correspond to the (200), (220), (222), (400), (420), (511), (440), (731) and (882) crystal planes.48–50 The narrow and sharp peaks and the main peaks are coincided well with the simulated peaks, which prove that the synthesized material is the target product. The SEM image shows that the Cu-MOF sample is mainly composed of polyhedron, and some irregular particles can also be seen on the surface of the sample (Fig. 2). The FTIR spectrum of Cu-MOF is shown in Fig. S1,† the peaks at 1370 cm−1 can be attributed to the aromatic ring extension. 690–900 cm −1 were assigned to the out-of-plane bending vibration of aromatic hydrocarbons C–H.51 The absorption bands at 600–800 cm−1 are due to lattice vibrations of Cu–O, Cu–O–Cu and O–Cu–O. The stretching vibration of benzene ring is at 1579 cm−1, whereas the peak at 3404 cm−1 can be ascribed to water molecules and hydroxyl groups on the surface of the sample.51–55 The energy dispersive spectrum (EDS) and element mapping indicate that Cu, O and C elements are coexisted and distributed evenly in the sample (Fig. S2†).
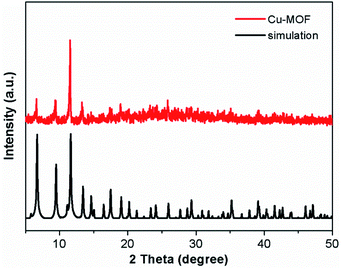 |
| Fig. 1 The XRD pattern of Cu-MOF. | |
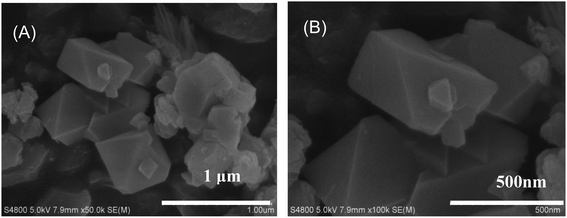 |
| Fig. 2 The SEM images of Cu-MOF with different magnifications. | |
3.2. Peroxidase activity
The peroxidase-like activity of the Cu-MOF was evaluated by the catalytic reaction of OPDA and TMB with H2O2. Fig. 3A shows that the Cu-MOF can catalyze H2O2 to oxidize OPDA at 30 °C, which produce a color product since the solution become yellow after the reaction (Fig. 3A, inset). The oxidation product of OPDA shows an absorption band at wavelength of 416 nm in the visible region (Fig. 3A). Besides, the Cu-MOF can also catalyze the oxidation of TMB by H2O2, and the oxidation product of TMB exhibited a broad absorption band at 652 nm in the visible region and a peak at 371 nm in the UV region (Fig. 3B). The color of the TMB solution changed to blue after oxidation by H2O2 (Fig. 3B, inset). The absorption peaks at 416 nm and 652 nm for OPDA and TMB solutions, respectively, were not observed in the UV-Vis spectra without addition of Cu-MOF (Fig. 3A and B), and the corresponding solutions were still colorless (the little glass bottles on the right of the insets in Fig. 3A and B, respectively). These results proved that the colored products generated by the reaction of OPDA and TMB with H2O2 were indeed catalyzed by the Cu-MOF material.
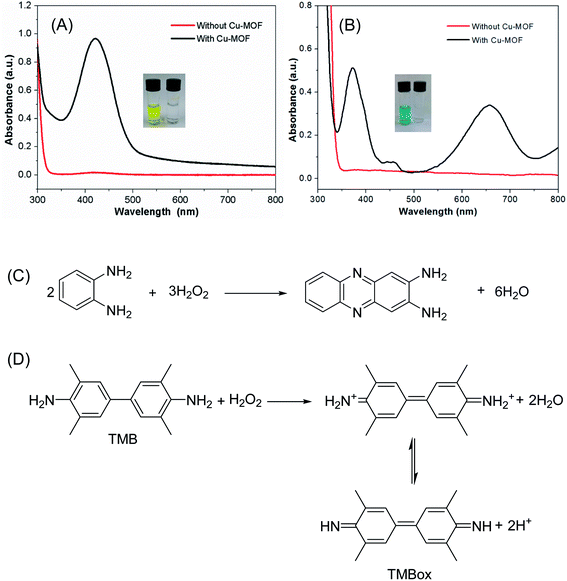 |
| Fig. 3 The UV-Vis spectra of OPDA (A) and TMB (B) (insets: photographs of OPDA and TMB oxidized with H2O2) with and without Cu-MOF material. (C and D) Are the corresponding reaction equations for Cu-MOF catalyzed OPDA and TMB, respectively. | |
Fig. 3C and D show the corresponding reaction equations for Cu-MOF catalyzed OPDA and TMB oxidation, respectively. In the presence of Cu-MOF as catalysts, OPDA and TMB are oxidized by H2O2, producing a yellow-colored dimer product (2,3-diaminophenazine) (Fig. 3C) and a blue oxidized product complex (Fig. 3D), and H2O2 is reduced to H2O simultaneously. The 371 and 652 nm bands in the absorption spectrum of TMB oxidation product are a charge transfer complex consisting of the diamine (TMB) as a donor and the diimine dication (TMB2+) as an acceptor.56,57
3.3. Experimental condition optimization
Natural enzymes are easily inactivated under extreme conditions such as strong acid, strong base and high temperature. Herein, the effect of pH, temperature, and catalyst concentration were investigated for the peroxidase activity. First, we explored the effect of pH. For the OPDA, the reaction activity increased from pH 3.0 to pH 4.5, while decreased with further pH increase up to 9.0 (Fig. 4A), which similar to that of the peroxidase activity of HRP effected by pH. While for the TMB, the reaction activity increased from pH 3.0 to pH 4.0, while decreased with further increasing of the pH (Fig. 4B). We further investigated the effect of temperature on the peroxidase-like activity of Cu-MOF. It can be seen that the catalytic activity of Cu-MOF increased from 25 °C to 70 °C when oxidation of OPDA with H2O2 (Fig. 4C) and the activity of the enzyme was not lose even up to 70 °C. While the highest activity was at 30 °C for TMB oxidation, and the color of the oxidation product faded marvelously at 45 °C (Fig. 4D), which indicated that the enzyme had been inactivated. Finally, we studied the catalyst concentration effect and the results are shown in the Fig. 4E and F. The activity of OPDA oxidation increased linearly with the increase of catalyst between 0.03 and 0.06 mg mL−1, which reached maximum at 0.06 mg mL−1 and then tends to be flat. However, the concentration of catalyst had little effect on the activity for the oxidation of TMB with the activity higher than 70% between 0.03 and 0.09 mg mL−1.
