DOI:
10.1039/D1RA05269A
(Paper)
RSC Adv., 2021,
11, 27992-27999
Enhanced 1.5 μm emission from Yb3+/Er3+ codoped tungsten tellurite glasses for broadband near-infrared optical fiber amplifiers and tunable fiber lasers
Received
8th July 2021
, Accepted 13th August 2021
First published on 18th August 2021
Abstract
Strong 1.5 μm emission with full width at half maximum (FWHM) of 64 nm has been obtained in 3 mol% Yb2O3 and 1 mol% Er2O3 codoped tungsten tellurite glass under the excitation of a 980 nm laser diode. Spectroscopic properties of Yb3+/Er3+ codoped tungsten tellurite glasses as a function of Yb3+ and Er3+ contents are systematically investigated by absorption spectra, emission spectra and lifetime measurement. The structure of tungsten tellurite glass is characterized by Raman spectrum. In addition, emission cross section and gain coefficient of Er3+ ions near 1.5 μm are determined and respective maximum values attain 1.06 × 10−20 cm2 and 4.07 cm−1. Moreover, gain bandwidth and figure of merit associated with gain properties in tungsten tellurite glass are calculated and compared with other reported glasses. These results indicate that Yb3+/Er3+ codoped tungsten tellurite glasses with better gain properties are promising candidates in constructing broadband optical fiber amplifiers and tunable fiber lasers in the optical telecommunication window.
1. Introduction
In the past few decades, wavelength-division-multiplexing (WDM) technology has been extensively used in optical communication systems to meet the rapid increase in demand for information transmission capacity of networks.1–3 Optical fiber amplifiers with broadband gain bandwidth and high gain are the key devices for WDM network systems.4 Since the Er3+:4I13/2 → 4I15/2 radiative transition emits fluorescence at around 1.5 μm that lies in the third optical telecommunication window and Er3+ ions can be excited by high power and low cost 980 nm laser diode (LD), Er3+ doped fiber amplifiers (EDFA) were initially developed and have been widely used in WDM network systems.5 At present, most of the commercial EDFA adopted in WDM network systems are made of silica glass. However, silica glass has relatively narrow emission bandwidth at 1.5 μm (∼35 nm) and lower doping concentration of Er3+ ions resulting in narrow and low gain profile, which limits data transmission capacity of WDM systems.2,6
In order to overcome these shortcomings and achieve broad gain bandwidth and high gain, a lot of works have been dedicated to the exploitation for new glass host materials such as silicate, phosphate, germanate and tellurite glasses.7–10 Among these glass hosts, tellurite glasses own relatively low phonon energy (650–800 cm−1), broad infrared transmission region (up to 5 μm), high rare-earth solubility and refractive index (∼2).11 It is very attractive that tellurite glasses possess a variety of structural units such as TeO4 trigonal bipyramid, TeO3+δ polyhedron and TeO3 trigonal pyramid, which creates a range of electro-static fields around rare earth ions and thus results in inhomogeneous broadening of the spectrum.12 Broad gain amplification of more than 20 dB in the wavelength range from 1530 to 1610 nm has been demonstrated in EDFA based on tellurite glass.10 In addition, WO3 not only improves the thermal stability of tellurite glasses but also creates new structural units such as WO4 tetrahedra and WO6 octahedra.13–15 Therefore, further inhomogeneous broadening can be expected in tungsten tellurite glasses. On the other hand, co-doping Yb3+ ions play a role of sensitization for Er3+ ions because Yb3+ ions with larger absorption cross section at 980 nm than Er3+ ions can enhance the pump efficiency of 980 nm LD and then transfer the energy to Er3+:4I11/2 energy level via resonant energy transfer process (Yb3+:2F5/2 + Er3+:4I15/2 → Yb3+:2F7/2 + Er3+:4I11/2).5 To further enhance 1.5 μm emission from Er3+ through Yb3+ → Er3+, the optimization of Yb3+ and Er3+ doping concentrations are very essential.
In the present work, we systematically study spectroscopic properties of Yb3+/Er3+ codoped tungsten tellurite glasses as a function of Yb3+ and Er3+ concentrations by absorption spectra, emission spectra and lifetime measurement. The structure of tungsten tellurite glass is characterized by Raman spectrum. Furthermore, Judd–Ofelt (J–O) intensity parameters, emission cross section and gain coefficient of Er3+:4I13/2 → 4I15/2 transition are evaluated based on absorption and emission spectra. Besides, gain bandwidth and figure of merit associated with gain properties in tungsten tellurite glass are calculated and compared with other reported glasses.
