DOI:
10.1039/D1RA06404E
(Review Article)
RSC Adv., 2021,
11, 34572-34588
Nanotechnology in cardiac stem cell therapy: cell modulation, imaging and gene delivery
Received
24th August 2021
, Accepted 4th October 2021
First published on 26th October 2021
Abstract
The wide arena of applications opened by nanotechnology is multidimensional. It is already been proven that its prominence can continuously influence human life. The role of stem cells in curing degenerative diseases is another major area of research. Cardiovascular diseases are one of the major causes of death globally. Nanotechnology-assisted stem cell therapy could be used to tackle the challenges faced in the management of cardiovascular diseases. In spite of the positive indications and proven potential of stem cells to differentiate into cardiomyocytes for cardiac repair and regeneration during myocardial infarction, this therapeutic approach still remains in its infancy due to several factors such as non-specificity of injected cells, insignificant survival rate, and low cell retention. Attempts to improve stem cell therapy using nanoparticles have shown some interest among researchers. This review focuses on the major hurdles associated with cardiac stem cell therapy and the role of nanoparticles to overcome the major challenges in this field, including cell modulation, imaging, tracking and gene delivery.
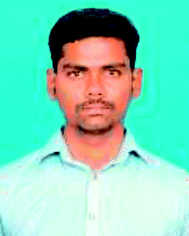 Sarathkumar E | Sarathkumar Elangovan is a PhD student at Division of Biophotonics and Imaging, Sree Chitra Tirunal Institute for Medical Sciences and Technology, Trivandrum. He got his B.Tech from Anna University, Chennai and obtained his M.Tech in Biotechnology from Motilal Nehru National Institute of Technology, Allahabad. His area of research interest includes exploring the role of nanomaterials in biomedical engineering and development of point of care biosensors. Currently, he works on interdisciplinary field, and it is involved in development of multifunctional nanoprobes for cardiac stem cell therapy. |
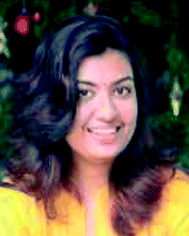 Marina Victor | Marina Victor was a research student at the Division of Biophotonics and Imaging, Sree Chitra Tirunal Institute for Medical Sciences and Technology, Trivandrum. She did BS MS from Indian Institute of Science Education and Research, Pune. Her research interests include cardiac stem cell therapy, nanomaterials and their biomedical applications. |
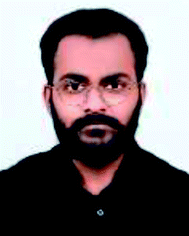 Jaivardhan A. Menon | Jaivardhan A Menon is a medical graduate doing his internship in Sree Narayana Institute of Medical Sciences, Kochi. He has wide research interest spanning from cardiac stem cell therapy, cell senescence, autophagy, mitophagy and, is an aspiring surgeon. |
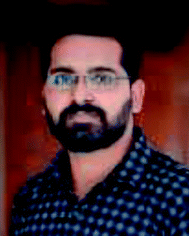 Kunnumpurathu Jibin | Kunnumpurathu Jibin is currently a Researcher in Division of Biophotonics and Imaging, Sree Chitra Tirunal Institute for Medical Sciences and Technology (SCTIMST). He received his PhD from SCTIMST in 2020. His research work is mainly focused on graphene plasmonic nanostructures for theranostic applications, biomarker sensing for point-of-care diagnostics and personalized nanomedicine. |
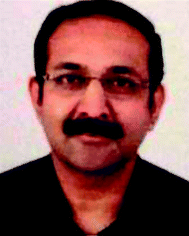 Suresh Padmini | Suresh Padmini is Professor and Head of the Department of Medicine and Diabetology, S. N. Medical Collage, Ernakulam and the Director of SR Medicare Centre, Koratty, India. He is a Member of American Endocrine Society and holds Diploma in Diabetology (Liverpool) with broad interest in medical sciences ranging from diabetology, intensive care medicine and cardiac functions of diabetic patients. |
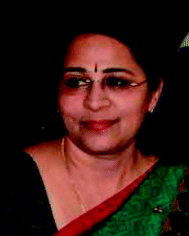 Ramapurath S. Jayasree | Ramapurath S. Jayasree is heading the division of Biophotonics and Imaging of SCTIMST at its Biomedical Technology Wing. She is an experienced researcher with her signature imprinted in the fields of biophotonics and bionanophotonics, with passion to develop nanoprobes for biosensing, bioimaging, photodynamic and photothermal therapy. Her contributions are mostly in the fields of cancer research, brain imaging and cardiac stem cell therapy. She has also contributed significantly in the use of laser based techniques and spectroscopy for biomedical applications. |
1. Introduction
The World Health Organization (WHO) reports that around 17.9 million people lose their lives every year from cardiovascular diseases (CVDs). This accounts for 31% of all deaths, globally. Cardiovascular diseases are disorders of the heart and the coronary blood vessels. This comprises coronary heart disease, cerebrovascular disease, rheumatic heart disease and other conditions. But myocardial infarct (MI) is the major reason for life loss. The risk of CVD is generally demonstrated by monitoring levels of blood pressure, glucose, lipids along with overweight and obesity (WHO). Heart transplants and LVDs (left ventricle assist devices) contribute to the solutions in some of the cases. However, regenerative cell therapies could be a better option for patients who suffer MI, heart failure, or congenital heart disease. This is because of the limited capability of the heart to regenerate endogenously, and the lack of sufficient cells to repopulate the myocardium, post-injury.1
In the current scenario, an injured myocardium cannot be reversed, and the few cardiomyocytes left in the injured site possess insufficient regeneration capacity for repairing the myocardium. Considering this factor, the focus of research has moved to the development of an efficient and promising approach to treat MI, which would address questions such as, how to prevent the loss of cardiomyocytes, how to improve their regenerative ability and how to reduce the size of the infarct.2
A schematic representation of various advancements in translational cardiac regenerative therapies is shown in Fig. 1.3 Particularly, stem cell therapy is one such promising approach, not only for MI, but for a large number of diseases.4,5 Since the last decade, lots of researchers have placed their efforts in overcoming various barriers in stem cells therapy and finding the solution for these challenges. Both animal studies and clinical studies have proved the potential of stem cells to differentiate into cardiomyocytes, indicating the possibility of cardiac repair and regeneration during MI. More recently, studies have offered data to show that in vitro manipulation of MSCs can be considered for therapeutic management for cardio-protection. By genetically engineering MSCs before in vivo transplantation, it is expected that the survival rate and differentiation potential of the cells could be improved during ischemic conditions.
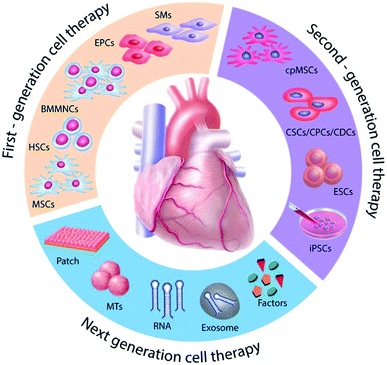 |
| Fig. 1 Schematic representation of evolution in cardiac regenerative therapies.3 | |
In this context, the support of nanotechnology for stem cell treatment of cardiovascular diseases has a great scope with many advantages. It is high time to utilize the knowledge and application of nanoscience in medicine for the improved treatment of these diseases.6 Thus, nanotechnology may provide a safe and effective platform for controlled drug delivery for a variety of active ingredients, imaging and tracking of the transplanted stem cells, a diagnostic tool for cardiovascular diseases, gene delivery agents, etc.7 The use of iron oxide nanoparticles for magnetically-controlled drug targeting is one of the earliest studies for the use of nanoparticles in cardiac stem cell therapy.8 In this study, a bioactive molecule having magnetic property is tailored into an injectable nanoparticle complex, which is guided to and retained in a specific target site (for the scope of this discussion, in the myocardial infarct) with the aid of applied magnetic fields. The use of nanomaterials in improving the prognosis of MI is being extensively studied recently, with much significance falling on gold, silica and polymer-based nanoparticles as well as exosomes and hydrogels. The following sections discuss these aspects in detail.