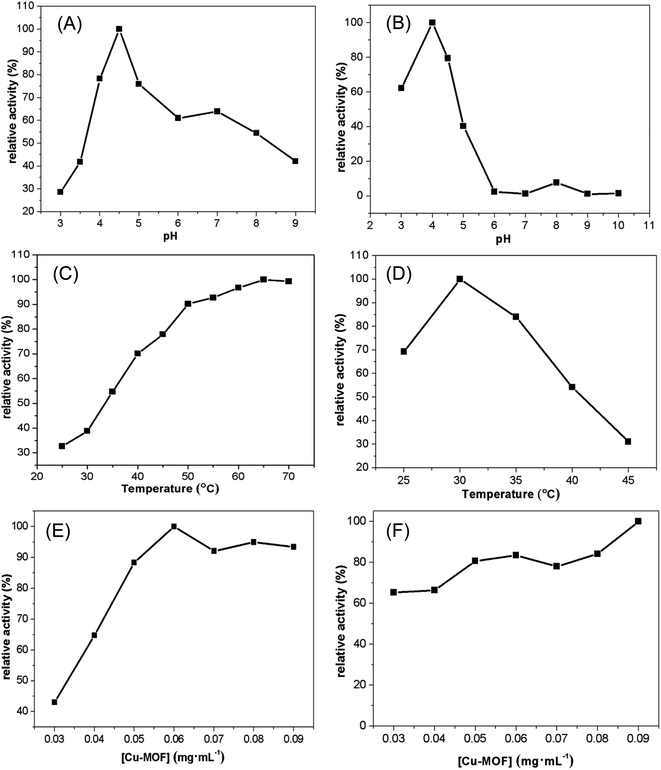 |
| Fig. 4 The effect of pH for oxidation of OPDA (A) and TMB (B), temperature effect for oxidation of OPDA (C) and TMB (D), and the effect of catalyst concentration for oxidation of OPDA (E) and TMB (F). | |
3.4. Steady-state kinetics study
Steady-state kinetics assays were carried out to explore the mechanism of the peroxidase-like activity of the synthesized Cu-MOF. The kinetic data were measured by fixing concentration of H2O2 and varying the OPDA (or TMB) concentration or vice versa with Cu-MOF. The formation rates of the OPDA and TMB oxidized products were monitored from the increasing of the absorbance at 416 nm and 652 nm, respectively. The Fig. 5A showed the reaction rate of Cu-MOF peroxidase gradually increased with the increase of H2O2 when fixing OPDA. While when the H2O2 increases to a certain extent, the increasing rate of the reaction rate becomes slower and gradually flattens out. Secondly, when the concentration of H2O2 is fixed, the reaction rate of Cu-MOF peroxidase gradually increases with the increase of OPDA (Fig. 5B). However, when the concentration of OPDA increases to a certain extent, the reaction rate also gradually slows down. The fitting parameters (Km and Vmax) could be obtained through hyperbola curve fitting, which were shown in the Fig. 5C and D.