2. Experimental methods
Tungsten tellurite glasses with different nominal compositions (in mol%) of 60TeO2–30WO3–3ZnO–7La2O3, 60TeO2–30WO3–3ZnO–(6 − x)La2O3–1Er2O3–xYb2O3 (x = 0, 1, 2, 3 and 4) and 60TeO2–30WO3–3ZnO–(4 − y)La2O3–3Yb2O3–yEr2O3 (y = 0, 0.5, 1, 1.5, 2 and 3) which are henceforth labeled as TW, TWEY-x and TWYE-y, respectively, were synthesized by standard melt-quenching method. Since La, Er and Yb belong to lanthanide family, La3+ is easily replaced by Er3+ and Yb3+, which is beneficial to the enhancement of doping concentrations of Er3+ and Yb3+ in the host glass. La2O3 may act as glass network modifier. In addition, the addition of ZnO can decrease the melting temperature of the host glass and increase the glass formation ability. All high-purity reagents (99.99% minimum) were weighed and mixed thoroughly before batches of 15 g mixtures were melted in alumina crucibles in an electric furnace at ∼850 °C for 30 min. These melts were cast into preheated cylindrical graphite molds and annealed at 450 °C for 2 h to relax the internal stresses, followed by slow cooling to room temperature. Finally, the annealed samples were optically polished to the disks with the size of Φ 15 mm × 1.5 mm for optical measurements.
Glass density (ρ) was calculated by Archimedes' principle using distilled water as an immersed liquid. After obtaining glass density, the total population of Er3+ or Yb3+ ions (N) was determined by the equation:
|
 | (1) |
where
N's unit is cm
−3,
x stands for the doping concentration of Er
3+ or Yb
3+ ions (mol%),
M represents the average molecular mass of glass and
NA is Avogadro's number.
The refractive indices at 632.8, 1309 and 1533 nm were obtained by the prism coupling method (Metricon model 2010/M, Metricon Corp., America). Raman spectrum was measured by Raman spectrometer (Renishaw inVia, Gloucestershire, UK) and 532 nm laser as the excitation source. Absorption spectra of samples were recorded by Perkin-Elmer Lambda 900 UV/VIS/NIR double beam spectrophotometer (Waltham, MA, America) in the wavelength range from 400 to 2000 nm. The fluorescence spectra were recorded with a computer-controlled iHR 320 spectrofluorometer (Jobin-Yvon Corp., Horiba Scientific, France) under the excitation of a 980 nm LD. The fluorescence signal was collected by InGaAs detector. In addition, the luminescence decay curves of Er3+ were measured by a digital oscilloscope (TDS3012C, Tektronix Inc., America) and a function generator (TFG3051C, Tektronix Inc., America) connected to the iHR 320 spectrofluorometer. All of the measurements were carried out at room temperature.
3. Results and discussion
3.1 Raman and absorption spectra
Fig. 1 represents Raman spectrum of undoped tungsten tellurite glass (TW) in the wavenumber range of 200–1200 cm−1. It is observed that five main bands appear in tungsten tellurite glass. The peak A at ∼360 cm−1 and sharp peak E at ∼930 cm−1 are attributed to the bending vibrations of corner-shared WO6 octahedra and the vibrations of W–O− and W
O bonds in WO4 tetrahedra, respectively.13–15 In addition, the peak B, C and D at ∼450, 678 and 750 cm−1 are assigned to the symmetrical stretching or bending vibrations of Te–O–Te linkages at corner sharing sites, the anti-symmetric stretching vibrations of Te–O–Te linkages constructed by two un-equivalent Te–O bonds containing bridging oxygens (BO) in TeO4 trigonal bipyramid and the symmetrical stretching vibrations of Te–O− and Te
O bonds with non-bridging oxygens (NBO) in TeO3 trigonal pyramid and TeO3+1 polyhedra, respectively.15,16 Therefore, very diverse types of structural units in the present glass can provide various local ligand field environments for Er3+ ions in favor of inhomogeneous broadening of 1.5 μm emission band.
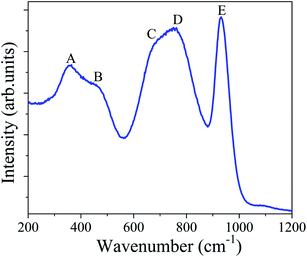 |
| Fig. 1 Raman spectrum of TW glass. | |
Fig. 2 depicts the absorption spectra of Yb3+ singly doped, Er3+ singly doped and Yb3+/Er3+ codoped tungsten tellurite glasses in the wavelength range from 400 to 2000 nm. Different characteristic absorption bands from Yb3+ and Er3+ ions are labeled in the figure. For Er3+ singly doped glass, eight absorption bands are located at 1532, 978, 798, 654, 544, 522, 490 and 452 nm, which are assigned to respective transitions from the 4I15/2 ground state to the higher excited states 4I13/2, 4I11/2, 4I9/2, 4F9/2, 4S3/2, 2H11/2, 4F7/2 and 4F5/2. Energy levels above 4F5/2 energy level are not clearly distinguished due to strong intrinsic bandgap absorption in the host glass. It is worth mentioning that absorption bands centered at 978 and 798 nm well match with the laser wavelength of commercial