DOI:
10.1039/D1RA05376K
(Paper)
RSC Adv., 2021,
11, 32454-32458
Removal of radioactive cesium from contaminated water by whey protein amyloids–carbon hybrid filters
Received
13th July 2021
, Accepted 14th September 2021
First published on 1st October 2021
Abstract
We report on the application of an innovative whey protein amyloids–carbon hybrid filter for the removal and disposal of the long-lived radioactive fission product 137Cs from aqueous samples. Test experiments revealed a reduction of 137Cs radioactivity by a factor of 340 compared to the initial solution, with an efficiency as high as 99.7%. The adsorption capacity of the membrane was explored by performing several cycles of filtration, indicating a potential retention of more than 115 MBq per gram of filtering material at the applied experimental conditions. These results pave the way for further investigations on the applicability of this filter material to other nuclear fission products.
1. Introduction
Radioactive waste is in general a burden to the public. Currently, the storage and/or disposal of large volumes of low- and intermediate-level radioactive liquid waste represents an issue of high concern.1–3 The compaction of these large volumes, that comprise thousands of cubic meters, is highly desirable from both an environmental and an economical point of view. Among the different fission products in nuclear power reactors, cesium-137 (137Cs) is of particular importance. In fact, this radioactive isotope has a half-life of roughly 30 years, and it is both a beta- and a gamma-emitter.4 As a result of its nuclear properties, 137Cs is the primary source of penetrating gamma radiation for decades after discharge of, e.g., spent nuclear fuel.5,6 Similar to the other alkali metals, cesium is water-soluble and prone to bioaccumulation in marine and freshwater ecosystems due to its capability of substituting sodium and potassium in living organisms 7–10. Ingestion of 137Cs leads to the accumulation of this radioactive isotope in soft tissues from the thyroid, lungs, breast, and bone marrow,11–14 resulting in a biological action similar to a continuous exposure to radiation from external sources. The ultimate effect of bioaccumulation of 137Cs in humans, in dependency to the amount ingested, is the development of radiation-induced malignancies,15–17 as well as mutagenesis in germinal cells.18,19 Many methods are currently available for decontamination of radioactive wastewater from nuclear fission products.20–27 However, most of the existing technologies, like co-precipitation, evaporation, reverse osmosis, and ion exchange, show strong disadvantages, with the main ones being low efficiencies, high production and operational costs, or limited reusability. Clay minerals (e.g., zeolite, bentonite, vermiculite, and montmorillonite) suffer from the competitive interactions of sodium and potassium with the available adsorption sites. The consequences are slow kinetics and/or poor selectivity.28,29 Furthermore, avoiding the production of toxic derivatives and residues from the decontamination process is of utmost importance.
In this work, we exhibit the potential of a new filter material, based on a blend of whey-protein fibrils and active charcoal,30 for the treatment of radioactive aqueous waste deriving from nuclear industry and other radiochemical activities. Previously, this filter membrane demonstrated to remove a wide variety of contaminants from wastewaters, under diverse chemical conditions.30–34 The surprisingly broad retention capacity of this material offers thus excellent prospects for the decontamination of radioactive aqueous waste deriving from nuclear power industry, radionuclide production, as well from nuclear medicine. In this study, we focused our attention in the treatment of wastewater containing 137Cs, since an effective removal of this problematic fission product can be considered as a benchmark for the successful application of the above-mentioned filtering material in the cleaning of low-to medium-level radioactive aqueous waste containing other fission products (e.g., 90Sr).
First, we synthesized the amyloids–carbon hybrid membranes as indicated in ref. 30. Then, preliminary tests on the performance of the filter material regarding the removal of 137Cs from radioactive samples in aqueous phase were conducted. The average 137Cs activity per unit volume of each test sample amounted to 2.5·105 Bq L−1. This is comparable to the maximum concentration of 137Cs in the seawater just off the accident site of the Fukushima Daichii Nuclear Power Plant one month after the accident, i.e., 105 Bq L−1.35 In an initial set of adsorption experiments, we investigated the interaction of trace amounts (i.e., carrier free) of ionic 137Cs with the protein-bearing filter membrane at zero surface coverage. A successive set of experiments included the addition of weighable amounts of natCsCl with admixtures of the radioactive tracer 137Cs, enabling us to explore the sorption capacity of this amyloid–carbon hybrid filtering material. The latter is a decisive parameter for a potential industrial application. Gamma-ray spectrometry was applied for measuring the activity of 137Cs before and after filtration of the contaminated water samples. Finally, the homogeneity of the distribution of the retained 137Cs in the filter material was investigated by autoradiography.