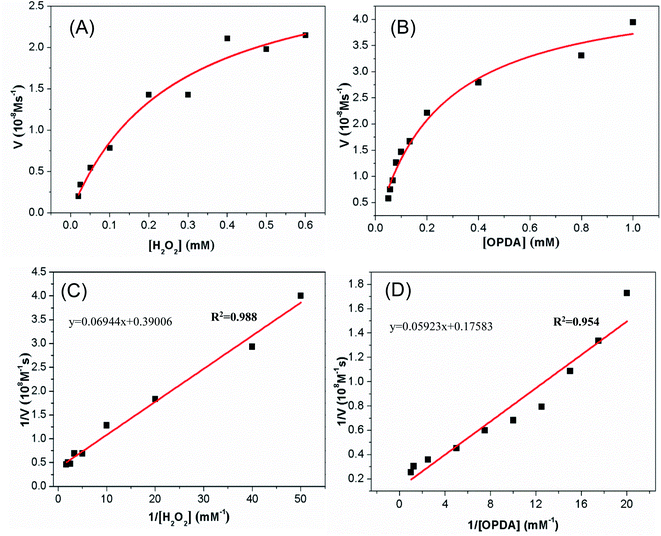 |
| Fig. 5 Steady-state kinetics for the reaction of OPDA with H2O2 in the present of Cu-MOF. (A and C) Fixed OPDA concentration (0.4 mM) and varied H2O2 concentration (0.02 mM–0.6 mM); (B and D) fixed H2O2 concentration (0.6 mM) and varied OPDA concentration (0.05 mM–1 mM). The curves in panels A and B were obtained by nonlinear least square fitting to Michaelis–Menten equation, V = Vmax·[S]/(Km + [S]), and the lines in panels C and D were obtained by linear least square fitting to Lineweaver–Burk double reciprocal model, 1/V = (Km/Vmax)·(1/[S]) + 1/Vmax. | |
The steady-state kinetics of TMB oxidation by H2O2 catalyzed with Cu-MOF is shown in Fig. 6A. The reaction rate of TMB oxidation gradually increased with the increasing of H2O2 when fixing TMB. While when the H2O2 increases to a certain extent, the increasing of the reaction rate becomes slower and gradually flattens out. Secondly, when the concentration of H2O2 is fixed, the reaction rate of Cu-MOF peroxidase gradually increases with the increase of TMB (Fig. 6B). However, when the concentration of TMB increases to a certain extent, the reaction rate also gradually slows down. By using the Lineweave–Burk double-reciprocal model to fit the data in the Michaelis curve, the straight lines can be obtained as shown in the Fig. 6C and D.
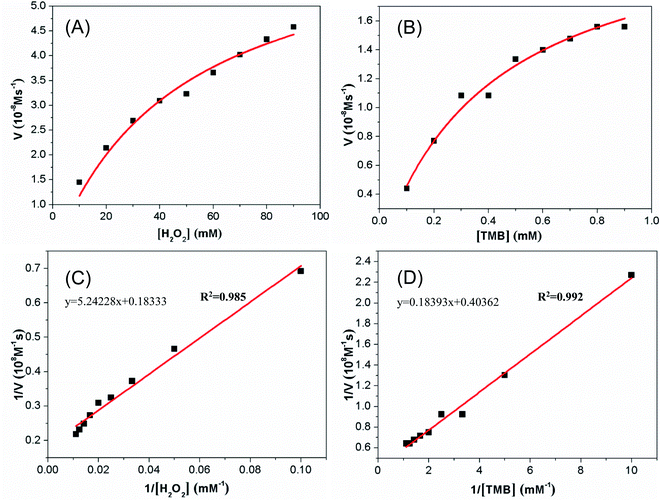 |
| Fig. 6 Steady-state kinetics for the reaction of TMB with H2O2 in the present of Cu-MOF. (A and C) Fixed TMB concentration (0.2 mM) and varied H2O2 concentration (10 mM–90 mM); (B and D) fixed H2O2 concentration (3.5 mM) and varied TMB concentration (0.1 mM–0.9 mM). The curves in panels A and B and the lines in panels C and D were obtained as stated in Fig. 5. | |
The kinetic parameters Km and Vmax for the catalyst of Cu-MOF were calculated through the intercept and slope in the straight line. Tables S1 and S2† list the Km and Vmax values of several catalysts for substrates OPDA, TMB and H2O2. Km value is one of the characteristic constant of enzymes, which indicates the affinity between enzyme and substrate. The higher the Km value, the smaller the affinity between enzyme and substrate. These results showed that the Km values of the Cu-MOF for OPDA and TMB were 0.54 mM and 0.456 mM, respectively. And the Km values of natural HRP for OPDA and TMB were 0.59 mM and 0.434 mM, respectively.28,58 Therefore, the affinity of Cu-MOF was similar to that of natural HRP, indicating it can be used as an artificial peroxidase. Through further comparison, we found that the affinity of Cu-MOF for OPDA and TMB is better than some of the published peroxidase mimetic materials, such as the affinity of Fe3O4@Cu@Cu2O to OPDA and the affinity of Cu NCs or MoO2 to TMB (Tables S1 and S2†).
3.5. Colorimetric detection of H2O2, ascorbic acid and sodium thiosulfate
The oxidation of TMB by H2O2 via the catalysis of Cu-MOF to generate a blue product, and the reaction rate was proportional to the concentration of H2O2. Based on this principle, a colorimetric method to detect H2O2 was established. As shown in Fig. 7A, the absorbance at 652 nm increased with the concentration increasing of H2O2 from 5 μM to 400 μM. A well linear relationship can be achieved between the absorbance intensity and H2O2 concentration from 5 μM to 300 μM (Fig. 7B). The detection limit was 4.6 μM and the correlation coefficient was 0.997. The detection limit was determined by LOD = KS0/S. K is the numerical factor chosen on the basis of the confidence level desired. S0 is the standard deviation59 of the blank measurements (n = 11, K = 3), while S is the slope of the calibration curve. Table S3† list several peroxidase mimics for colorimetric detection of H2O2. According to the Table S3,† the linear range of the as-prepared Cu-MOF is wider than some of the published peroxidase mimics such as CuS-GNS,60 and the detect limitation is also lower than some reported peroxidase mimics such as MnO2,58 indicated that Cu-MOF is suitable for the colorimetric detection of H2O2.