high power 980 and 800 nm LD. Based on the Beer–Lambert equation,17 absorption cross section at 978 nm (3.45 × 10−21 cm2) is 2.6 times as high as that at 798 nm (1.32 × 10−21 cm2), indicating that more efficient pump absorption can be achieved under the excitation of 980 nm LD. In addition, absorption intensity at 978 nm is greatly enhanced by the addition of Yb3+ ions due to Yb3+:2F7/2 → 2F5/2 transition. Therefore, Yb3+/Er3+ codoped tungsten tellurite glasses can be efficiently pumped by 980 nm LD. The inset of Fig. 2 presents the integral absorption intensity at 654 nm as a function of Er2O3 concentration. Good linear fitting implies that Er3+ ions are uniformly distributed without phase separation and agglomeration in the present tungsten tellurite glasses.18
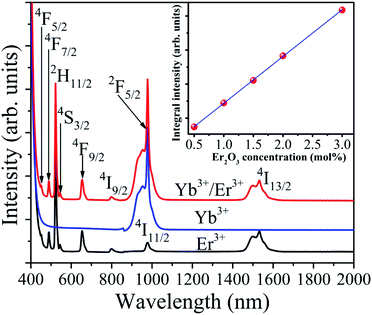 |
| Fig. 2 Absorption spectra of TWEY-0, TWYE-0 and TWYE-1 glasses. The inset shows the integral absorption intensity at 654 nm as a function of Er2O3 concentration. | |
It is well known that J–O theory19,20 is a common tool to analyze spectroscopic properties of rare-earth (RE) ions doped crystals and glasses. With the help of this theory, three J–O intensity parameters Ωt (t = 2, 4, 6) of Er3+ ions in TWEY-0 glass are determined using Fig. 2 and the procedure introduced elsewhere.21 Three J–O intensity parameters of Er3+ doped TWEY-0 glass are compared with that in other typical glass hosts in Table 1. The root mean square deviation for the best-fitted oscillator strengths is only 2.2 × 10−7 for TWEY-0 glass, indicating that calculated J–O intensity parameters are dependable. Higher Ω2 implies that the degree of asymmetry of coordination environment for RE ions is higher and the covalency between RE ions and ligand is stronger.21 It is found that Ω2 of Er3+ doped TWEY-0 glass is higher than that of other glass hosts in Table 1, resulted from the co-existence of various structural units as shown in Fig. 1, indicating that Er–O bond in the present glass possesses stronger covalency and coordination environment for Er3+ ions is of low symmetry.
Table 1 J–O intensity parameters in Er3+ doped TWEY-0 glass and other glass hosts
Glasses |
J–O intensity parameters (10−20 cm2) |
References |
Ω2 |
Ω4 |
Ω6 |
Oxyfluoroborosilicate |
6.69 |
3.08 |
3.28 |
22 |
Phosphate |
3.50 |
0.78 |
0.57 |
23 |
Fluoride |
3.74 |
1.78 |
1.50 |
24 |
TeO2–GeO2–Na2O–Nb2O5 |
6.23 |
1.66 |
1.25 |
25 |
TWEY-0 |
6.89 |
1.85 |
1.14 |
This work |
3.2 Fluorescence spectra, lifetime and energy transfer mechanism
To investigate the effect of Yb3+ concentration on spectra properties of TWEY-x glasses, near-infrared and upconversion fluorescence spectra are recorded upon 980 nm excitation, as shown in Fig. 3. It is noted from Fig. 3(a) that a strong and broad emission band is located at 1536 nm, attributed to Er3+:4I13/2 → 4I15/2 transition. Furthermore, with increasing Yb2O3 concentration from 0 to 3 mol%, this emission intensity is enhanced by about 3 times, which is due to the reduction of the distance between Yb3+ and Er3+ ions and thus increases the energy transfer probability from Yb3+:2F5/2 to Er3+:4I11/2 energy level. But the emission intensity begins to reduce with further increasing the concentration, which may be ascribed to concentration quenching phenomenon and the enhancement of upconversion processes, as presented in Fig. 3(b). Three visible fluorescence peaks around 524, 546 and 658 nm appear, corresponding to Er3+:2H11/2 → 4I15/2, 4S3/2 → 4I15/2 and 4F9/2 → 4I15/2 transitions, respectively. Moreover, with the increment of Yb3+ content, three visible fluorescence intensities monotonously increase due to the enhancement of energy transfer process from Yb3+:2F5/2 to Er3+:4I11/2 energy level. Consequently, the optimum concentration of Yb2O3 is 3 mol% in view of 1.5 μm emission intensity.
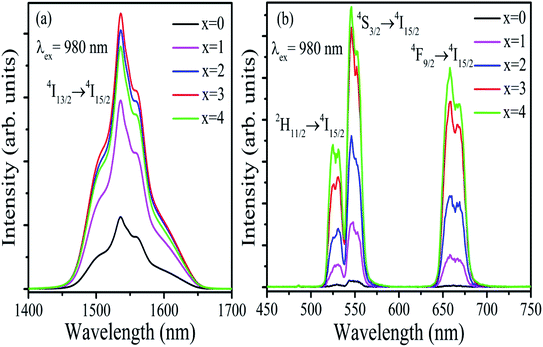 |
| Fig. 3 (a) Near-infrared and (b) upconversion spectra of TWEY-x glasses upon 980 nm excitation. | |
In the case where Yb2O3 concentration is fixed at 3 mol%, near-infrared emission spectra in TWYE-y glasses with different Er2O3 concentration are measured and depicted in Fig. 4 to further discuss energy transfer from Yb3+ to Er3+ ions. It is found from Fig. 4(a) that with increasing Er2O3 concentration from 0 to 3 mol%, 1018 nm emission intensity from Yb3+:2F5/2 → 2F7/2 transition decreases monotonously, which provides evidence for energy transfer process from Yb3+:2F5/2 to Er3+:4I11/2 energy level again. It should be mentioned that the emission band centered at 1018 nm can't be completely recorded upon 980 nm excitation because of getting close to the pump wavelength.