2. Pathophysiology of MI
In a pathophysiological expression, MI is a condition in which there occurs cardiomyocyte cell death as a result of an ischemic insult. But in terms of a clinical perspective, it is a condition wherein rupture of a vulnerable plaque causes thrombotic occlusion of a coronary vessel (Fig. 2).9,10
 |
| Fig. 2 Schematic of acute myocardial infarction. (A) Infarcted myocardium. (B) Blocked artery due to plaque formation (source: National Heart, Lung, and Blood Institute; National Institutes of Health; U.S. Department of Health and Human Services).11 | |
In the early 1970s, by the definition of WHO, the presence of any two of the following three characteristics was considered as MI.12,13 They are (i) symptoms of acute chest pain (ischemia), (ii) appearance of Q waves in electrocardiogram (ECG) and (iii) enhancement in the level of enzymes like creatine kinase (CK), creatine kinase myocardial band (CK-MB), aspartate aminotransferase (AST) and lactate dehydrogenase (LDH), in the blood. But by 1999, the European Society of Cardiology and the American College of Cardiology Committee jointly announced a new definition for MI, stating the importance of sensitive and serological biomarkers, and introduced cardiac troponins (cTn) as the gold standards for the diagnosis of AMI.14 However, it is noted that even if the sudden elevations in circulating troponin levels are generally correlated with myocardial injury, they are not always specific markers of ischemic cardiomyocyte death.9 MI is always associated with an imbalance between the demand and supply of oxygen to the cardiac tissues. At rest, even a major luminal narrowing of the order of more than 75% does not cause inadequate oxygenation so as to lead to tissue ischemia. But when there is an increase in demand, the flow restriction precludes an increase in oxygen supply, resulting in ischemia and causing angina pectoris.15 Immediate functional depression during MI is due to the generation of inorganic phosphates and the binding of calcium to contractile proteins.16,17 Even though the restoration of blood supply within 4–5 min after coronary occlusions can reverse functional depression in an ischemic heart, the generation of reactive oxygen species and perturbations in calcium homeostasis results in severe dysfunction. For better prognosis, quick identification of AMI is mandatory. The recent concept of a diagnosis of AMI focuses on the 12-lead ECG and the assessment of early cardiac biomarkers. This is mainly due to the inadequacy of ECG itself to diagnose AMI. The majorly reported diagnostic cardiac markers are classified into three types, namely inflammatory markers, plaque destabilization markers and myocardial necrosis markers and are listed in Table 1.18 Before the scientific advances, markers such as creatine kinases, aspartate amino transferases were used as a cardiac biomarkers. However, owing to their low specificity to cardiac disease, these markers became outdated. After the revolution in immuno-assays, myocardial necrosis markers such as cardiac troponins and myoglobin are extensively used as cardiac disease diagnostic markers. Whereas, plaque destabilization and inflammatory markers are emerging as a new era of cardiac markers to identify various cardiac impairments.18,19
Table 1 A list of cardiac markers
Inflammatory markers |
Plaque destabilization markers |
Myocardial necrosis markers |
• C-reactive protein (CRP) |
• Myeloperoxidase (MPO) |
• Troponin |
• Pentraxin 3 (PTX3) |
• Pregnancy-associated plasma protein A (PAPPA) |
• Myoglobin |
• Interleukin (IL)6 |
• A soluble cluster of differentiation 40 ligands (sCD40L) |
• CK and CKMB |
• TNFα |
• Heart fatty acid-binding protein (HFABP) |
• B-type natriuretic peptide (BNP) |
• Ischemia modified albumin (IMA) |
• Growth differentiation factor 15 (GDF15) |
• Copeptin |
3. Cardiac regenerative biology
Regenerating damaged hearts in patients with heart diseases is a major challenge. Recent reports on cell-based therapies suggest improvement in cardiac function thus mediates cardiac regeneration.5 Three different principles can be considered as the underlying factors for cardiac regenerative biology.
3.1. The existence of cardiac progenitor cells in the embryonic heart
The early developmental studies of Moretti et al.20 confirmed the existence of cardiac progenitor cells in the human embryonic heart. They have shown the development of primary and secondary heart fields from a subpopulation of cells that are of mesodermal origin. Critical evaluation of different steps in the formation of the heart, especially various initiator and terminator signals are of vital importance for development of various cardiac regenerative therapies.
3.2. The renewal of cardiomyocytes in mammals after childbirth
Earlier it was believed that cardiomyocyte proliferation ceases immediately after childbirth. But, in 1990s, Kajstura et al.21 and Quaini et al.22 identified crucial results, which proved that the cells of the heart could undergo cellular division even after birth. The C14-based experimental approach of Bergmann et al.23,24 showed that approximately 1.5% of new cardiomyocytes are formed per year untill the age of 25, which decreases substantially in the latter half of life. Using the same method, Anversa and colleagues21 found a higher turnover of cardiomyocytes in humans. But later studies showed similar results to that of Bergmann et al. These results confirm that there is definitely cardiomyocyte proliferation after childbirth, but at a very slow rate.
3.3. Myocardial regeneration in certain vertebrates following injury
The remarkable regenerative potential of cardiac tissues of newts and zebra fish made researchers look into the regenerative potential of the human heart. Newts and zebra fish survive by cardiac regeneration even after heart apex amputation. In similar lines, a mouse model was developed with about 50% damage to the heart tissue and it was replaced by healthy tissue during the fetal heart development stage.25,26 Furthermore, Sadek and colleagues showed that one-day-old neonatal mouse heart regenerates after removal of 15% of its ventricle at the apex.27,28 However, the regeneration capacity of the myocardium is rapidly lost by 7 days after the birth. Afterwards, the heart develops fibrotic scars similar to the response observed in adult mice and humans.
4. Cardiac stem cell therapy
Triggered by the above findings, scientists have offered various cell-based methods to enhance the regenerative ability of the heart. Stem cell-based cardiac therapy was one among the many. Stem cells are capable of self-renewal, and differentiate into almost any other cells or tissue present in the body and have received considerable attention in the area of regenerative medicine. Due to the limited regenerative ability of cardiomyocytes, stem cells can directly replace the damaged cardiomyocytes or induces cardio myogenesis by chemokine signaling mechanism.29 Though different stem cell types, from various tissue sources, have been identified for cell therapy, mesenchymal stem cells (MSCs) are found to be better candidates for cardiac regeneration, based on their easiness in isolation, improved self-renewal capacity, better immuno-modulatory properties, broad-spectrum release of trophic factors and their capability to differentiate into mesodermal and non-mesodermal tissues.30 In 2001, Orlic and colleagues first reported the regenerative potential of intramyocardially injected bone marrow-derived cells (BMCs) in repairing the infarcted myocardium.31 Since then, various stem cell-based therapeutic approaches have been proposed for cardio vascular diseases (CVD) in preclinical and clinical research. Various challenges faced by the current stem cell therapy along with their potential milestones achieved so far in CVD treatment are explored in a subsequent section.
5. Challenges in stem cell therapy
Though stem cell therapy is projected as the simple solution for the treatment of MI, the reality of the situation is more complex than it seems. The researchers must go through numerous challenges and hurdles to get the optimum strategy for the treatment of MI. Because of the same reason, the major research on stem cell therapy for cardiovascular diseases is to address these challenges. The major challenges include (1) identifying the right source of stem cell, (2) increase homing of stem cells to injured hearts and (3) the survival of homed cells under hypoxic conditions. These points are discussed in detail in the following sections.
5.1. Identifying the right source of stem cells
Identification and selection of the best-suited stem cell are one of the most challenging tasks for regeneration therapy. Generally used cells include bone marrow mononuclear cells, bone marrow-derived mesenchymal stem cells (BM-MSCs), resident or endogenous cardiac stem cells, endothelial progenitor cells and induced pluripotent stem cells (iPSCs). These cell types have been extensively studied for their regenerative capacity of lost myocardium. Various results of clinical trials have shown heterogeneous behavior in terms of efficacy. The reports of poor survival, proliferation, engraftment and differentiation of the transplanted cells have somehow damped the enthusiasm for cell therapies for MI.32
Embryonic stem cells being capable of indefinite division are potentially the best candidate for stem cell therapy. The ability to electrically integrate with the heart muscle makes the ESC-derived cardiomyocytes (ESC-CMs) superior to other cell types. Xue et al. have successfully demonstrated this in a swine model of an atrioventricular block, the transplanted human ESC-CMs showed electrical coupling and a reversal of the block.33 The ESC-cardiomyocytes developed by Chong et al. were also successful to engraft and regenerate the injured myocardium in a large animal model of MI.34 Though these cells have been proven to possess significant regenerative capacity followed by MI even in primate models, the use of embryonic stem cells are limited due to their tumorogenic and immunogenic potential, genetic instability, ethical concerns, etc.34
The next choice of stem cell therapy for the treatment of MI is the cells derived from bone marrow. These include the bone marrow-derived mononuclear cells, hematopoietic stem cells and the bone marrow-derived mesenchymal stem cells. In 2015, Dutta et al. showed that MI can trigger the activation of hematopoietic stem cells and their progenitor, which in turn facilitates the repair of MI. However, the mechanism associated with this activation is still unclear.35 In a 2009 study, which compared the effect of various stem cells to escape the first-pass effect and repopulate in the heart, bone marrow-derived mononuclear cells showed better results due to their smaller size and surface markers.36 Though numerous research groups are working on the efficacy of bone marrow-derived stem cells, many studies failed to show any benefit from BMCs and hence from the available clinical trial results, it is extremely difficult to draw conclusions on the efficacy of BMCs.37
MSCs have the capacity for self-renewal and can differentiate into the mesenchymal lineages, including skeletal myoblasts, chondrocytes, and adipose tissue.38 These cells are not restricted to just bone marrow. They have diverse distribution and can also be isolated from adipose tissue, cord blood, etc. MScs are hypoimmunogenic, often lacking major histocompatibility complex IIMHC-II and costimulatory molecule expression. This makes it one of the most used stem cell sources as it opens the possibility of non-autologous transplantation of these cells in patients.38,39 Owing to the presence of CD49 on the surface, the umbilical cord-derived mesenchymal stem cells have been shown to pass the pulmonary capillaries.36 It is postulated that MSCs can contribute to neo-vascularization and cardiomyocyte protection, mainly through the activation of paracrine factors.40 Hence these cells not only differentiate into cardiomyocytes but also stimulate the inherent cells to differentiate and take part in cardiac regeneration.