2. Experimental
2.1. Preparation of the hybrid membrane
Whey protein isolate (β-lactoglobulin) was purchased from Bipro @Agropure. The preparation of the amyloid fibrils was carried out without any further purification by heat denaturation of the 2 wt% monomer solution at pH 2 for 5 hours. Activated carbon (Norit carbon, particle diameter less than 60 mesh) was utilized. Hybrid composite membranes having 10 wt% amyloid fibrils were prepared by initial mixing of β-lactoglobulin solution, activated carbon and paper pulp in the ratio of 10
:
40
:
50 wt% respectively, as well as cellulose as supporting material. Water was removed from this mixture by vacuum filtration followed by a pressing and drying process similar to paper manufacturing. The obtained membranes (3 mm in thickness) were cropped to disks with a diameter of 50 mm and finally used to filter the radioactive water samples containing 137Cs. The characteristics of the membrane are found in Table 1.
Table 1 Characteristics of the membrane
Protein content |
10 wt% |
Activated carbon total surface area (BET) |
1150 m2 g−1 |
Thickness of membrane |
3 mm |
Membrane pore size |
0.7 μm |
2.2. Preparation of the aqueous carrier-free and carrier-added 137Cs-solutions
Aqueous samples containing tracer amounts of 137Cs were prepared by diluting an aliquote (100 μL) of a stock solution of 137CsCl to 50 mL with MilliQ-2 water. Each test solution was then acidified to a pH = 4 using 1 M HCl in order to mimic a corrosive environment. Aqueous samples containing macroscopic amounts of CsCl were obtained by preparation of a 10 ppm natCsCl (analytical grade EMSURE®, Merck KGaA) aqueous solution (50 mL in volume), to which 100 μL of the above-mentioned 137CsCl stock solution were added. It should be noted here that the addition of the 137Cs tracer does not change the overall concentration of the carrier-added cesium solution with a concentration of 10 ppm. The 137CsCl stock solution was obtained from the large-scale research facility Hotlab at Paul Scherrer Institute, Switzerland. All solutions were prepared using ultrapure water, obtained from the MilliQ-2 system water purifier (Millipore Corp., Bedford, USA). The mineral acid was of ACS grade, and procured from Sigma-Aldrich (HCl, 37%). Each test solution showed an average activity in the range of 10–12 kBq, i.e., an activity concentration of approx. 2.5⋅105 Bq L−1. The pH of each test solution was determined using pH indicator strips MColorpHast™ (pH 0–14). Aliquots of various volumes of the test solutions were used for filtration.
2.3. Filtration procedure
Experiments were conducted with the syringe-aided setup shown in Fig. 1a. The synthesized hybrid membrane filter was placed inside the filter holder (Fig. 1b and c). The edges of the screwcap of the adaptor were greased with silicone lubricant to ensure a leak-proof and tight fit. Before filtration of the radioactive samples, the membranes were conditioned with MilliQ-2 water (5 mL) acidified with HCl to a pH ≈ 4. After the pre-treatment of the filter, the radioactive test solutions were passed through the filtration cell by applying gentle pressure with the syringe at a flux of approx. 1 mL min−1.
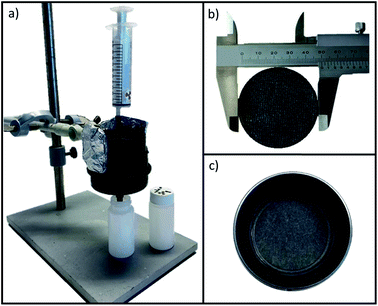 |
| Fig. 1 (a) Syringe-aided filtration setup for the removal of 137Cs from aqueous samples; (b) top view of filter disk (50 mm diameter); (c) top-down view of the filter holder containing the amyloid–carbon hybrid filter. | |
2.4. Gamma-ray spectroscopy
The monitoring and quantification of the amount of 137Cs in each sample was done by integrating the total number of counts in the main gamma-line of 137Cs at 662 keV. Gamma-spectroscopy measurements were performed with a Broad Energy Germanium (BEGe) detector (Canberra, Mirion Technologies, Inc.). All the solutions were measured in a defined geometry using HDPE scintillation vials. The energy calibration of the spectrometer was done with a certified 152Eu standard reference gamma-source from PTB (Physikalisch-Technische Bundesanstalt), Braunschweig, Germany. The efficiency calibration of the spectrometer was performed with a 133Ba liquid calibration source with a specific activity of 2 Bq μL−1. The determination of this specific activity itself was determined by using a certified source of 133Ba (PTB). All gamma-spectra were recorded and analyzed with the Genie 2000 Gamma Analysis software (Canberra, Mirion Technologies, Inc.).