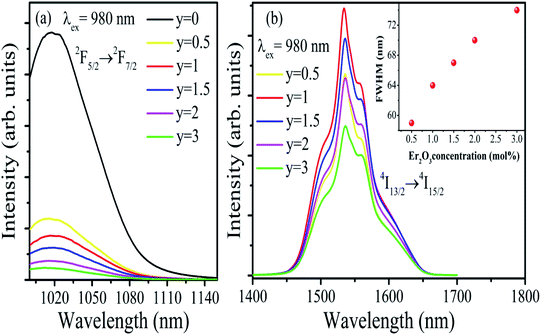 |
| Fig. 4 Near-infrared spectra of TWYE-y glasses in the wavelength range of (a) 1000–1150 nm and (b) 1400–1700 nm pumped by 980 nm LD. The inset shows Er2O3 concentration dependence of FWHM. | |
The influence of Er2O3 content on 1.5 μm emission intensity and full width at half maximum (FWHM) is presented in Fig. 4(b). With the argument of Er2O3 concentration, this intensity heightens gradually due to enhanced energy transfer probability from Yb3+ to Er3+ before it reaches the maximum value of 1 mol%. And then the intensity decreases gradually with further enhancing the concentration owing to diffusion towards OH− and other impurities present in the starting materials.26 The broadening related to 1.5 μm emission band is very important for EDFA, which is usually evaluated by FWHM. Large FWHM is beneficial for wavelength tuning laser and WDM system.27 The inset of Fig. 4(b) shows that FWHM of 4I13/2 → 4I15/2 emission band increases gradually from 59 to 74 nm when Er2O3 concentration varies from 0.5 to 3 mol%. These values are larger than that in other glass hosts used for optical fiber amplifiers, such as 38 nm in lead borosilicate glasses,28 47 nm in lead phosphate glasses,29 46 nm in niobium germanate glasses30 and 48 nm in fluorotellurite glasses.5 The larger FWHM is ascribed to not only high refractive index (∼2.1) which strengthens the ligand fields around Er3+ but also to the existence of multiple structural units such as TeO4 trigonal bipyramid, TeO3 trigonal pyramid, TeO3+1 polyhedra, WO6 octahedra and WO4 tetrahedra, which creates diverse coordination environments. Therefore, it is in favor of covering the optical communication C (1530–1565 nm) and L (1565–1625 nm) bands and the achievement of tunable fiber lasers.
Fig. 5 shows the fluorescence decay curve of Er3+:4I13/2 energy level monitored at 1536 nm in TWYE-1 glass along with the lifetime values in TWYE-y glass. It is found that the lifetime of Er3+:4I13/2 energy level monotonously reduces from 3.96 to 2.44 ms as Er2O3 concentration increases from 0.5 to 3 mol%. It is worth noting that the lifetime value slightly changes and is still relatively long (3.83 ms) in TWYE-1 glass.
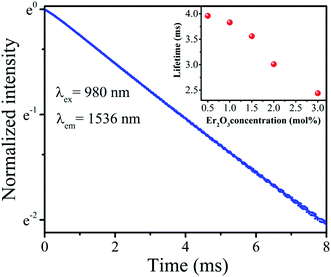 |
| Fig. 5 Fluorescence decay curves of Er3+:4I13/2 energy level monitored at 1536 nm in TWYE-1 glass. The inset shows Er2O3 concentration dependence of the lifetime. | |
Based on above-mentioned results, the related energy transfer (ET) mechanism in Er3+/Yb3+ codoped tungsten tellurite glasses is illustrated in Fig. 6 with the help of the simplified energy level diagram of Er3+ and Yb3+. When samples are excited by 980 nm LD, both Yb3+ and Er3+ ions are initially motivated from the ground states 2F7/2 and 4I15/2 to the excited states 2F5/2 and 4I11/2, respectively. Since absorption cross section of Yb3+ ions at this wavelength is much larger than that of Er3+ ions and Yb3+:2F5/2 and Er3+:4I11/2 energy levels are closely in resonance, a majority of Yb3+ ions transfer their energy to nearby Er3+ ions through energy transfer process (ET1): Yb3+:2F5/2 + Er3+:4I15/2 → Yb3+:2F7/2 + Er3+:4I11/2, except that a small part of ions de-excite radiatively to 2F7/2 energy level and emits fluorescence at 1018 nm. Thereafter, a part of Er3+ ions on this energy level relax radiatively and non-radiatively (NR) to Er3+:4I13/2 and then return radiatively to the ground state producing fluorescence at 1.5 μm while the remainder is excited to 4F7/2 energy level via excited state absorption (ESA), ET2: Yb3+:2F5/2 + Er3+:4I11/2 → Yb3+:2F7/2 + Er3+:4F7/2 and energy transfer upconversion process (ETU1): Er3+:4I11/2 + Er3+:4I11/2 → Er3+:4I15/2 + Er3+:4F7/2. Hence, the green emitting energy levels of 2H11/2 and 4S3/2 can be populated by relaxing NR from the upper 4F7/2 energy level, followed by radiative transitions of 2H11/2 → 4I15/2 and 4S3/2 → 4I15/2 emitting fluorescence at 524 and 546 nm. On the other hand, the red emitting energy level of 4F9/2 can be populated by ESA, ET3: Yb3+:2F5/2 + Er3+:4I13/2 → Yb3+:2F7/2 + Er3+:4F9/2 and relaxing NR from 2H11/2 and 4S3/2 energy levels. Then radiative transition of 4F9/2 → 4I15/2 produces fluorescence at 658 nm. It is worth noting that both ESA from Er3+:4I13/2 and ETU2: Er3+:4I13/2 + Er3+:4I13/2 → Er3+:4I15/2 + Er3+:4I9/2 depopulate 4I13/2 energy level and thus lead to decreased emission intensity at 1.5 μm.