Induced pluripotent cells are the most recent candidates for stem cell therapy. Introduced by Yamanaka in 2006, these cells have the same potential of ESCs to regenerate but with less ethical concerns.41 The iPSCs differentiate into cardiomyocytes, endothelial cells, and smooth muscle cells in vitro. iPSCs have been shown to differentiate into the cardiac phenotype when they were injected into the infarcted heart of mice.42,43 However, as they inherit ESC-like properties, they turn out to be tumorigenic. To overcome the problem of tumorigenesis, Martens and colleagues differentiated iPSCs into cardiomyocytes in vitro before the transplantation into the infarcted heart. These cells successfully improved cardiac functions, due to significant localization to the host myocardium.44 In another study by Christoforou et al. cardiac cells derived from iPSCs were even used for the creation of biosynthetic tissues.45 The research is now focused on enhancing the efficacy of iPSC-derived transplants to improve their engraftment, survival, and functionality.46 However, before considering the clinical translation, the in vivo safety as well as the functionality of these cells are needed to be guaranteed.
Endogenous cardiac stem cells, though seen in very small numbers, can be potential sources of stem cells for cardiac regeneration. Until recently, researchers had the impression that the cardiac tissue is terminally differentiated and hence not capable of regeneration. Recent research has proven the existence of resident cardiac progenitor cells as well as cardiac stem cells (CSCs) in the adult rat (and human) heart.47 But the studies also add that the turnover of myocytes is very low, which might not be good enough to repopulate an injured heart.48 But CSCs, even from a small myocardial biopsy can be expanded to many folds in vitro. CSC can be good candidates for treating MI because of its cardiac commitment and ability to undergo cardiomyogenic and angiogenic differentiation, uniformly. However, unlike MSCs, these cells seem to express the overlapping surface marker expression, which makes their isolation difficult. There are enormous studies all over the world using these cells, but among them, Stem Cell Infusion in Patients with Ischemic Cardiomyopathy (SCIPIO) and Cardiosphere-derived aUtologous stem CElls to reverse ventricUlar dySfunction (CADUCEUS) study has gained a lot of attention. SCIPIO is the first-in-human, randomized, open-label trial of autologous c-kit+ CSCs in patients with heart failure due to ischemic heart disease (IHD)undergoing coronary artery bypass graft (CABG).49 On the other hand, CADUCEUS study utilized cardiosphere-derived cells to deliver into the randomly selected patients after myocardial infarction and the result showed great improvement in cardiac tissue recovery.50 The results of these trials are highly promising and have proven that CSCs can be clonally-expanded and that these cells could be successfully administered by intracoronary injection to patients with prior MI.
The sources of stem cells available for therapy are numerous, treatment using them became complicated due to the practical and technical difficulties such as isolation of these cells, preconditioning of stem cells, choosing the appropriate delivery route, and dosages. Another major challenge in stem cell therapy for cardiac diseases is controlled differentiation of stem cells into cardiomyocytes and identification of differentiated cardiomyocytes. These can be performed by characterizing the genotypic and phenotypic markers of differentiated cardiomyocytes. The most commonly utilized cardiomyocyte-specific markers are troponin T2 (TNNT2), myosin light chain 7 (MYL7) and troponin I3 (TNNI3). The presence of cardiac progenitor cells in the early stages of cardiac differentiation can be detected by ISL1 and NKX2-5 markers.51 Further, functional cardiomyocytes can be distinguished by measuring their activity in electrical conductance, ionic current, cell surface proteins, cell morphology etc.52 Even if we could overcome the above difficulties and successfully administer the cells to the patient, there arises another major issue of proper homing of stem cells to an injured heart.
5.2. Increase homing of stem cells to the injured heart
The migration of SCs from endogenous and exogenous sources through the blood or tissue to a destination where they undergo differentiation, replace or repair injured tissue is generally termed as homing. An MI is generally associated with the transient increase in levels of cardiac cytokines such as MCP-3, GRO-1 and SDF-1. These factors generally act as chemoattractants for stem cells for active homing. But unfortunately, the expression of these factors persists only for a limited period of time. SDF-1, the most widely studied factor loses its expression in 1 week, while MCP-3 can be detected for 10 days post-MI. Granulocyte colony-stimulating factor (G-CSF) is clinically used for stem cell homing. G-CSF is sometimes used in conjunction with other factors such as granulocyte-macrophage colony-stimulating factor, stem cell factor, fms-like tyrosine kinase (Flt)-3 ligand, and interleukin-1, -3, -6, -7, -8, -11, and -12.53 Recently, Yamada et al. showed that S1P–S1PR2 axis can mediate homing of multilineage-differentiating stress enduring (Muse) cells to a damaged heart.54 In this study, Muse cells showed preferential homing of the cells to the post-infarct heart at 3 days and 2 weeks. About 14.5% of GFP (green fluorescent protein)-Muse cells were estimated to be engrafted into the heart at 3 days. The pharmacological and genetic evaluation confirmed the migration and homing of the Muse cells. Monsanto et al. successfully developed “Cardio Clusters”, which are three-dimensional microenvironments with three distinct types of stem cells isolated from the human heart. These cardio-clusters are delivered as an intramyocardial injection into the infarcted myocardium and have been shown to provide better homing and engraftment to injury sites.55 Gottipati et al. demonstrated that a gelatin polymer coating on stem cells can increase stem cell retention and homing to myocardial infarct in comparison to uncoated cells.56 Very recently a group from Egypt has reported that BMSCs preconditioned with sodium hydrosulfide (NaHS) in vitro showed enhanced stem cell homing and improved left ventricular function, proliferation and differentiation. Higher manifestation of the cardiac expression of GATA-4 and myocyte enhancer factor 2 confirmed this.57
Furthermore, many studies have reported the accumulation of platelets on injured myocardium due to their inflammatory responses. Platelets are tiny, circular anucleated cells that are circulating throughout the bloodstream in the body. Apart from the primary function of these platelets in hemostasis, they also play a crucial role in immune-modulatory actions that lead to the wound healing process.58 Many groups of researchers have been extensively studying the role of platelets as a targeting delivery vehicle for treating several kinds of diseases such as tumor and bacterial infections. Tang et al. adopted this natural homing ability of platelets to target the stem cells into an injured myocardium. They intravenously delivered platelet nanovesicles fused with CSCs into rats and pigs and observed a better homing of stem cells in the infarcted myocardium. They confirmed that the modified stem cell expresses both stem cell and platelet-specific surface markers, and increases cell migration and engraftment towards the injured site.59 In another study, the same group reported the antibody-armed platelets for redirecting the endogenous CD-34-positive stem cells into the infarcted myocardium. The results indicated that the accumulation of platelets-CD-34 positive stem cells over the infarcted myocardium following intravenous injection of CD-34 antibody-linked platelets. The above studies suggested that platelets might be a promising candidate for homing of stem cells into an injured myocardium and other tissues as well.60
5.3. The survival of homed cells under hypoxic condition