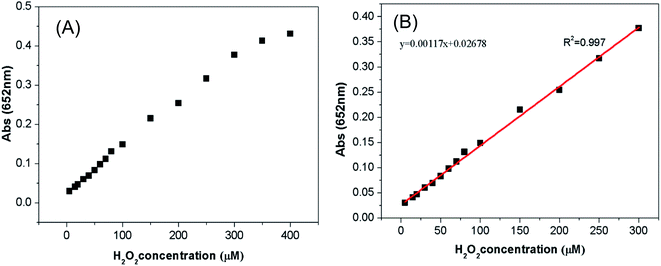 |
| Fig. 7 Colorimetric detection of H2O2. Dose–response curve (A) and linear calibration plot (B) for the detection of H2O2 with Cu-MOF. | |
Moreover, it was found that the color reaction of TMB with H2O2 could be inhibited by ascorbic acid (AA) and sodium thiosulfate (Na2S2O3). Accordingly, a colorimetric detection method of AA and Na2S2O3 was also developed based on the color reaction inhibition (ESI, Fig. S3 and S4†). It should be pointed out that the detection of AA and Na2S2O3 is not specific, for other reductive substance such as Na2SO3 can also inhibit the color reaction of TMB with H2O2.21,61
3.6. Glucose detection
GOX is a typical oxidoreductase that can be used to catalyze the oxidation of glucose to produce glucono-δ-lactone and H2O2.62 It can be used in clinical diagnosis, rapid and accurate determination of glucose content in body fluid, which provides reliable data for doctors to accurately judge the patient's condition.37,63,64 As shown in the Fig. 8A and B, the absorbance at 652 nm for TMB oxidation increased with the glucose concentration from 5 μM to 500 μM. A well linear relationship can be obtained between the intensity of absorbance and the glucose concentration from 5 μM to 500 μM. The detection limit is 4.7 μM and the correlation coefficient is 0.993.
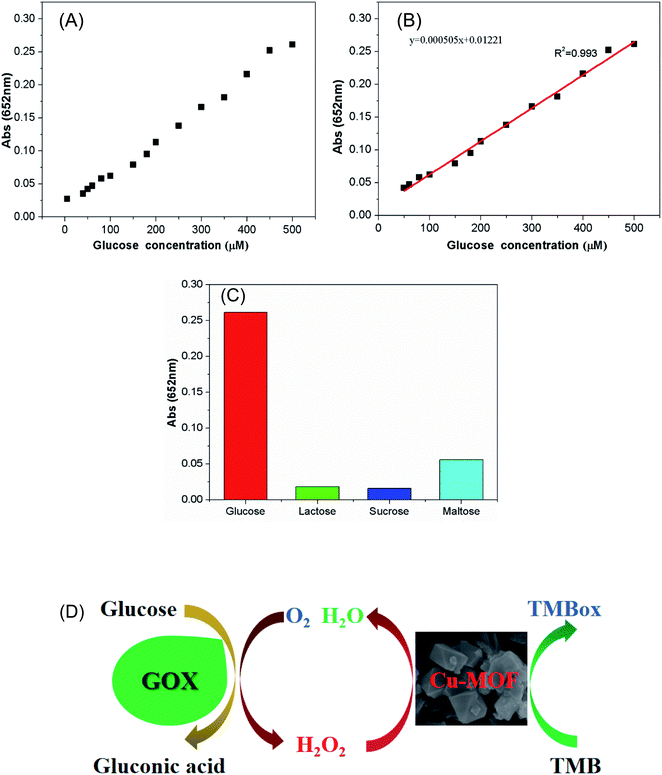 |
| Fig. 8 The colorimetric detection of glucose. Dose–response curve (A) and linear calibration plot (B) for glucose detection with Cu-MOF. (C) Determination of the selectivity detection for glucose with 300 μM glucose, 2 mM sucrose, 2 mM lactose, and 2 mM maltose. (D) A proposed reaction mechanism for detection of glucose. | |
To explore the selectivity of glucose detection, we performed the colorimetric reaction by replacing glucose with other sugar species, such as sucrose, lactose or maltose. The results show that the absorbance increase at 652 nm is very low for the selected sugar species instead of glucose (Fig. 8C). Therefore, the developed colorimetric detection method has high selectivity for the detection of glucose.
The reaction mechanism for glucose detection is illustrated in Fig. 8D. First, GOX catalyzed the oxidation of glucose to gluconic acid and O2 dissolved in the solution was reduced to H2O2. Then the produced H2O2 oxidized TMB to a blue-colored oxidation product (TMBox) via the catalysis of Cu-MOF. The production of TMBox is proportional to the amount of H2O2, and the yield of H2O2 is proportional to the amount of glucose. Therefore, the production of TMBox is proportional to the amount of glucose and glucose can be detected by measuring the amount of TMBox. It also can be seen from the reaction mechanism that the detection of glucose is achieved by measuring the content of H2O2, thus the selectivity of glucose detection depends on the selectivity of GOX to glucose, but not on the catalysis of Cu-MOF.