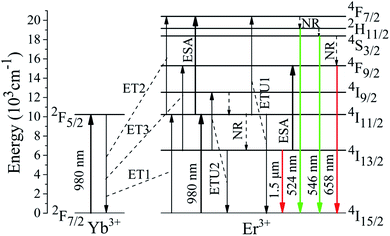 |
| Fig. 6 Simplified energy level diagram and energy transfer mechanism for Yb3+/Er3+ codoped tungsten tellurite glasses. | |
3.3 Gain properties of Er3+/Yb3+ codoped tungsten tellurite glasses
Emission cross section of Er3+:4I13/2 → 4I15/2 transition is very crucial parameter to evaluate the suitability for broadband EDFA. The emission cross section (σe) can be estimated by the following McCumber formula:31 |
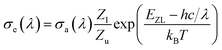 | (2) |
where σa is absorption cross section, Zl and Zu represent the partition function of the lower and upper state for 4I13/2 → 4I15/2 transition, respectively, kB is the Boltzmann constant, T is the temperature and EZL is the zero-line energy, defined as the energy difference between the lowest stark of the upper and lower manifolds. The absorption cross section can be estimated by the Beer–Lambert equation:17 |
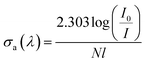 | (3) |
where I0 and I represent the intensities of incident and transmitted light, respectively, N is the total population of Er3+ ions and l is the thickness of the sample. According to eqn (1), the total population of Er3+ ions in TWYE-1 glass reaches 3.84 × 1020 cm−3 (2 mol%). Fig. 7(a) presents the absorption and emission cross section as a function of wavelength in TWYE-1 glass. It is found that the maximum σe of Er3+ reaches 1.06 × 10−20 cm2 near 1.5 μm which is larger than those values reported in oxyfluorosilicate glass (4.88 × 10−21 cm2),32 lead phosphate glass (4.9 × 10−21 cm2),29 bismuthate glass (8.2 × 10−21 cm2)33 and gallogermanate glass (7.6 × 10−21 cm2),2 indicating that high gain coefficient and low pump threshold can be excepted in the present glass.34 Large emission cross section of Er3+ in TWYE-1 glass is due to high refractive index. The measured refractive indices at 632.8, 1309 and 1533 nm in TWYE-1 glass reach 2.0673, 2.0186 and 2.0152, respectively.
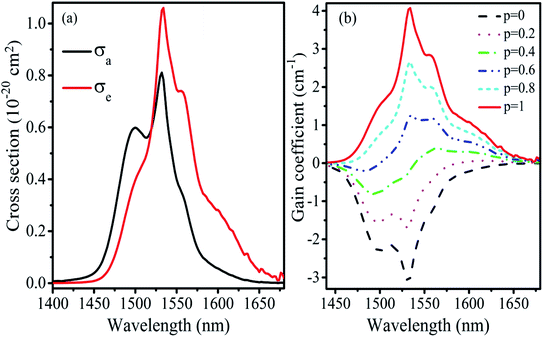 |
| Fig. 7 (a) Absorption and emission cross sections of Er3+:4I13/2 ↔ 4I15/2 transitions (b) the effect of 4I13/2 → 4I15/2 transition wavelength on the gain coefficient in TWYE-1 glass. | |
Assuming that Er3+ ions are only in either 4I15/2 ground state or 4I13/2 first excited state, the gain coefficient G(λ) near 1.5 μm associated with the absorption and emission cross section is evaluated by the expression to estimate the gain properties qualitatively:35
|
G(λ) = N[pσe − (1 − p)σa]
| (4) |
where
N stands for the total population of Er
3+ ions and
p represents the population inversion defined as the ratio between the population at
4I
13/2 energy level and the total population.
Fig. 7(b) depicts the gain coefficient of Er
3+:
4I
13/2 →
4I
15/2 transition as a function of the wavelength in TWYE-1 glass while
p increases from 0 to 1 in step of 0.2. It is noticed that respective wavelength of the maximum gain shifts toward shorter wavelength side as
p raises, revealing a typical characteristic of the quasi-three-level system. Moreover, positive gain coefficient is obtained when
p is higher than 0.2, indicating a lower pump threshold. It is worth noting that the maximum gain coefficient of TWYE-1 glass is 4.07 cm
−1 at 1534 nm, which is larger than those values reported in borosilicate glass (1.01 cm
−1),
22 phosphate glasses (1.02 cm
−1),
36 bismuthate glass (3.51 cm
−1)
33 and niobium germanate glass (0.28 cm
−1).