Once the stem cells are homed and engrafted to the injury site, the next step is to keep these cells alive and prevent them from getting washed off. It is observed that a large number of engrafted cells die off within four days after being grafted into injured hearts.61,62 In addition, the cells are incapable of survival during repeated bouts of ischemia. Apart from ischemia, endogenous and environmental factors also contribute to cell death. Ischemia-reperfusion, inflammatory response, and pro-apoptotic factors are major ones. Hence, cytoprotection for at least one-week post-engraftment is critical for efficient stem cell therapy. In 2006, Tang et al. showed that vector modification of MSCs with hypoxia-regulated heme oxygenase-1 (HO-1) enhanced the tolerance level of engrafted MSCs to cardiac injury in vitro and also improved their viability in ischemic hearts.63 They observed a five-fold increase in the survival of MSC MSCHO-1 after seven days of implantation, compared to the control group.64 Recently, a group from China reported the exceptional role of harsh environment-induced exosomes in mediating communication and cell survival during myocardial injury. In this study, they use the cardiomyocytes-conditioned medium and cardiac exosomes obtained from H2O2-treated cardiomyocyte culture medium under oxidative stress to mimic the environment that is encountered by the transplanted MSCs.65 Scientists were also successful in the development of an injectable hydrogel-based system that can release oxygen continuously to the injury site for about 4 weeks.66 Overexpression of sirtuin-3, a molecule involved in oxidative stress and life span, is also shown to improve the survival rate of aged mesenchymal cells after transplantation to the damaged heart. The protective effect of sirtuin-3 on aged-hMSCs against oxidative stress was by the positive regulation of antioxidant enzymes such as manganese-superoxide dismutase and catalase by inducing the expression of forkhead box O3a in the nucleus.67 Atorvastatin (ATV) may show a promising role in supporting the survival of MSC after MI, which leads to adverse conditions such as inflammatory reactions, oxidative damage and hypoxia stress at the site of tissue damage. But the efficiency of the molecule was largely reduced due to its limited systemic availability caused by its poor insolubility in aqueous environments. To address this limitation, Yao et al. developed a nanoparticle-engineered ATV that ensured its maximum systemic availability, for a prolonged period and to support MSC survival in the injury site.68 Long back, Pons et al. have reported the long-term culture of MSCs and showed the expression of cellular stress markers such as p16INK, p21 and p19ARF. This can reduce the survival of mesenchymal stem cells in an injury site. In order to overcome this issue, they co-injected MSCs with VEGF to MI hearts. This increased cell engraftment and cardiac function than that injected with MSCs or VEGF alone.69 In addition to VEGF, secreted frizzled-related protein 2 (Sfrp2) also shown to mediate MSC survival in MI.70 In another approach, blocking Nox2 enhanced the anti-apoptotic and anti-aging ability of BMSCs to oxidative stress, and improved therapeutic efficacy in MI.71
6. Nanotechnology in cardiac stem cell therapy
Nanotechnology has been rapidly advancing during the past decades in various fields. Due to the unique biological and physiochemical characteristics of nanomaterials, they have made tremendous contributions in the field of medical science. Nanomaterials have been widely used as tools for drug delivery, therapy, gene delivery, imaging and cell tracking. Nanotechnology-mediated stem cell therapy for cardiac diseases got considerable attention from researchers due to its superior properties at the nanoscale, ease of modification/functionalization, possibility to tune their properties, etc. The commonly employed nanomaterials include metallic nanoparticles,72,73 polymeric nanoparticles,74–76 liposomes,77,78 graphene and carbon-based nanoparticles.79,80 In the case of cardiac stem cell therapy, nanoparticles can even modulate the microenvironment and thus can control stem cell behaviors such as self-renewal or differentiation.81–84 There are various reports on applications of nanomaterials in cardiac stem cell therapy along with different stem cells (Table 2). They are also shown to significantly increase cell retention at the injury site; and thus enhancing cardiac repair due to the increased number of available stem cells.85 There are also reports of nanomaterials that can be beneficial for cardiac tissue engineering as well. Herein, we will look into the applications of nanomaterials in various aspects of cardiac stem cell therapy (Fig. 3).
Table 2 Application of various nanomaterials in cardiac stem cell therapya
Stem cell |
Delivery route |
Nanoparticle (NP) |
Animal model |
Targeting |
Applications |
Ref. |
Abbreviations: hMSCs (human mesenchymal stem cells), CREKA (Cys-Arg-Glu-Lys-Ala), ADSCs (adipose-derived stem cells), hiPSC-CMs (human-induced pluripotent stem cells derived cardiomyocytes), iPSC-CM (induced pluripotent stem cells derived cardiomyocytes), BADSCs (brown adipose derived stem cells), BM-MSCs (bone marrow derived mesenchymal stem cells), hCDCs (human cardiosphere derived cells), CD-CSCs (cardiosphere derived cardiac stem cells), CSCs (cardiac stem cells), PLGA-mPEG (poly (lactide-co-glycolide)-monomethoxy(polyethylene glycol)), IM (intramyocardial), IV(intravenous), EC (endocardial), IC (intracoronary), HIF1-α (hypoxia-inducible factor 1-α), HGF (hepatocyte growth factor), PEI (polyethyleneimine), MRI (magnetic resonance imaging), CXCR4 (C-X-C chemokine receptor type 4), SDF1 (stromal cell-derived factor 1), DNA (deoxyribonucleic acid), GO (graphene oxide), ROS (reactive oxygen species). |
hiPSC-CMs |
Intramyocardial |
PLGA |
Mice |
— |
Encapsulation of hiPSC-CMs in microparticles increases cell survival and retention |
86 |
ADSCs |
Intramyocardial |
PLGA-mPEG |
Rat |
— |
Melatonin–NP complex increases the survival rate of transplanted stem cells |
87 |
ADSCs |
Intravenous |
Simvastatin conjugated PLGA nanoparticles |
Mice |
— |
Nanomaterial conjugate utilized to increase cardiac activity |
88 |
BM-MSCs |
Intravenous and intramyocardial |
SPION and 1,1′-dioctadecyl-3,3,3′,3′-tetramethylindodicarbocyanine, 4-chlorobenzenesulfonate salt (DiD) |
Rat |
— |
IM group showed an increase in cardiac function compared to the IV group |
89 |
CDC |
Intramyocardial |
Nano-structured PEG-scaffolds |
Rat |
— |
Nanostructured PEG surface mimics native myocardial matrix and supports transplanted cell activity |
90 |
BM-MSCs |
Intramyocardial |
Iron oxide |
Rat |
Connexin 43 |
Co-culturing of iron oxide NP harboring H9C2 with MSC showed an increase in cardiac function |
91 |
BADSCs |
Intramyocardial |
Fullerenol/alginate hydrogel |
Rat |
— |
Hydrogel increases the survival limit of transplanted cells by protecting its oxidative stress damage |
92 |
Cardiomyocytes |
Intravenous |
Chlorin e6, luminol, and PEG conjugate |
Mice |
ROS |
ROS specific self -assembly fluorescent nanomaterials used to target the infarcted myocardium |
93 |
BM-MSCs |
Intramyocardial |
Iron oxide |
Rat |
— |
Stem cell retention increases for several weeks, the process tracked by iron oxide NP |
94 |
BM-MSCs |
Retrograde coronary venous infusion |
Iron oxide |
Rat |
— |
2.73- to 2.87-fold increase in cell retention compared to the control group. NP facilitated cell tracking |
95 |
hCDCs |
Intracoronary |
Ferumoxytol nanoparticles |
Rat |
— |
Cell retention achieved by magnetic targeting |
96 |
hMSCs |
Intramyocardial |
Silica-iron oxide |
Mice |
— |
Nano system facilitates imaging, drug delivery and magnetic manipulation |
97 |
MSCs |
Intracoronary |
SPION |
Rat |
Fibrin |
Cell homing achieved by CREKA-fibrin directed migration |
98 |
BM-MSCs |
Intramyocardial |
Europium (Eu) |
Rat |
Collagen matrix |
Implanting of cells in a collagen matrix increases cell retention. Eu NP is used for cell tracking |
99 |
Murine embryonic stem cells |
Intramyocardial |
SPION-luciferase |
Rat |
— |
Dual model bioluminescence and magnetic resonance imaging utilized to better monitoring of cells |
100 |
MSCs |
Intravenous |
SPION-labeled hMSC |
Mice |
— |
Magnetic particles used to track intravenously injected MSCs |
101 |
Murine induced pluripotent stem cells |
Intramyocardial |
SPION |
Mice |
— |
Bioluminescence and MRI were used to track transplanted iPSC |
102 |
Autologous BM-MSCs |
Catheter-based delivery and intracoronary |
SPION |
Pig |
— |
MRI imaging of the transplanted cells performed using SPIONs showed that catheter-based delivery of stem cell increases the myocardial function |
103 |
BM-MSCs |
Intramyocardial |
SPION |
Sheep |
HIF1-α |
HIF1-α overexpression showed increased cardiac protection, tracked by SPION |
104 |
BM-MSCs |
Intramyocardial |
Mesoporous organo-silica nanoparticles |
Rat |
— |
NP enhances HGF gene transfection efficiency in BM-MSCs |
105 |
BM-MSCs |
Intramyocardial |
PEI |
Rat |
Hypoxamir-210 |
PEI NP is used for gene transfection of MSCs which significantly increases the cardio protective effect |
106 |
hADSCs |
Intramyocardial |
TAT peptide/DNA |
Rat |
Angiopoietin-1 (Ang1) |
Angiopoietin-1 gene delivery for acute infarction |
107 |
Cardiomyocytes |
Intramyocardial |
PEI-graphene oxide |
Rat |
VEGF |
GO nanocomplex promotes the controlled release of VEGF DNA into myocardial tissue |
108 |
CD34-positive endogenous stem cells |
Intravenous |
Platelets |
Mice |
CD-34 positive endogenous stem cells |
Platelets linked with CD-34 antibodies used to redirect circulating CD-34 positive stem cells into the infarcted myocardium |
60 |
Cardiosphere-derived cardiac stem cells (CSCs) |
Intravenous |
Platelets vesicles |
Rat and pig |
Platelets |
Platelet membrane fused CSCs used to target the injured myocardium |
59 |
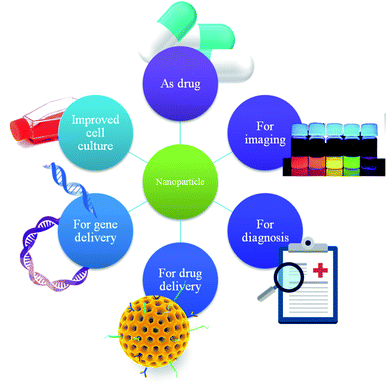 |
| Fig. 3 Scheme showing various applications of nanoparticles in cardiac stem cell therapy. | |
6.1. Nanoparticles for improved cell culture