Table S4† listed several peroxidase mimic catalysts for the colorimetric detection of glucose. According to the Table S4,† the linear range of the as-prepared Cu-MOF is wider than that of NiFe2O4 MNPs and Ch-Ag NPs.65,66 The detection limit is relatively large, which indicated that Cu-MOF has better linear range and detection limit to detect glucose. These results demonstrated that this colorimetric method may has potential application for determine glucose concentration.
4. Conclusion
In conclusion, we prepared Cu-MOF with high peroxidase-like activity by a simple hydrothermal method. In the presence of H2O2, Cu-MOF can catalyze the oxidation of OPDA and TMB. Kinetics studies suggested that the affinity of Cu-MOF to TMB and OPDA is close to that of HRP. Based on the color reaction of TMB with H2O2, colorimetric methods for the detection of H2O2 and glucose were established. Moreover, the detection of ascorbic acid and sodium thiosulfate was also performed upon the inhibition of TMB oxidation. These results indicate that Cu-MOF based peroxidase-like activity may be applied in the fields of catalysis, biosensor, food detection and environmental monitoring.
Conflicts of interest
There are no conflicts to declare.
Acknowledgements
This study was funded by the Open Project of Chemical Synthesis and Pollution Control Key Laboratory of Sichuan Province (CSPC2016-3-2), and the Opening Project of Key Laboratory of Green Chemistry of Sichuan Institutes of Higher Education (LZJ2002).
References
- D. Li, S. Zhang, X. Feng, H. Yang, F. Nie and W. Zhang, A novel peroxidase mimetic Co-MOF enhanced luminol chemiluminescence and its application in glucose sensing, Sens. Actuators, B, 2019, 296, 126631 CrossRef CAS.
- K. Shen, X. Chen, J. Chen and Y. Li, Development of MOF-derived carbon-based nanomaterials for efficient catalysis, ACS Catal., 2016, 6, 5887–5903 CrossRef CAS.
- L. E. Kreno, K. Leong, O. K. Farha, M. Allendorf, R. P. Van Duyne and J. T. Hupp, Metal–organic framework materials as chemical sensors, Chem. Rev., 2012, 112, 1105–1125 CrossRef CAS.
- D. Saha, H. A. Grappe, A. Chakraborty and G. Orkoulas, Postextraction separation, on-board storage, and catalytic conversion of methane in natural gas: a review, Chem. Rev., 2016, 116, 11436–11499 CrossRef CAS.
- D. Cunha, M. Ben Yahia, S. Hall, S. R. Miller, H. Chevreau, E. Elkaïm, G. Maurin, P. Horcajada and C. Serre, Rationale of drug encapsulation and release from biocompatible porous metal–organic frameworks, Chem. Mater., 2013, 25, 2767–2776 CrossRef CAS.
- G. Maurin, C. Serre, A. Cooper and G. Férey, The new age of MOFs and of their porous-related solids, Chem. Soc. Rev., 2017, 46, 3104–3107 RSC.
- L. Chen, R. Luque and Y. Li, Controllable design of tunable nanostructures inside metal–organic frameworks, Chem. Soc. Rev., 2017, 46, 4614–4630 RSC.
- D. Feng, T.-F. Liu, J. Su, M. Bosch, Z. Wei, W. Wan, D. Yuan, Y.-P. Chen, X. Wang and K. Wang, Stable metal-organic frameworks containing single-molecule traps for enzyme encapsulation, Nat. Commun., 2015, 6, 1–8 Search PubMed.
- Y. Qin, X. Han, Y. Li, A. Han, W. Liu, H. Xu and J. Liu, Hollow Mesoporous Metal–Organic Frameworks with Enhanced Diffusion for Highly Efficient Catalysis, ACS Catal., 2020, 10, 5973–5978 CrossRef CAS.
- M. Fiaz, M. Kashif, M. Fatima, S. R. Batool, M. A. Asghar, M. Shakeel and M. Athar, Synthesis of Efficient TMS@MOF-5 Catalysts for Oxygen Evolution Reaction, Catal. Lett., 2020, 150, 2648–2659 CrossRef CAS.
- H. T. Nguyen, D. N. Doan and T. Truong, Unprecedented salt-promoted direct arylation of acidic sp2 CH bonds under heterogeneous Ni-MOF-74 catalysis: synthesis of bioactive azole derivatives, J. Mol. Catal. A: Chem., 2017, 426, 141–149 CrossRef CAS.
- S. K. Maji, A. K. Dutta, S. Dutta, D. N. Srivastava, P. Paul, A. Mondal and B. Adhikary, Single-source precursor approach for the preparation of CdS nanoparticles and their photocatalytic and intrinsic peroxidase like activity, Appl. Catal., B, 2012, 126, 265–274 CrossRef CAS.
- P. V. Iyer and L. Ananthanarayan, Enzyme stability and stabilization—aqueous and non-aqueous environment, Process Biochem., 2008, 43, 1019–1032 CrossRef CAS.
- M. Yuan, H. Zhao, Q. Huang, X. Liu, Y. Zhou, X. Diao and Q. X. Li, Comparison of three palm tree peroxidases expressed by Escherichia coli: Uniqueness of African oil palm peroxidase, Protein Expression Purif., 2020, 179, 105806 CrossRef PubMed.