30
Gain bandwidth is a key parameter for optical fiber amplifiers and is usually defined as the product of FWHM and emission cross section (FWHM × σe).37 The larger FWHM × σe implies the better properties of amplifier. FWHM × σe in TWYE-1 glass reaches 6.78 × 10−26 cm,3 which is larger than those values reported in silicate glass (2.2 × 10−26 cm3),38 zincfluorophosphate glass (1.61 × 10−26 cm3),27 gallogermanate glass (4.02 × 10−26 cm3)2 and zinctellurite glass (4.91 × 10−26 cm3).37 On the other hand, the figure of merit (FOM) is another vital parameter for optical fiber amplifiers to achieve high gain amplification, which is usually defined as the product of emission cross section and measured lifetime.39 The larger FOM indicates a lower pump threshold. FOM in TWYE-1 glass reaches 4.06 × 10−23 cm2 s, which is higher than that in silicate glass (2.09 × 10−23 cm2 s),38 zincfluorophosphate glass (1.26 × 10−23 cm2 s),27 germanate glass (2.89 × 10−23 cm2 s)40 and fluorotellurite glass (1.84 × 10−23 cm2 s).38 As a consequence, Yb3+/Er3+ codoped tungsten tellurite glasses with larger emission cross section and better gain properties are potential candidates for broadband optical fiber amplifiers and tunable fiber lasers.
4. Conclusions
In conclusion, Yb3+/Er3+ codoped tungsten tellurite glasses with a series of doping concentration were prepared and their spectroscopic characteristics dependence of Yb3+ and Er3+ concentrations under 980 nm excitation were measured and studied in detail. Strong 1.5 μm emission with FWHM of 64 nm has been achieved in tungsten tellurite glass codoped with 3 mol% Yb2O3 and 1 mol% Er2O3, profiting from enormous absorption coefficient of Yb3+ ions at the pump wavelength and efficient resonance energy transfer process from Yb3+:2F5/2 to Er3+:2I11/2 energy level. Furthermore, the present glass owns multiple structural units and shows long lifetime of Er3+:4I13/2 energy level (3.96 ms), high emission cross section (1.06 × 10−20 cm2) and gain coefficient (4.07 cm−1) near 1.5 μm, resulting in larger gain bandwidth (6.78 × 10−26 cm3) and figure of merit (4.06 × 10−23 cm2 s) than those values reported in other glasses. In consequence, these results indicate that Yb3+/Er3+ codoped tungsten tellurite glasses with better gain properties are promising candidates in constructing broadband optical fiber amplifiers and tunable fiber lasers in the optical telecommunication window.
Author contributions
Jian Yuan conceived the idea and supervised the experimental work and paper writing. Guodong Zheng and Yingyi Ye performed the sample preparation. Yongbin Chen and Yichen Ye performed the optical measurements. Tingting Deng, Peng Xiao and Weichao Wang contributed to data analysis and secured funding. All authors reviewed the manuscript.
Conflicts of interest
There are no conflicts to declare.
Acknowledgements
The authors gratefully acknowledge the financial support from the National Natural Science Foundation of China (Grant No. 51902053, 61804029), Natural Science Foundation of Guangdong Province (Grant No. 2019A1515011988), Guangdong Basic and Applied Basic Research Foundation (Grant No. 2019A1515110002), the Foundation for Distinguished Young Talents in Higher Education of Guangdong (Grant No. 2019KQNCX172), the Project of Foshan Education Bureau (Grant No. 2019XJZZ02), Research Fund of Guangdong-Hong Kong-Macao Joint Laboratory for Intelligent Micro-Nano Optoelectronic Technology (Grant No. 2020B1212030010), the Open Fund of the State Key Laboratory of Luminescent Materials and Devices (South China University of Technology, Grant No. 2020-skllmd-13) and the Open Fund of the Guangdong Provincial Key Laboratory of Fiber Laser Materials and Applied Techniques (South China University of Technology, Grant No. 2021-07).
References
- H. Hu and L. K. Oxenløwe, Chip-based optical frequency combs for high-capacity optical communications, Nanophotonics, 2021, 10(5), 1367–1385 CrossRef.
- D. M. Shi, Y. G. Zhao, X. F. Wang, G. H. Liao, C. Zhao, M. Y. Peng and Q. Y. Zhang, Effects of alkali ions on thermal stability and spectroscopic properties of Er3+-doped gallogermanate glasses, Phys. B, 2011, 406, 628–632 CrossRef CAS.
- A. Q. L. Quang, V. G. Truong, A. M. Jurdyc, B. Jacquier, J. Zyss and I. Ledoux, Gain properties of an Er3+ complex in a poly(methylmethacrylate) matrix for 1540 nm broadband optical amplification, J. Appl. Phys., 2007, 101(2), 023110 CrossRef.
- Q. L. Chen, H. Wang, Q. W. Wang and Q. P. Chen, Structural study of the origin of the largest 1.5 μm Er3+ luminescence band width in multicomponent silicate glass, J. Non-Cryst. Solids, 2014, 404, 145–150 CrossRef CAS.
- J. M. Liu, X. Huang, H. Pan, X. Zhang, X. J. Fang, W. X. Li, H. Zhang, A. P. Huang and Z. S. Xiao, Broadband near infrared emission of Er3+/Yb3+ co-doped fluorotellurite glass, J. Alloys Compd., 2021, 866, 158568 CrossRef CAS.