One of the major difficulties associated with stem cell therapy for MI is in mimicking the structural, chemical and physical environments of a damaged heart, in vitro. In the case of cardiac tissues, factors such as the shape and characteristics of the extracellular matrix are important for the successful development of functional and dynamic cardiomyocytes. The role of growth hormones and various physical stresses such as cell–cell tension and electrical stimulation also have to be considered. The development of 3D structures that can support the growth of stem cells are possible due to the advancements in nanotechnology. They can even facilitate the differentiation of stem cells to functional myocardium. Scaffolds made of nanofibers, which have high tensile strength and surface area facilitates easy exchange of nutrition and waste with the extracellular environment.109 50–500 nm-sized fibers can be developed using methods such as phase-separation, electrospinning and self-assembly.110,111 It has also been shown that nanofiber polyglycolic acid and collagen scaffolds can considerably increase cell adhesion in comparison to microfiber-sized scaffolds of the same material. It was also noted that cell attachment and proliferation are much better in a 3D system incubated in a bioreactor perfusion system compared to 3D static and 2D systems. Micro and nanocomposites along with a 3D culture system are promising strategies to promote stem cell culture.112 When using a scaffold system, its shape or pattern can also influence cell maturation. In a study by Kim et al. the scaffolds made out of polyethylene glycol (PEG) with patterned ridges exhibited enhanced stem cell retention, growth, and integration than non-ridged surfaces in an MI model.90 Similar to this, cardiomyocytes grown on a PEG scaffold with surface pillars developed using UV light had greater cell adhesion than controls. These scaffolds showed greater 3D growth with improved signal conduction and contractility, as observed by the beating cells showing active action potentials.113
The addition of gold to scaffolds has also been demonstrated to increase the functionality of the system. Gold nanoparticles, nanowires etc. have been widely added to scaffolds. These studies showed that over expression of troponin I, alpha-sarcomeric actin, and connexin-43, which are crucial in providing chemical and electrical communication between cells. The increased expression of these molecules indicates better and synchronized contraction of myocytes, which is very important in the success of grafted myocardial tissue.110 Supplementing the scaffolds with growth factors such as IGF-1, VEGF have also been shown to improve cardiomyocyte growth and function.114
Still, the major hurdle in clinical stem cell therapy is the long-term survival of transplanted cells. Poorly engrafted cells at the targeted site limit their potential application in clinical medicine. Recent studies on stem cells indicated that the encapsulation of cells in microparticles increases their survival limit and enables long-term retention in the targeted tissues. The microparticles derived from PLGA and encapsulated with poly-D-lysine and collagen increased cardiomyocytes retention in mice up to 2 months. Also, the results proved that the survival limit of transplanted cells improved by 1.99 folds in 4 days and significantly enhanced cardiac activity.86 Further conjugation of specific drug molecules into nanosystems expand the cell survival limit by protecting them from oxidative stress. Melatonin, act as an endogenous antioxidant and is tested in cell culture to utilize its protective action on transplanted stem cells from oxidative injury. Melatonin encapsulated with PLGA-mPEG nanoparticles significantly protected the stem cells from oxidative injury, both in vitro and in vivo compared to melatonin alone and enhanced their survival limit during myocardial infarction.87
6.2. Nanoparticles in stem cell imaging and tracking
Labelling stem cells directly or indirectly for in vivo applications has been a topic of great interest among the scientific community. The reporter is loaded on the MSCs before administration in case of direct loading while reporter genes are genetically introduced into the cell before transplantation. Stem cell imaging and tracking are crucial as it gives a comprehensive account on the transplanted stem cell location, survival and even differentiation status.115 Direct labeling methods stand superior to indirect methods owing to their biocompatibility, simplicity, functional modification along with real-time monitoring. The most accepted label to do the job is nanoparticles.
Magnetic nanoparticles find great applications as a stem-cell-labeling agent considering the fact that MRI can be used to detect the signals and at the same time provide 2D or 3D imaging of various tissues.116,117 For example, a citrate-coated iron oxide nanoparticle was developed for MRI imaging of liver fibrosis with enhanced relaxivity.118 In another study, the same group reported dextran stabilized super paramagnetic iron oxide nanoparticles (SPIONs) with high magnetic relaxivity for the purpose of liver fibrosis imaging.119 SPIONs have been shown to label stem cells in the myocardium without altering the major properties such as cell proliferation, differentiation, migration, and viability.120,121 However, there are few reports which show that the survival rate using SPIONs is over estimated due to the presence of macrophages.122,123 In another study, superparamagnetic microspheres labeled pluripotent mouse embryonic stem (mES) cells transplanted in a rodent model of chronic MI showed largely stable locations over a period of 4 weeks. Here, major cardiac structural and functional characteristics were simultaneously evaluated using MRI using the contrast from the MNP.124 However, most of these contrast agents possess lower magnetic relaxivity and ultimately needed a large volume of contrast agents. This problem is addressed by encapsulating Gd ions in single-walled carbon nanotubes.125 Gd–liposome complex is shown to facilitate long-term stem cell tracking in vivo.126 Mojdeh et al. very recently conducted a study to develop chitosan-alginate nanoparticles carrying Dotarem for stem cell tracking.127
Optical tracking methods include the conjugation of dyes to NPs or the nanoparticle itself acting as an imaging probe. Silica NPs, gold nanorods, carbon nanotubes, carbon dots, quantum dots, etc. can act as optical probes tagged with or without optical agents. For example, a novel zerovalent iron nanoparticle was developed with liver-specific polysaccharide, pullulan. Here, by complexing the particle with fluorescent carbon dots enables both magnetic resonance and optical imaging of the liver.128 These types of nanoparticles can be used for stem cell labeling and its direct detection and tracking.116 Au nanoparticles are widely used for stem cell tracking and imaging owing to their stability and minimal cytotoxicity.129 Kim et al. in 2017 showed in vivo detection threshold of ≈2 × 104 cells using gold-poly-L-lysine nanocomplexes to label hMSCs using a micro-CT imager.130 An upconversion nanoparticle made of (α-NaYbF4:Tm3+)/CaF2 covalently conjugated with PEI was used to track rat MSCs for biomedical applications.131 Jooken et al. used quantum dots to functionalize the artificially created extracellular matrix and adopted it for ex vivo monitoring of cardiomyocytes' activity. This probe allowed high spatial and temporal resolutions and higher penetration depths, making it highly promising for biological imaging.132 In another study, a unique molecular targeting mechanism was developed, where a ROS-responsive self-assembled fluorescent nanoparticle was used to target heart tissue undergoing ischemic/reperfusion injury. Evaluation of this probe (an amphiphilic conjugate of chlorin e6, luminol, and PEG) showed highly-specific targeting to the ischemic/reperfused myocardium in a mouse model of MI.93
6.3. Nanoparticles in gene delivery
One of the widely accepted methods to overcome the challenges of reduced homing and increased angiogenesis is to genetically reprogram MSCs with required characters, prior to injection. Transfection of MSCs with VEGF cDNA or HGF cDNA followed by a myocardial injection to the border of infarcted cardiomyocytes already has shown good results.133,134 Similarly, the facially amphipathic bile acid-modified PEI (BA-PEI) conjugates have shown higher transfection efficiency, which subsequently enhanced VEGF expression and angiogenesis at the injury site.135
Although virus-mediated gene transfer has shown encouraging results with respect to cardiomyocyte survival, cardiac function and reduced fibrosis in mice, the associated safety and ethical issues hinder its translation from animal studies to clinical practice. The limitations of virus-mediated gene transfer are poor in targeting efficiency, immunogenicity and non-specificity. Nanoparticles provide an alternative and safer option for gene delivery and manipulation of MSCs for cardiac applications. But achieving nanoparticle-assisted gene delivery is not a very easy task.