2.5. Radiographic imaging
The homogeneity of the distribution of the retained 137Cs was investigated by autoradiography. A GE Typhoon FLA 7000 IP imaging plate scanner, equipped with a BAS-IP MS 2040 E super resolution film was used to obtain the autoradiographic images. The filters were placed over the film for 72 hours, and the data were analyzed with the ImageJ software (U. S. National Institutes of Health, Maryland, USA, https://imagej.nih.gov/ij).
2.6. Safety and protection measures
The assessment of the adverse effects upon exposure (by ingestion or inhalation) to materials containing pathological amyloid fibrils is still under study.36–38 At present, global safety guidelines and/or protocols for handling this type of material are not yet fully defined. However, there start to be converging opinions that the use of food-based amyloids, as in the present case, is safe for human consumption.39 Therefore, in our studies, we applied the same ALARA (As Low As Reasonably Achievable) safety concept that is applied when handling radioactive material. Synthesis, characterization, and experiments with the filters containing the amyloid fibrils and the radioactive material were conducted in a variable air volume fume hood, wearing protective nitrile gloves (EN ISO 374-1 and EN ISO 374-5 type), laboratory face masks (KN95 lab grade), and safety eyewear (EN 166, EN 169, and EN 170 standards). For further radiochemical safety, the dose rate (below the μSV h−1 level) was measured at the working distance using dose rate meters 6150AD® Automation und Messtechnik GmbH. The personnel was equipped with personal dosimeters for the detection of gamma, beta, and neutron radiation.
3. Results and discussion
The gamma-spectrometric measurements demonstrated that after a single filtration cycle the 137Cs content in 20 mL of radioactive water is reduced by a factor of approx. 340 to about 700 Bq L−1, with a decontamination efficiency of 99.7% (see Fig. 2a). According to the International Commission on Radiological Protection,40 the guidance level value of 137Cs in drinking water is 10 Bq L−1. Hence, two consecutive filtering cycles are sufficient to render an initial sample of radioactively contaminated water suitable for human consumption. To clarify the role played by the amyloid fibrils in the adsorption process, retention experiments were performed with the same typology and mesh-size of filter support deprived of the amyloid–carbon hybrid admixture. As shown in Fig. 2b, the retention of 137Cs without the amyloid–carbon hybrid filter is about 100 times less efficient, clearly indicating that the protein aggregates are the main contributors to the chemical binding and retention of Cs.
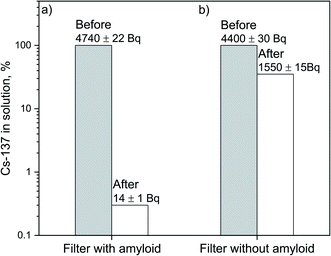 |
| Fig. 2 Activity of 137Cs in [Bq] before and after the filtration of a radioactive aqueous sample (volume = 20 mL) through (a) an amyloid–activated carbon hybrid membrane, (b) a quartz fibre membrane. | |
The uptake of cations by the filter material originates from a supramolecular interaction between the cation and the β-sheets of the β-lactoglobulin amyloid fibrils, stabilized by the presence of multiple amino-acid residues.32,41 As shown in previous studies, the strongest binding motif of the β-lactoglobulin protein sequence is the 121-cys residue containing the amyloidogenic fragment LACQCL.30,42,43
Radiographic imaging of the amyloid–carbon hybrid filter (Fig. 3) shows the spatial distribution of 137Cs as captured in the membrane. The homogenous distribution indicates no preferential flow paths of the liquid through the filtration unit. The observed darker spots in Fig. 3 are due to accumulation of activity caused by the rough nature of the filtering material itself. The retention capacity of the membrane was investigated by performing consecutive filtrations of carrier-added CsCl solutions (10 ppm) spiked with 137Cs for monitoring purposes, at a flux of approx. 1 mL min−1. Six consecutive filtration cycles of a total volume of 30 mL led to a total uptake of 102 μg of Cs, without reaching saturation (see Fig. 4). This indicates a specific sorption capacity of at least 36 μg of Cs per gram of filter material, at the applied conditions.