- K. Linganna, M. Rathaiah, N. Vijaya, Ch. Basavapoornima, C. K. Jayasankar, S. Ju, W. T. Han and V. Venkatramu, 1.53 μm luminescence properties of Er3+-doped K–Sr–Al phosphate glasses, Ceram. Int., 2015, 41, 5765–5771 CrossRef CAS.
- M. P. Hehlen, N. J. Cockroft, T. R. Gosnell and A. J. Bruce, Spectroscopic properties of Er3+- and Yb3+-doped soda-lime silicate and aluminosilicate glasses, Phys. Rev. B: Condens. Matter Mater. Phys., 1997, 56(15), 9302–9318 CrossRef CAS.
- Y. Zhang, M. M. Li, J. Li, J. Tang, W. J. Cao and Z. N. Wu, Optical properties of Er3+/Yb3+ co-doped phosphate glass system for NIR lasers and fiber amplifiers, Ceram. Int., 2018, 44, 22467–22472 CrossRef CAS.
- T. Wei, F. Z. Chen, Y. Tian and S. Q. Xu, 1.53 μm emission properties in Er3+ doped Y2O3 and Nb2O5 modified germanate glasses for an optical amplifier, J. Lumin., 2014, 154, 41–45 CrossRef CAS.
- Y. Ohishi, A. Mori, M. Yamada, H. Ono, Y. Nishida and K. Oikawa, Gain characteristics of tellurite-based erbium-doped fiber amplifiers for 1.5 μm broadband amplification, Opt. Lett., 1998, 23(4), 274–276 CrossRef CAS PubMed.
- B. Richards, A. Jha, Y. Tsang, D. Binks, J. Lousteau, F. Fusari, A. Lagatsky, C. Brown and W. Sibbett, Tellurite glass lasers operating close to 2 μm, Laser Phys. Lett., 2010, 7(3), 177–193 CrossRef CAS.
- A. Jha, S. Shen and M. Naftaly, Structural origin of spectral broadening of 1.5 μm emission in Er3+-doped tellurite glasses, Phys. Rev. B: Condens. Matter Mater. Phys., 2000, 62(10), 6215–6227 CrossRef CAS.
- B. V. R. Chowdari and P. P. Kumari, Raman spectroscopic study of ternary silver tellurite glasses, Mater. Res. Bull., 1999, 34(2), 327–342 CrossRef CAS.
- J. Yuan, Q. Yang, D. D. Chen, Q. Qian, S. X. Shen, Q. Y. Zhang and Z. H. Jiang, Compositional effect of WO3, MoO3, and P2O5 on Raman spectroscopy of tellurite glass for broadband and high gain Raman amplifier, J. Appl. Phys., 2012, 111(10), 103511 CrossRef.
- R. Jose, Y. Arai and Y. Ohishi, Raman scattering characteristics of the TBSN-based tellurite glass system as a new Raman gain medium, J. Opt. Soc. Am. B, 2007, 24(7), 1517–1526 CrossRef CAS.
- J. Yuan, W. C. Wang, Y. C. Ye, T. T. Deng, D. Q. Ou, J. Y. Cheng, S. J. Yuan and P. Xiao, Effect of BaF2 variation on spectroscopic properties of Tm3+ doped gallium tellurite glasses for efficient 2.0 μm laser, Front. Chem., 2021, 8, 628273 CrossRef PubMed.
- K. F. Li, Q. Zhang, G. X. Bai, S. J. Fan, J. J. Zhang and L. L. Hu, Energy transfer and 1.8 μm emission in Tm3+/Yb3+ codoped lanthanum tungsten tellurite glasses, J. Alloys Compd., 2010, 504, 573–578 CrossRef CAS.
- W. C. Wang, L. Y. Mao, J. L. Liu and S. H. Xu, Glass-forming regions and enhanced 2.7 μm emission by Er3+ heavily doping in TeO2–Ga2O3–R2O (or MO) glasses, J. Am. Ceram. Soc., 2020, 103, 4999–5012 CrossRef CAS.
- B. R. Judd, Optical absorption intensities of rare-earth ions, Phys. Rev., 1962, 127(3), 750–761 CrossRef CAS.
- G. S. Ofelt, Intensities of crystal spectra of rare-earth ions, J. Chem. Phys., 1962, 37(3), 511–520 CrossRef CAS.
- Y. A. Lakshmi, K. Swapna, K. S. R. K. Reddy, M. Venkateswarlu, Sk. Mahamuda and A. S. Rao, Structural and optical NIR studies of Er3+ ions doped bismuth boro tellurite glasses for luminescence materials applications, J. Lumin., 2019, 211, 39–47 CrossRef.
- M. Rajesh, M. R. Babu, N. J. Sushma and B. D. P. Raju, Influence of Er3+ ions on structural and fluorescence properties of SiO2–B2O3–Na2CO3–NaF–CaF2 glasses for broadband 1.53 μm optical amplifier applications, J. Non-Cryst. Solids, 2020, 528, 119732 CrossRef CAS.