The main challenge encountered by nanocarriers for DNA/siRNA is the intracellular and extracellular barriers to reach the cell. Within the extracellular milieu, the nanoplex encounters various challenges that result in quick clearance and/or degradation of the nanoplex even before it reaches the targeted site. The half-life of intravenously delivered naked pDNA within the serum, ranges from 1.2 to 21 minutes corresponding to the top form of the DNA.136 This is because of both endonuclease and exonuclease activity in the plasma. Plasmid DNA delivered intramuscularly also gives a similar degradation pattern.137 DNA can be protected from nuclease activity by the use of cationic lipids, such as DOTAP: DOPE, PEGylated lipids and polymers that can encapsulate the plasmid and thus protect it from enzymatic attack outside the cell.138 The major cationic polymers used for gene delivery include poly L-lysine, chitosan, dendrimers, polyamidoamines, polyethyleneimine, etc.
After the introduction of PEI by Boussif in 1995 for gene delivery, PEI-based nanoparticles are considered to be the gold standard of cationic polymeric vectors. PEI shows effective condensation of plasmids into colloidal particles that results in efficient transfection in various cell lines, both in vitro and in vivo.139 However nanoparticles based on PEI are generally considered to be cytotoxic.140 In an advanced study, linking PEI with succinic acid improved the hydrophilic and hydrophobic balance of the particle, which subsequently reduced the toxicity in the as-formed nanoparticles having the size of 130 nm. In 2016, Kai et al. showed that the conjugation of hollow mesoporous organo-silica nanoparticles with PEI facilitated high loading capacity of hepatocyte growth factor (HGF) gene and improved gene protection in bone marrow-derived MSCs. These cells were then transplanted to a rat MI model to prove the ability of the developed system in effective cardiac repair.105 Similarly in another study, PEI-based nanoparticles were used to stimulate ischemic preconditioning in mesenchymal stem cells to protect host cardiomyocytes by the delivery of miR-210, which is known to play a role in rescuing cardiac function after MI.106
Dendrimers have excellent properties that could facilitate gene delivery for MI treatment as they show cationic properties, enhanced cellular uptake and proton-sponge effect that mediate endosomal escape.141,142 PAMAM or polyamidoamine is one of the most widely used dendrimers.143 Functionalization of dendrimers with cell-penetrating peptides such as oligo-arginine or TAT can highly improve its cellular internalization after functionalization with PEG and also can improve its biocompatibility. Hence a cell-penetrating peptide -PEG-dendrimer could deliver angiotensin 2 type 1 receptor siRNA in a rat ischemia-reperfusion model to reduce the effects of angiotensin 2.142 Similarly, arginine grafted bio-reducible poly(disulfide amine) dendrimers carrying VEGF plasmid could enhance VEGF expression and improve blood supply in the myocardium and help in preserving the left ventricular function in the rat model.144 In another study, Wang et al. used DNA/dendrimer complexes combined with electroporation to see enhancement in gene transfection in cardiac grafts.145
Very recently, graphene-based carriers have also emerged as novel gene delivery systems. Graphene is the single carbon sheet with sp2 bond, which shows unique physical and chemical properties as it has a number of modifiable hydroxyl and carboxylic acid groups on its surface.146 Gene delivery using a combination of graphene oxide (GO) with molecules such as PEI and hydrogels has been reported. Recently, Zhang et al. demonstrated successful delivery of plasmid DNA and siRNA using PEI-GO/DNA complexes.147 In another study, a hybrid system of GO and an injectable hydrogel facilitated controlled release of PEI-GO/DNA (VEGF) nanocomplexes in myocardial tissues in a rat MI model with very good biocompatibility.108
Chitosan is a polycationic polysaccharide obtained from partial deacetylation of chitin. It is also considered as an ideal carrier for DNA and siRNA delivery owing to its good complex-forming ability, long lasting release capability and less cytotoxicity.148–150 But, the possibility of genetically modified stem cell using chitosan nanoparticles is yet to be addressed. Intriguingly, macrophages show a preferential uptake of mannosylated chitosan nanoparticles.151 This also opens future scope to explore the possibility of chitosan nanoparticles for the suppression of inflammation and attenuation of MI.
Magnetic nanoparticles can also act as agents of gene delivery as well. However, the coating strategies of these nanoparticles are a great issue. This is successfully achieved for suicide gene therapy of hepatocellular carcinoma using mesoporous silica-coated magnetic nanoparticles.152 But considering the different nature and mechanisms between cancer and infarcted cardiomyocytes, applications of magnetic nanoparticles for gene therapy are not yet known in MI.
7. Conclusion and future outlook
To summarize, NP-based approaches through non-viral gene delivery are expected to contribute significantly to the field of stem cell-based cardiac repair. There is no question on the wide range of applications available for nanoparticle-mediated cardiac stem cell therapy. CVD being a major cause for morbidity, nanoparticle-mediated stem cell therapy is the need of the hour. Scientists across the world have been attempting to bring translational potential to nanoparticles available so as to best utilize them in the clinical setting. Biosafety concerns prevent their smooth journey to be translated into the clinic. A comprehensive understanding of the characteristics of NPs and the material interaction studies with cells can benefit the design of biocompatible materials following suitable surface modification. Integrating multiple dimensions of nanoparticle-mediated stem cell therapy, early detection, tracking and treatment can be done simultaneously. Such a holistic treatment modality would surely be of significance regarding statistically common and fatal cardiac pathology, such as MI. More efforts are needed to explore the effect of NP-based modulation on other types of stem cells. In addition, mechanisms of stem cell-based cardiac repair need deep exploration for further progress in this field. We believe that NP-based stem cell therapy can benefit MI patients very soon and will also support biomedical research in this field on a large scale.
Data availability
This manuscript being a review, the data presented here are all reproduced from published articles. The figures are either drawn by the authors or reproduced with permission.
Conflicts of interest
There are no conflicts to declare.
Acknowledgements
Authors acknowledge the financial support from the Department of Biotechnology, Government of India (BT/PR27222/NNT/28/1337/2017) and Science and Engineering Research Board, DST(CVD/2020/000778). Sarathkumar E, acknowledge the Department of Science and Technology, Government of India for providing the PhD research fellowship (Project number: SEED/SCSP/2019/117/dated 20-03-2020).
References
- N. T. Feric and M. Radisic, Strategies and Challenges to Myocardial Replacement Therapy, Stem Cells Transl. Med., 2016, 5(4), 410–416, DOI:10.5966/sctm.2015-0288.
- B. Chen, X. Chen, C. Liu, J. Li, F. Liu and Y. Huang, Co-expression of Akt1 and Wnt11 promotes the proliferation and cardiac differentiation of mesenchymal stem cells and attenuates hypoxia/reoxygenation-induced cardiomyocyte apoptosis, Biomed. Pharmacother., 2018, 108, 508–514, DOI:10.1016/j.biopha.2018.09.047.
- E. Cambria, F. S. Pasqualini and P. Wolint, et al. Translational cardiac stem cell therapy: advancing from first-generation to next-generation cell types, npj Regener. Med., 2017, 2(1), 1–10, DOI:10.1038/s41536-017-0024-1.
- H. Yu, K. Lu, J. Zhu and J. Wang, Stem cell therapy for ischemic heart diseases, Br. Med. Bull., 2017, 121(1), 135–154, DOI:10.1093/bmb/ldw059.
- V. F. M. Segers and R. T. Lee, Stem-cell therapy for cardiac disease, Nature, 2008, 451(7181), 937–942, DOI:10.1038/nature06800.
- A. Radomska, J. Leszczyszyn and M. W. Radomski, The nanopharmacology and nanotoxicology of nanomaterials: New opportunities and challenges, Adv. Clin. Exp. Med., 2016, 25(1), 151–162, DOI:10.17219/acem/60879.
- J. W. Rhee and J. C. Wu, Advances in nanotechnology for the management of coronary artery disease, Trends Cardiovasc. Med., 2013, 23(2), 39–45, DOI:10.1016/j.tcm.2012.08.009.