- Z. Wang, M. Chen, J. Shu and Y. Li, One-step solvothermal synthesis of Fe3O4@Cu@Cu2O nanocomposite as magnetically recyclable mimetic peroxidase, J. Alloys Compd., 2016, 682, 432–440 CrossRef CAS.
- Q. Wang, S. Liu, H. Sun and Q. Lu, Synthesis and Intrinsic Peroxidase-Like Activity of Sisal-Like Cobalt Oxide Architectures, Ind. Eng. Chem. Res., 2014, 53, 7917–7922 CrossRef CAS.
- Y. Chen, H. Cao, W. Shi, H. Liu and Y. Huang, Fe–Co bimetallic alloy nanoparticles as a highly active peroxidase mimetic and its application in biosensing, Chem. Commun., 2013, 49, 5013–5015 RSC.
- M. Chen, Z. H. Wang, J. X. Shu, X. H. Jiang, W. Wang, Z. H. Shi and Y. W. Lin, Mimicking a Natural Enzyme System: Cytochrome c Oxidase-Like Activity of Cu2O Nanoparticles by Receiving Electrons from Cytochrome c, Inorg. Chem., 2017, 56, 9400–9403 CrossRef CAS PubMed.
- Y. W. Lin, Rational design of heme enzymes for biodegradation of pollutants toward a green future, Biotechnol. Appl. Biochem., 2020, 67, 484–494 CrossRef CAS PubMed.
- Y.-l. Dong, H.-g. Zhang, Z. U. Rahman, L. Su, X.-j. Chen, J. Hu and X.-g. Chen, Graphene oxide–Fe 3 O 4 magnetic nanocomposites with peroxidase-like activity for colorimetric detection of glucose, Nanoscale, 2012, 4, 3969–3976 RSC.
- W. Lu, J. Shu, Z. Wang, N. Huang and W. Song, The intrinsic oxidase-like activity of Ag2O nanoparticles and its application for colorimetric detection of sulfite, Mater. Lett., 2015, 154, 33–36 CrossRef CAS.
- M. Chen, Z. Wang, J. Shu, X. Jiang, W. Wang, Z.-H. Shi and Y.-W. Lin, Mimicking a natural enzyme system: cytochrome c oxidase-like activity of Cu2O nanoparticles by receiving electrons from cytochrome c, Inorg. Chem., 2017, 56, 9400–9403 CrossRef CAS.
- S. Maeno, Y. Mizutani, Q. Zhu, T. Miyamoto, M. Fukushima and H. Kuramitz, The oxidation of tetrabromobisphenol A by potassium monopersulfate with an iron(III)-phthalocyanine-tetrasulfonic acid catalyst in the presence of humic acid, J. Environ. Sci. Health, Part A: Toxic/Hazard. Subst. Environ. Eng., 2014, 49, 981–987 CrossRef CAS PubMed.
- T. Ying, F. Zhong, Z. H. Wang, J. Xie, X. Tan and Z. X. Huang, Generation of novel functional metalloproteins via hybrids of cytochrome c and peroxidase, Protein Eng., Des. Sel., 2013, 26, 401–407 CrossRef CAS PubMed.
- I. Artaud, K. Ben-Aziza and D. Mansuy, Iron porphyrin-catalyzed oxidation of 1, 2-dimethoxyarenes: a discussion of the different reactions involved and the competition between the formation of methoxyquinones or muconic dimethyl esters, J. Org. Chem., 1993, 58, 3373–3380 CrossRef CAS.
- C. Liu, J. K. Xu, S. Q. Gao, B. He, C. W. Wei, X. J. Wang, Z. H. Wang and Y. W. Lin, Green and efficient biosynthesis of indigo from indole by engineered myoglobins, RSC Adv., 2018, 8, 33325–33330 RSC.
- J. J. X. Wu, X. Y. Wang, Q. Wang, Z. P. Lou, S. R. Li, Y. Y. Zhu, L. Qin and H. Wei, Nanomaterials with enzyme-like characteristics (nanozymes): next-generation artificial enzymes (II), Chem. Soc. Rev., 2019, 48, 1004–1076 RSC.
- L. Gao, J. Zhuang, L. Nie, J. Zhang, Y. Zhang, N. Gu, T. Wang, J. Feng, D. Yang, S. Perrett and X. Yan, Intrinsic peroxidase-like activity of ferromagnetic nanoparticles, Nat. Nanotechnol., 2007, 2, 577–583 CrossRef CAS PubMed.
- S. Song, Y. Liu, A. Song, Z. Zhao, H. Lu and J. Hao, Peroxidase mimetic activity of Fe3O4 nanoparticle prepared based on magnetic hydrogels for hydrogen peroxide and glucose detection, J. Colloid Interface Sci., 2017, 506, 46–57 CrossRef CAS PubMed.
- Y. Shi, J. Huang, J. Wang, P. Su and Y. Yang, A magnetic nanoscale Fe3O4/Pβ-CD composite as an efficient peroxidase mimetic for glucose detection, Talanta, 2015, 143, 457–463 CrossRef CAS.
- J. Mu, Y. Wang, M. Zhao and L. Zhang, Intrinsic peroxidase-like activity and
catalase-like activity of Co3O4 nanoparticles, Chem. Commun., 2012, 48, 2540–2542 RSC.