- A. A. Reddy, S. S. Babu and G. V. Prakash, Er3+-doped phosphate glasses with improved gain characteristics for broadband optical amplifiers, Opt. Commun., 2012, 285, 5364–5367 CrossRef.
- F. F. Huang, Y. Tian, S. Q. Xu and J. J. Zhang, Spectroscopic and energy transfer mechanism of Er3+, Pr3+-codoped ZBYA glass, Ceram. Int., 2016, 42, 7924–7928 CrossRef CAS.
- O. B. Silva, V. A. G. Rivera, Y. Ledemi, Y. Messaddeq and E. Marega Jr, Germanium concentration effects on the visible emission properties of Er3+ in tellurite glasses, J. Lumin., 2021, 232, 117808 CrossRef CAS.
- H. Gebavi, D. Milanese, R. Balda, S. Chaussedent, M. Ferrari, J. Fernandez and M. Ferraris, Spectroscopy and optical characterization of thulium doped TZN glasses, J. Phys. D: Appl. Phys., 2010, 43, 135104 CrossRef.
- V. B. Sreedhar, N. Vijaya, D. Ramachari and C. K. Jayasankar, Luminescence studies on Er3+-doped zincfluorophosphate glasses for 1.53 μm laser applications, J. Mol. Struct., 2017, 1130, 1001–1008 CrossRef CAS.
- M. R. Babu, N. M. Rao and A. M. Babu, Effect of erbium ion concentration on structural and luminescence properties of lead borosilicate glasses for fiber amplifiers, Luminescence, 2018, 33(1), 71–78 CrossRef CAS.
- Ch. Basavapoornima, T. Maheswari, S. R. Depuru and C. K. Jayasankar, Sensitizing effect of Yb3+ ions on photoluminescence properties of Er3+ ions in lead phosphate glasses: Optical fiber amplifiers, Opt. Mater., 2018, 86, 256–269 CrossRef CAS.
- L. M. Marcondes, C. R. D. Cunha, B. P. D. Sousa, S. Maestri, R. R. Gonçalves, F. C. Cassanjes and G. Y. Poirier, Thermal and spectroscopic properties studies of Er3+-doped and Er3+/Yb3+-codoped niobium germanate glasses for optical applications, J. Lumin., 2019, 205, 487–494 CrossRef CAS.
- D. E. McCumber, Einstein relations connecting broadband emission and absorption spectra, Phys. Rev., 1964, 136(4A), 954–957 CrossRef CAS.
- G. Devarajulu, O. Ravi, C. M. Reddy, S. Z. A. Ahamed and B. D. P. Raju, Spectroscopic properties and upconversion studies of Er3+-doped SiO2–Al2O3–Na2CO3–SrF2–CaF2 oxyfluoride glasses for optical amplifier applications, J. Lumin., 2018, 194, 499–506 CrossRef CAS.
- X. Y. Song, D. Y. Jin, D. C. Zhou, P. F. Xu and K. X. Han, Er3+/Yb3+ co-doped bismuthate glass and its large-mode-area double-cladding fiber for 1.53 μm laser, J. Alloys Compd., 2021, 853, 157305 CrossRef CAS.
- H. Desirena, E. D. L. Rosa, V. H. Romero, J. F. Castillo, L. A. Díaz-Torres and J. R. Oliva, Comparative study of the spectroscopic properties of Yb3+/Er3+ codoped tellurite glasses modified with R2O (R = Li, Na and K), J. Lumin., 2012, 132, 391–397 CrossRef CAS.
- X. L. Zou and H. Toratani, Spectroscopic properties and energy transfers in Tm3+ singly- and Tm3+/Ho3+ doubly-doped glasses, J. Non-Cryst. Solids, 1996, 195, 113–124 CrossRef CAS.
- A. A. Reddy, S. S. Babu, K. Pradeesh, C. J. Otton and G. V. Prakash, Optical Properties of highly Er3+-doped sodium-aluminium–phosphate glasses for broadband 1.5 μm emission, J. Alloys Compd., 2011, 509, 4047–4052 CrossRef CAS.
- I. Jlassi, H. Elhouichet, M. Ferid and C. Barthou, Judd-Ofelt analysis and improvement of thermal and optical properties of tellurite glasses by adding P2O5, J. Lumin., 2010, 130, 2394–2401 CrossRef CAS.
- M. Pokhrel, G. A. Kumar, S. Balaji, R. Debnath and D. K. Sardar, Optical characterization of Er3+ and Yb3+ co-doped barium fluorotellurite glass, J. Lumin., 2012, 132, 1910–1916 CrossRef CAS.
- K. Linganna, G. L. Agawane and J. H. Choi, Longer lifetime of Er3+/Yb3+ co-doped fluorophosphate glasses for optical amplifier applications, J. Non-Cryst. Solids, 2017, 471, 65–71 CrossRef CAS.
- T. Wei, Y. Tian, F. Z. Chen, M. Z. Cai, J. J. Zhang, X. F. Jing, F. C. Wang, Q. Y. Zhang and S. Q. Xu, Mid-infrared fluorescence, energy transfer process and rate equation analysis in Er3+ doped germanate glass, Sci. Rep., 2014, 4, 6060 CrossRef CAS PubMed.
|
This journal is © The Royal Society of Chemistry 2021 |
Click here to see how this site uses Cookies. View our privacy policy here.