- M. Bietenbeck, A. Florian, C. Faber, U. Sechtem and A. Yilmaz, Remote magnetic targeting of iron oxide nanoparticles for cardiovascular diagnosis and therapeutic drug delivery: Where are we now?, Int. J. Nanomed., 2016, 11, 3191–3203, DOI:10.2147/IJN.S110542.
- H. D. White, Pathobiology of troponin elevations, J. Am. Coll. Cardiol., 2011, 57(24), 2406–2408, DOI:10.1016/j.jacc.2011.01.029.
- G. Yao, G. Su, K. Li, B. Li and H. Dong, Comparative study of ticagrelor and clopidogrel in therapeutic effect of acute myocardial infarction patients undergoing percutaneous coronary intervention, Saudi J. Biol. Sci., 2017, 24(8), 1818–1820, DOI:10.1016/j.sjbs.2017.11.020.
- Heart Attack|NHLBI, NIH, https://www.nhlbi.nih.gov/health-topics/heart-attack, accessed September 16, 2021 Search PubMed.
- L. M. Friedman, A Randomized Trial of Propranolol in Patients With Acute Myocardial Infarction: I. Mortality Results, JAMA, J. Am. Med. Assoc., 1982, 247(12), 1707–1714, DOI:10.1001/jama.1982.03320370021023.
- A. Abbate, M. C. Kontos and J. D. Grizzard, et al. Interleukin-1 Blockade With Anakinra to Prevent Adverse Cardiac Remodeling After Acute Myocardial Infarction (Virginia Commonwealth University Anakinra Remodeling Trial [VCU-ART] Pilot Study), Am. J. Cardiol., 2010, 105(10), 1371–1377, DOI:10.1016/j.amjcard.2009.12.059.
- G. G. Amaral, V. C. de Oliveira and E. A. de Azevedo Guimarães, et al. Evaluation of the Psychometric Properties of the Immunobiological Agent Conservation Assessment Scale, J. Nurs. Meas., 2020, JNM-D-20-00032, DOI:10.1891/jnm-d-20-00032.
- M. A. DeWood, J. Spores and R. Notske, et al. Prevalence of Total Coronary Occlusion during the Early Hours of Transmural Myocardial Infarction, N. Engl. J. Med., 1980, 303(16), 897–902, DOI:10.1056/nejm198010163031601.
- A. C. Elliott, G. L. Smith, D. A. Eisner and D. G. Allen, Metabolic changes during ischaemia and their role in contractile failure in isolated ferret hearts, J. Physiol., 1992, 454(1), 467–490, DOI:10.1113/jphysiol.1992.sp019274.
- R. J. Solaro, J. A. Lee, J. C. Kentish and D. G. Allen, Effects of acidosis on ventricular muscle from adult and neonatal rats, Circ. Res., 1988, 63(4), 779–787, DOI:10.1161/01.RES.63.4.779.
- S. Mythili and N. Malathi, Diagnostic markers of acute myocardial infarction, Biomed. Rep., 2015, 3(6), 743–748, DOI:10.3892/br.2015.500.
- V. Lubrano, Consolidated and emerging inflammatory markers in coronary artery disease, World J. Exp. Med., 2015, 5(1), 21, DOI:10.5493/wjem.v5.i1.21.
- A. Moretti, L. Caron and A. Nakano, et al. Multipotent Embryonic Isl1+ Progenitor Cells Lead to Cardiac, Smooth Muscle, and Endothelial Cell Diversification, Cell, 2006, 127(6), 1151–1165, DOI:10.1016/j.cell.2006.10.029.
- A. Leri, J. Kajstura and P. Anversa, Cardiac stem cells and mechanisms of myocardial regeneration, Physiol. Rev., 2005, 85(4), 1373–1416, DOI:10.1152/physrev.00013.2005.
- F. Quaini, K. Urbanek and A. P. Beltrami, et al. Chimerism of the Transplanted Heart, N. Engl. J. Med., 2002, 346(1), 5–15, DOI:10.1056/nejmoa012081.
- O. Bergmann, R. D. Bhardwaj and S. Bernard, et al. Evidence for cardiomyocyte renewal in humans, Science, 2009, 324(5923), 98–102, DOI:10.1126/science.1164680.
- E. Lázár, H. A. Sadek and O. Bergmann, Cardiomyocyte renewal in the human heart: Insights fromthe fall-out, Eur. Heart J., 2017, 38(30), 2333–2339, DOI:10.1093/eurheartj/ehx343.
- M. Magarin, H. Schulz, L. Thierfelder and J.-D. Drenckhahn, Transcriptional profiling of regenerating embryonic mouse hearts, Genomics Data, 2016, 9, 145–147, DOI:10.1016/j.gdata.2016.08.009.
- J. D. Drenckhahn, Q. P. Schwarz and S. Gray, et al. Compensatory Growth of Healthy Cardiac Cells in the Presence of Diseased Cells Restores Tissue Homeostasis during Heart Development, Dev. Cell, 2008, 15(4), 521–533, DOI:10.1016/j.devcel.2008.09.005.
- E. R. Porrello and E. N. Olson, A neonatal blueprint for cardiac regeneration, Stem Cell Res., 2014, 13(3), 556–570, DOI:10.1016/j.scr.2014.06.003.
- A. I. Mahmoud, E. R. Porrello, W. Kimura, E. N. Olson and H. A. Sadek, Surgical models for cardiac regeneration in neonatal mice, Nat. Protoc., 2014, 9(2), 305–311, DOI:10.1038/nprot.2014.021.
- K. Nakamura and C. E. Murry, Function Follows Form — A Review of Cardiac Cell Therapy, Circ. J., 2019, 83(12), 2399, DOI:10.1253/CIRCJ.CJ-19-0567.
- V. Karantalis and J. M. Hare, Use of mesenchymal stem cells for therapy of cardiac disease, Circ. Res., 2015, 116(8), 1413–1430, DOI:10.1161/circresaha.116.303614.
- D. Orlic, J. Kajstura, S. Chimenti, I. Jakoniuk, S. M. Anderson, B. Li, J. Pickel, R. McKay, B. Nadal-Ginard, D. M. Bodine, A. Leri and P. Anversa, Nature, 2001, 410(6829), 701–705, DOI:10.1038/35070587.
- P. Dixit and R. Katare, Challenges in identifying the best source of stem cells for cardiac regeneration therapy, Stem Cell Res. Ther., 2015, 6(1), 1–12, DOI:10.1186/s13287-015-0010-8.
- T. Xue, H. C. Cho and F. G. Akar, et al. Functional integration of electrically active cardiac derivatives from genetically engineered human embryonic stem cells with quiescent recipient ventricular cardiomyocytes: Insights into the development of cell-based pacemakers, Circulation, 2005, 111(1), 11–20, DOI:10.1161/01.CIR.0000151313.18547.A2.
- J. J. H. Chong, X. Yang and C. W. Don, et al. Human embryonic-stem-cell-derived cardiomyocytes regenerate non-human primate hearts, Nature, 2014, 510(7504), 273–277, DOI:10.1038/nature13233.
- P. Dutta, H. B. Sager and K. R. Stengel, et al. Myocardial infarction activates CCR2+ hematopoietic stem and progenitor cells, Cell Stem Cell, 2015, 16(5), 477–487, DOI:10.1016/j.stem.2015.04.008.
- U. M. Fischer, M. T. Harting and F. Jimenez, et al. Pulmonary passage is a major obstacle for intravenous stem cell delivery: The pulmonary first-pass effect, Stem Cells Dev., 2009, 18(5), 683–691, DOI:10.1089/scd.2008.0253.
- A. Abbott, Doubts over heart stem-cell therapy, Nature, 2014, 508(7498), 15–16, DOI:10.1038/509015a.
- M. F. Pittenger, A. M. Mackay and S. C. Beck, et al. Multilineage potential of adult human mesenchymal stem cells, Science, 1999, 284(5411), 143–147, DOI:10.1126/science.284.5411.143.
- D. Kuraitis, M. Ruel and E. J. Suuronen, Mesenchymal stem cells for cardiovascular regeneration, Cardiovasc. Drugs Ther., 2011, 25(4), 349–362, DOI:10.1007/s10557-011-6311-y.
- Y. Miyahara, N. Nagaya and M. Kataoka, et al. Monolayered mesenchymal stem cells repair scarred myocardium after myocardial infarction, Nat. Med., 2006, 12(4), 459–465, DOI:10.1038/nm1391.
- K. Takahashi and S. Yamanaka, Induction of Pluripotent Stem Cells from Mouse Embryonic and Adult Fibroblast Cultures by Defined Factors, Cell, 2006, 126(4), 663–676, DOI:10.1016/j.cell.2006.07.024.
- S. P. Yu, Z. Wei and L. Wei, Preconditioning Strategy in Stem Cell Transplantation Therapy, Transl. Stroke Res., 2013, 4(1), 76–88, DOI:10.1007/s12975-012-0251-0.