- R. André, F. Natálio, M. Humanes, J. Leppin, K. Heinze, R. Wever, H. C. Schröder, W. E. G. Müller and W. Tremel, V2O5 Nanowires with an Intrinsic Peroxidase-Like Activity, Adv. Funct. Mater., 2011, 21, 501–509 CrossRef.
- W. Chen, J. Chen, Y. B. Feng, L. Hong, Q. Y. Chen, L. F. Wu, X. H. Lin and X. H. Xia, Peroxidase-like activity of water-soluble cupric oxide nanoparticles and its analytical application for detection of hydrogen peroxide and glucose, Analyst, 2012, 137, 1706–1712 RSC.
- M. Chen, J. Shu, Z. Wang and C. Ren, Porous surface MnO2 microspheres as oxidase mimetics for colorimetric detection of sulfite, J. Porous Mater., 2016, 24, 973–977 CrossRef.
- F. Qiao, L. Chen, X. Li, L. Li and S. Ai, Peroxidase-like activity of manganese selenide nanoparticles and its analytical application for visual detection of hydrogen peroxide and glucose, Sens. Actuators, B, 2014, 193, 255–262 CrossRef CAS.
- L. Ai, L. Li, C. Zhang, J. Fu and J. Jiang, MIL-53(Fe): a metal-organic framework with intrinsic peroxidase-like catalytic activity for colorimetric biosensing, Chem.–Eur. J, 2013, 19, 15105–15108 CrossRef CAS.
- Y. L. Liu, X. J. Zhao, X. X. Yang and Y. F. Li, A nanosized metal-organic framework of Fe-MIL-88NH(2) as a novel peroxidase mimic used for colorimetric detection of glucose, Analyst, 2013, 138, 4526–4531 RSC.
- F. Jing, R. Liang, J. Xiong, R. Chen, S. Zhang, Y. Li and L. Wu, MIL-68(Fe) as an efficient visible-light-driven photocatalyst for the treatment of a simulated waste-water contain Cr(VI) and Malachite Green, Appl. Catal., B, 2017, 206, 9–15 CrossRef CAS.
- Y. Jv, B. Li and R. Cao, Positively-charged gold nanoparticles as peroxidase mimic and their application in hydrogen peroxide and glucose detection, Chem. Commun., 2010, 46, 8017–8019 RSC.
- H. Yang, R. Yang, P. Zhang, Y. Qin, T. Chen and F. Ye, A bimetallic (Co/2Fe) metal-organic framework with oxidase and peroxidase mimicking activity for colorimetric detection of hydrogen peroxide, Microchim. Acta, 2017, 184, 4629–4635 CrossRef CAS.
- F. Liu, J. He, M. Zeng, J. Hao, Q. Guo, Y. Song and L. Wang, Cu–hemin metal-organic frameworks with peroxidase-like activity as peroxidase mimics for colorimetric sensing of glucose, J. Nanopart. Res., 2016, 18, 106 CrossRef.
- S. Wang, W. Deng, L. Yang, Y. Tan, Q. Xie and S. Yao, Copper-based metal–organic framework nanoparticles with peroxidase-like activity for sensitive colorimetric detection of Staphylococcus aureus, ACS Appl. Mater. Interfaces, 2017, 9, 24440–24445 CrossRef CAS.
- J. Wang, Y. Hu, Q. Zhou, L. Hu, W. Fu and Y. Wang, Peroxidase-like Activity of Metal-Organic Framework [Cu(PDA)(DMF)] and Its Application for Colorimetric Detection of Dopamine, ACS Appl. Mater. Interfaces, 2019, 11, 44466–44473 CrossRef CAS.
- W. Xuan, R. Ramachandran, C. Zhao and F. Wang, Influence of synthesis temperature on cobalt metal-organic framework (Co-MOF) formation and its electrochemical performance towards supercapacitor electrodes, J. Solid State Electrochem., 2018, 22, 3873–3881 CrossRef CAS.
- W. Dong, Y. Zhuang, S. Li, X. Zhang, H. Chai and Y. Huang, High peroxidase-like activity of metallic cobalt nanoparticles encapsulated in metal–organic frameworks derived carbon for biosensing, Sens. Actuators, B, 2018, 255, 2050–2057 CrossRef CAS.
- S. Fornera and P. Walde, Spectrophotometric quantification of horseradish peroxidase with o-phenylenediamine, Anal. Biochem., 2010, 407, 293–295 CrossRef CAS.
- P. D. Josephy, T. Eling and R. P. Mason, The horseradish peroxidase-catalyzed oxidation of 3, 5, 3', 5'-tetramethylbenzidine. Free radical and charge-transfer complex intermediates, J. Biol. Chem., 1982, 257, 3669–3675 CrossRef CAS.
- R. Kaur, A. Kaur, A. Umar, W. A. Anderson and S. K. Kansal, Metal organic framework (MOF) porous octahedral nanocrystals of Cu-BTC: Synthesis, properties and enhanced adsorption properties, Mater. Res. Bull., 2019, 109, 124–133 CrossRef CAS.
- J. Zhou, D. Liu, Y. Xiong and Y. Akinay, A novel approach to prepare polyaniline/Polypyrrole@Cu-BTC/NH2-MIL-101(Fe) MOFs for electromagnetic wave absorption, Ceram. Int., 2020, 46, 19758–19766 CrossRef CAS.