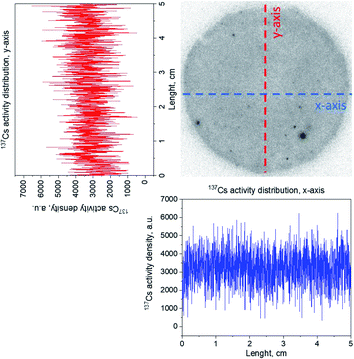 |
| Fig. 3 Radiographic image of the amyloid–carbon hybrid filter after uptake of 137Cs. The distribution of 137Cs along the x- and the y-axis is shown. | |
 |
| Fig. 4 Cumulative retention of macroscopic amounts of CsCl solution (10 ppm) spiked with 137Cs, during a consecutive series of filtrations (squares) using 2.8 g of filter material (10 wt% protein fibrils). Data fitting of the saturation-type plot is indicated (solid line); errors of data points are too small to be shown. | |
The fitting of the data points in Fig. 4 was performed by applying an asymptotic regression model, as described in ref. 44:
The method defines a limited growth, where y represents the cumulative retention of Cs by the filter, and x equals to the cumulative addition of Cs in the filter itself. The parameter a represents the asymptotic value with respect to y; b is the change in y when x passes from 0 to infinity; and c is the factor by which the difference (y–a) is reduced by increasing x. For the value of c the constrain 0 < c < 1 holds. In our experiments, for x = 0 (i.e., no Cs added to the filter), then it is obtained y = 0 (i.e., no Cs retained in the filter). This condition is true only if a = b. Hence, eqn (1) can be re-written as follows:
The fitting of the data with the Origin® 2018 software package, yields:
The curve asymptote a (i.e., the value at which the quantity of Cs retained by the filter reaches a plateau), represents the saturation limit of Cs for a 2.28 g filter containing 10 wt% of β-lactoglobulin fibrils. This gives an estimated maximum sorption capacity of 0.62 mg of Cs per gram of amyloid fibrils. For comparison, the Cs adsorption capacities of various materials at pH = 4 and at room temperature are listed in Table 2.
Table 2 Cesium sorption capacities of diverse filter materials at pH = 4 and T = 25 °C. Abbreviations: c.t. (contact time), a.c. (adsorption strongly affected by competing ions)
Adsorbent |
Adsorption capacity (mg g−1) |
Notes |
Ref. |
Potassium nickel hexacyanoferrate loaded-silica gels and chabazite. PAN = polyacrylonitrile. Hydrous manganese oxide. Hydrous stannic oxide. |
KNiFCa |
0.123 |
7 days c.t. |
45 |
Clinoptilolites |
1.31 |
48 h c.t. |
46 |
MnO2-PANb |
0.942 |
35 min c.t.; a.c. |
47 |
Cellulose + gypsum |
0.5 |
10 days c.t. |
48 |
Bentonite |
0.54 |
7 h c.t.; a.c. |
49 |
HMOc |
0.11 |
25 min c.t. |
50 |
HSOd |
0.12 |
20 min c.t. |
50 |
Amyloid fibrils |
0.62 |
∼6 min c.t. |
This work |
Based on these results, it is expected that radioactivity levels up to 0.2 TBq (i.e., in the order of 1011 decays per second) per kg of filter material can be extracted from radioactively contaminated water in a single filtration step. Different experimental settings of, e.g., contact time and pH of the filtered water may further influence the observed sorption capacity. Investigations on the reversibility of the retention process may define the maximum volume of liquid allowed per filter mass – a parameter that will greatly depend as well on the geometry of the filter arrangements optimized for industrial processing.
4. Conclusions
The studied hybrid membrane is an efficient filtration material for the decontamination of low-to medium-level radioactive wastewater from 137Cs. The application of this filter material for the removal of other fission products from radioactive aqueous waste is envisaged. Further investigations on the stability of the amyloid fibrils against radiation damage (i.e., degeneration of the protein-based binding sites) will help to understand whether this filter material can be efficiently applied in the treatment of high-level radioactive liquid wastes as well. Since the membranes are made completely from natural components, its controlled combustion to ashes further minimizes the final radioactive waste volume. These results may lead to an inexpensive and environmentally sustainable technology for the treatment of radioactive waste from various sources, including nuclear power plants.
Conflicts of interest
R. M. and S. B. are the inventors of a filed patent application related to the work presented here. All the other authors declare no competing interests.
Notes and references
- M. I. Ojovan, R. A. Robbins and M. Garamszeghy, MRS Adv., 2018, 3, 983–990 CrossRef CAS.