- Y.-F. Zhang, L.-G. Qiu, Y.-P. Yuan, Y.-J. Zhu, X. Jiang and J.-D. Xiao, Magnetic Fe3O4@C/Cu and Fe3O4@CuO core–shell composites constructed from MOF-based materials and their photocatalytic properties under visible light, Appl. Catal., B, 2014, 144, 863–869 CrossRef CAS.
- Q. Zhao, L. Zhu, G. Lin, G. Chen, B. Liu, L. Zhang, T. Duan and J. Lei, Controllable Synthesis of Porous Cu-BTC@polymer Composite Beads for Iodine Capture, ACS Appl. Mater. Interfaces, 2019, 11, 42635–42645 CrossRef CAS PubMed.
- R. M. Abdelhameed, H. E. Emam, J. Rocha and A. M. S. Silva, Cu-BTC metal-organic framework natural fabric composites for fuel purification, Fuel Process. Technol., 2017, 159, 306–312 CrossRef CAS.
- V. Jabbari, J. M. Veleta, M. Zarei-Chaleshtori, J. Gardea-Torresdey and D. Villagrán, Green synthesis of magnetic MOF@GO and MOF@CNT hybrid nanocomposites with high adsorption capacity towards organic pollutants, Chem. Eng. J., 2016, 304, 774–783 CrossRef CAS.
- R. M. Abdelhameed, H. Abdel-Gawad, M. Elshahat and H. E. Emam, Cu–BTC@cotton composite: design and removal of ethion insecticide from water, RSC Adv., 2016, 6, 42324–42333 RSC.
- T. J. Bandosz and C. Petit, MOF/graphite oxide hybrid materials: exploring the new concept of adsorbents and catalysts, Adsorption, 2010, 17, 5–16 CrossRef.
- Y. Misono, Y. Ohkata, T. Morikawa and K. Itoh, Resonance Raman and absorption spectroscopic studies on the electrochemical oxidation processes of 3, 3′, 5, 5′-tetramethylbenzidine, J. Electroanal. Chem., 1997, 436, 203–212 CrossRef CAS.
- X. Zhang, Q. Yang, Y. Lang, X. Jiang and P. Wu, Rationale of 3, 3′, 5, 5′-tetramethylbenzidine as the chromogenic substrate in colorimetric analysis, Anal. Chem., 2020, 92, 12400–12406 CrossRef CAS PubMed.
- X. Liu, Q. Wang, H. Zhao, L. Zhang, Y. Su and Y. Lv, BSA-templated MnO2 nanoparticles as both peroxidase and oxidase mimics, Analyst, 2012, 137, 4552–4558 RSC.
- Y. Li, R. Cui, H. Huang, J. Dong, B. Liu, D. Zhao, J. Wang, D. Wang, H. Yuan, X. Guo and B. Sun, High performance determination of Pb(2+) in water by 2,4-dithiobiuret-Reduced graphene oxide composite with wide linear range and low detection limit, Anal. Chim. Acta, 2020, 1125, 76–85 CrossRef CAS PubMed.
- G. Nie, L. Zhang, X. Lu, X. Bian, W. Sun and C. Wang, A one-pot and in situ synthesis of CuS-graphene nanosheet composites with enhanced peroxidase-like catalytic activity, Dalton Trans., 2013, 42, 14006–14013 RSC.
- M. Chen, J. Shu, Z. Wang and C. Ren, Porous surface MnO2 microspheres as oxidase mimetics for colorimetric detection of sulfite, J. Porous Mater., 2017, 24, 973–977 CrossRef CAS.
- F. Tan, Z. Wang, Y. Yang, X. Xie, X. Hua, X. Yang and H. Huang, Facile preparation of peroxidase-like core-shell nanorods and application
as platform for colorimetric determination of glucose, insulin and glucose/insulin ratio, Talanta, 2019, 204, 285–293 CrossRef CAS.
- Y. Long, Y. Pan, W. Zheng, D. Yi and H. Zheng, Supramolecular hydrogel-immobilized enzyme ficin as peroxidase mimics for colorimetric detection of glucose, Microchem. J., 2020, 158, 105276 CrossRef CAS.
- H. Q. Zheng, C. Y. Liu, X. Y. Zeng, J. Chen, J. Lu, R. G. Lin, R. Cao, Z. J. Lin and J. W. Su, MOF-808: A Metal-Organic Framework with Intrinsic Peroxidase-Like Catalytic Activity at Neutral pH for Colorimetric Biosensing, Inorg. Chem., 2018, 57, 9096–9104 CrossRef CAS.
- L. Su, W. Qin, H. Zhang, Z. U. Rahman, C. Ren, S. Ma and X. Chen, The peroxidase/catalase-like activities of MFe(2)O(4) (M=Mg, Ni, Cu) MNPs and their application in colorimetric biosensing of glucose, Biosens. Bioelectron., 2015, 63, 384–391 CrossRef CAS.
- H. Jiang, Z. Chen, H. Cao and Y. Huang, Peroxidase-like activity of chitosan stabilized silver nanoparticles for visual and colorimetric detection of glucose, Analyst, 2012, 137, 5560–5564 RSC.
Footnote |
† Electronic supplementary information (ESI) available. See DOI: 10.1039/d1ra04877e |
|
This journal is © The Royal Society of Chemistry 2021 |
Click here to see how this site uses Cookies. View our privacy policy here.