- R. Pusch, J. Kasbohm, S. Knutsson, T. Hoang-Minh and L. Nguyen-Thanh, J. Earth Sci. Geotech. Eng., 2019, 9, 237–272 Search PubMed.
- L. Abrahamsen-Mills and J. S. Small. Organic-containing nuclear wastes and national inventories across Europe, in The Microbiology of Nuclear Waste Disposal, Elsevier, 2021. pp. 1–20 Search PubMed.
- J. Magill and R. Dreher, AIP Conf. Proc., 2009, 1164, 100–106 CrossRef , http://www.nucleonica.net.
- S. Vaccaro, S. Tobin, A. Favalli, B. Grogan, P. Jansson, H. Liljenfeldt, V. Mozin, J. Hu, P. Schwalbach, A. Sjöland and H. Trellue, Nucl. Instrum. Methods Phys. Res., Sect. A, 2016, 833, 208–225 CrossRef CAS.
- J. Bruno and R. Ewing, Elements, 2006, 2, 343–349 CrossRef CAS.
- C. R. Bader and D. Bertrand, J. Physiol., 1984, 347, 611–631 CrossRef CAS PubMed.
- P. M. Hughes and A. D. Macknight, J. Physiol., 1977, 267, 113–136 CrossRef CAS PubMed.
- A. J. Levi, J. S. Mitcheson and J. C. Hancox, J. Physiol., 1996, 492, 1–9 CrossRef CAS PubMed.
- T. A. Baramanda, R. Bakri and H. Suseno, IOP Conf. Ser.: Mater. Sci. Eng., 2020, 902, 012056 CAS.
- Y. I. Bandazhevsky, Swiss Med. Wkly., 2003, 133, 35–36 Search PubMed.
- A. V. Nesterenko, V. B. Nesterenko and A. V. Yablokov, in Chernobyl: Consequences of the Catastrophe for People and the Environment, ed. A. V. Yablokov, V. B. Nesterenko, A. V. Nesterenko and J. D. Sherman-Nevinger, Annals of the New York Academy of Sciences, Boston, 2010, 26, p. 289 Search PubMed.
- E. R. Svendsen, I. E. Kolpakov, Y. I. Stepanova, V. Y. Vdovenko, M. V. Naboka, T. A. Mousseau, L. C. Mohr, D. G. Hoel and W. J. Karmaus, Environ. Health Perspect., 2010, 118, 720–725 CrossRef CAS PubMed.
- M. Izawa and H. Tsubota, J. Radiat. Res., 1962, 3, 120–129 CrossRef CAS PubMed.
- R. M. Rahal, M. E. Rocha, R. Freitas-Junior, R. da Silveira Corrêa, D. Rodrigues, E. Martins, L. R. Soares and J. C. Oliveira, Asian Pac. J. Cancer Prev., 2019, 20, 3811 CrossRef PubMed.
- Y. Shimizu, H. Kato, W. J. Schull and D. G. Hoel, Radiat. Res., 1992, 130, 249–266 CrossRef CAS PubMed.
- D. A. Pierce and D. L. Preston, Radiat. Res., 2000, 154, 178–186 CrossRef CAS PubMed.
- E. O. Alves Costa, D. de Melo e Silva, A. V. de Melo, F. Ribeiro Godoy and H. F. Nunes, Mutagenesis, 2011, 26, 651–655 CrossRef.
- A. D. a Cruz, D. D. a Silva, C. C. da Silva, R. J. Nelson, L. M. Ribeiro, E. R. Pedrosa, J. C. Jayme and M. P. Curado, Mutat. Res., Genet. Toxicol. Environ. Mutagen., 2008, 652, 175–179 CrossRef PubMed.
- X. Zhang, P. Gu and Y. Liu, Chemosphere, 2019, 215, 543–553 CrossRef CAS PubMed.
- M. Lin, I. Kajan, D. Schumann and A. Türler, J. Radioanal. Nucl. Chem., 2019, 322, 1857–1862 CrossRef CAS.
- D. Rana, T. Matsuura, M. Kassim and A. Ismail, Desalination, 2013, 321, 77–92 CrossRef CAS.
- M. Jiménez-Reyes, P. Almazán-Sánchez and M. Solache-Ríos, J. Environ. Radioact., 2021, 233, 106610–106622 CrossRef PubMed.
- X. Zhang and Y. Liu, Environ. Sci.: Nano, 2020, 7, 1008–1040 RSC.
- Z. X. Wu Y, W. YZ and M. H, Sep. Purif. Technol., 2017, 181, 76–84 CrossRef.
- O. Halevi, T. Chen, P. Lee, S. Magdassi and J. Hriljac, RSC Adv., 2020, 10, 5766–5776 RSC.
- H. Faghihian, M. Iravani, M. Moayed and M. G. Maragheh, Chem. Eng. J., 2013, 222, 41–48 CrossRef CAS.
- O. Ararema, F. Bouras and F. Arbaoui, Chem. Eng. J., 2011, 172, 230–236 CrossRef.
- E. H. Borai, R. Harjula and A. Paajanen, J. Hazard. Mater., 2009, 172, 416–422 CrossRef CAS PubMed.
- S. Bolisetty and R. Mezzenga, Nat. Nanotechnol., 2016, 11, 365–371 CrossRef CAS PubMed.
- S. Bolisetty, N. Reinhold, C. Zeder, M. Orozco and R. Mezzenga, Chem. Commun., 2017, 53, 5714–5717 RSC.
- M. Peydayesh, S. Bolisetty, T. Mohammadi and R. Mezzenga, Langmuir, 2019, 35, 4161–4170 CrossRef CAS PubMed.
- S. Bolisetty, N. Coray, A. Palika, G. Prenosil and R. Mezzenga, Environ. Sci.: Water Res. Technol., 2020, 6, 3249–3254 RSC.
- A. Palika, A. Armanious, A. Rahimi, C. Medaglia, M. Gasbarri, S. Handschin, A. Rossi, M. Pohl, I. Busnadiego, C. Gübeli and R. Anjanappa, Nat. Nanotechnol., 2021, 16, 918–925 CrossRef CAS PubMed.
- H. Kaeriyama, Fish. Oceanogr., 2017, 26, 99–103 CrossRef.
- V. Bellotti, M. Nuvolone, S. Giorgetti, L. Obici, G. Palladini, P. Russo, F. Lavatelli, V. Perfetti and G. Merlini, Ann. Med., 2007, 39, 200–207 CrossRef CAS PubMed.
- S. Tsukawaki, T. Murakami, K. Suzuki and Y. Nakazawa, Biomed. Mater., 2016, 11, 065010–065018 CrossRef PubMed.
- V. Bellotti and F. Chiti, Curr. Opin. Struct. Biol., 2008, 18, 771–779 CrossRef CAS PubMed.
- Y. Cao and R. Mezzenga, Adv. Colloid Interface Sci., 2019, 269, 334–356 CrossRef CAS PubMed.
- A. Wrixon, J. Radiol. Prot., 2008, 28, 161–168 CrossRef CAS PubMed.
- I. Javed, Y. Sun, J. Adamcik, B. Wang, A. Kakinen, E. H. Pilkington, F. Ding, R. Mezzenga, T. P. Davis and P. C. Ke, Biomacromolecules, 2017, 18, 4316–4322 CrossRef CAS PubMed.
- J. H. Viles, Coord. Chem. Rev., 2012, 256, 2271–2284 CrossRef CAS.
- J. Park, W. Lee, G. Lee, H. Choi, M. Kim, W. Kim, S. Na and J. Park, J. Electrochem. Soc., 2019, 166, B1497 CrossRef CAS.
- W. L. Stevens, Biometrics, 1951, 7, 247 CrossRef.
- H. Mimura, M. Kimura, K. Akiba and Y. Onodera, Solvent Extr. Ion Exch., 1999, 17, 403–417 CrossRef CAS.
- A. Abusafa and H. Yücel, Sep. Purif. Technol., 2002, 28, 103–116 CrossRef CAS.
- A. Nilchi, R. Saberi, S. R. Garmarodi and A. Bagheri, Appl. Radiat. Isot., 2012, 70, 369–374 CrossRef CAS PubMed.
- M. Y. Miah, K. Volchek, W. Kuang and F. H. Tezel, J. Hazard. Mater., 2010, 183, 712–717 CrossRef CAS PubMed.
- A. F. Seliman, Y. F. Lasheen, M. A. E. Youssief, M. M. Abo-Aly and F. A. Shehata, J. Radioanal. Nucl. Chem., 2014, 300, 969–979 CrossRef CAS.
- S. P. Mishra, Sep. Purif. Technol., 2007, 54, 10–17 CrossRef CAS.
Footnote |
† These authors contributed equally to this work. |
|
This journal is © The Royal Society of Chemistry 2021 |
Click here to see how this site uses Cookies. View our privacy policy here.