- S. V. Rojas, G. Kensah and A. Rotaermel, et al. Transplantation of purified iPSC-derived cardiomyocytes in myocardial infarction, PLoS One, 2017, 12(5), 1–14, DOI:10.1371/journal.pone.0173222.
- J. W. Riggs, B. L. Barrilleaux, N. Varlakhanova, K. M. Bush, V. Chan and P. S. Knoepfler, Induced pluripotency and oncogenic transformation are related processes, Stem Cells Dev., 2013, 22(1), 37–50, DOI:10.1089/scd.2012.0375.
- N. Christoforou, B. Liau, S. Chakraborty, M. Chellapan, N. Bursac and K. W. Leong, Induced Pluripotent Stem Cell-Derived Cardiac Progenitors Differentiate to Cardiomyocytes and Form Biosynthetic Tissues, PLoS One, 2013, 8(6), 1–17, DOI:10.1371/journal.pone.0065963.
- M. B. Fisher and R. L. Mauck, Tissue engineering and regenerative medicine: Recent innovations and the transition to translation, Tissue Eng., Part B, 2013, 19(1), 1–13, DOI:10.1089/ten.teb.2012.0723.
- A. P. Beltrami, L. Barlucchi and D. Torella, et al. Adult cardiac stem cells are multipotent and support myocardial regeneration, Cell, 2003, 114(6), 763–776, DOI:10.1016/S0092-8674(03)00687-1.
- B. Nadal-Ginard, J. Kajstura, A. Leri and P. Anversa, Myocyte death, growth, and regeneration in cardiac hypertrophy and failure, Circ. Res., 2003, 92(2), 139–150, DOI:10.1161/01.RES.0000053618.86362.DF.
- T. L. E. None, Expression of concern: The SCIPIO trial, Lancet, 2014, 383(9925), 1279, DOI:10.1016/S0140-6736(14)60608-5.
- R. R. Makkar, R. R. Smith and K. Cheng, et al. Intracoronary cardiosphere-derived cells for heart regeneration after myocardial infarction (CADUCEUS): A prospective, randomised phase 1 trial, Lancet, 2012, 379(9819), 895–904, DOI:10.1016/S0140-6736(12)60195-0.
- N. Balafkan, S. Mostafavi and M. Schubert, et al. A method for differentiating human induced pluripotent stem cells toward functional cardiomyocytes in 96-well microplates, Sci. Rep., 2020, 10(1), 1–14, DOI:10.1038/s41598-020-73656-2.
- C. L. Mummery, J. Zhang, E. S. Ng, D. A. Elliott, A. G. Elefanty and T. J. Kamp, Differentiation of Human ES and iPS Cells to Cardiomyocytes: A Methods Overview, Circ. Res., 2012, 111(3), 344, DOI:10.1161/circresaha.110.227512.
- J. Hoover-Plow and Y. Gong, Challenges for heart disease stem cell therapy, Vasc. Health Risk Manage., 2012, 8(1), 99–113, DOI:10.2147/vhrm.s25665.
- Y. Yamada, S. Wakao and Y. Kushida, et al. S1P–S1PR2 axis mediates homing of muse cells into damaged heart for long-lasting tissue repair and functional recovery after acute myocardial infarction, Circ. Res., 2018, 122(8), 1069–1083, DOI:10.1161/circresaha.117.311648.
- M. Monsanto, B. Wang, Z. Ehrenberg, R. Alvarez and M. Sussman, CardioClusters: Enhancing Stem Cell Engraftment and Myocardial Repair, J. Mol. Cell. Cardiol., 2018, 124, 85, DOI:10.1016/j.yjmcc.2018.07.014.
- A. Gottipati, L. Chelvarajan and H. Peng, et al. Gelatin Based Polymer Cell Coating Improves Bone Marrow-Derived Cell Retention in the Heart after Myocardial Infarction, Stem Cell Rev. Rep., 2019, 15(3), 404–414, DOI:10.1007/s12015-018-9870-5.
- M. Abdelmonem, N. N. Shahin, L. A. Rashed, H. A. A. Amin, A. A. Shamaa and A. A. Shaheen, Hydrogen sulfide enhances the effectiveness of mesenchymal stem cell therapy in rats with heart failure: In vitro preconditioning versus in vivo co-delivery, Biomed. Pharmacother., 2019, 112, 108584, DOI:10.1016/j.biopha.2019.01.045.
- K. Ghoshal and M. Bhattacharyya, Overview of platelet physiology: Its hemostatic and nonhemostatic role in disease pathogenesis, Sci. World J., 2014, 2014, 1–16, DOI:10.1155/2014/781857.
- J. Tang, T. Su and K. Huang, et al. Targeted repair of heart injury by stem cells fused with platelet nanovesicles, Nat. Biomed. Eng., 2018, 2(1), 17–26, DOI:10.1038/s41551-017-0182-x.
- D. Shen, Z. Li and S. Hu, et al. Antibody-Armed Platelets for the Regenerative Targeting of Endogenous Stem Cells, Nano Lett., 2019, 19(3), 1883–1891, DOI:10.1021/acs.nanolett.8b04970.
- M. Zhang, D. Methot, V. Poppa, Y. Fujio, K. Walsh and C. E. Murry, Cardiomyocyte grafting for cardiac repair: Graft cell death and anti-death strategies, J. Mol. Cell. Cardiol., 2001, 33(5), 907–921, DOI:10.1006/jmcc.2001.1367.
- C. Toma, M. F. Pittenger, K. S. Cahill, B. J. Byrne and P. D. Kessler, Human mesenchymal stem cells differentiate to a cardiomyocyte phenotype in the adult murine heart, Circulation, 2002, 105(1), 93–98, DOI:10.1161/hc0102.101442.
- Y. L. Tang, Y. Tang, Y. C. Zhang, K. Qian, L. Shen and M. I. Phillips, Improved graft mesenchymal stem cell survival in ischemic heart with a hypoxia-regulated heme oxygenase-1 vector, J. Am. Coll. Cardiol., 2005, 46(7), 1339–1350, DOI:10.1016/j.jacc.2005.05.079.
- Y. L. Tang, Y. Tang, Y. C. Zhang, K. Qian, L. Shen and M. I. Phillips, Improved graft mesenchymal stem cell survival in ischemic heart with a hypoxia-regulated heme oxygenase-1 vector, J. Am. Coll. Cardiol., 2005, 46(7), 1339–1350, DOI:10.1016/j.jacc.2005.05.079.
- M. Hu, G. Guo and Q. Huang, et al. The harsh microenvironment in infarcted heart accelerates transplanted bone marrow mesenchymal stem cells injury: The role of injured cardiomyocytes-derived exosomes, Cell Death Discovery, 2018, 9(3), 1–14, DOI:10.1038/s41419-018-0392-5.
- Z. Fan, Z. Xu and H. Niu, et al. An Injectable Oxygen Release System to Augment Cell Survival and Promote Cardiac Repair Following Myocardial Infarction, Sci. Rep., 2018, 8(1), 1–22, DOI:10.1038/s41598-018-19906-w.
- D. Y. Zhang, C. F. Zhang and B. C. Fu, et al. Sirtuin3 protects aged human mesenchymal stem cells against oxidative stress and enhances efficacy of cell therapy for ischaemic heart diseases, J. Cell. Mol. Med., 2018, 22(11), 5504–5517, DOI:10.1111/jcmm.13821.
- G. t. Yao, L. p. Song, W. h. Xue, G. h. Su, A. h. Ning and J. Wang, Nano-particle engineered atorvastatin delivery to support mesenchymal stem cell survival in infarcted myocardium, Saudi J. Biol. Sci., 2018, 25(6), 1016–1021, DOI:10.1016/j.sjbs.2017.03.015.
- J. Pons, Y. Huang and J. Arakawa-Hoyt, et al. VEGF improves survival of mesenchymal stem cells in infarcted hearts, Biochem. Biophys. Res. Commun., 2008, 376(2), 419–422, DOI:10.1016/j.bbrc.2008.09.003.
- M. Mirotsou, Z. Zhang and A. Deb, et al. Secreted frizzled related protein 2 (Sfrp2) is the key Akt-mesenchymal stem cell-released paracrine factor mediating myocardial survival and repair, Proc. Natl. Acad. Sci. U. S. A., 2007, 104(5), 1643–1648, DOI:10.1073/pnas.0610024104.
- D. Feng, L. Zhang and F. Ding, et al. Blocking Nox2 improves mesenchymal stem cells therapy in myocardial infarction via antagonizing oxidant and promoting survival, J. Cell. Physiol., 2018, 233(10), 7004–7015, DOI:10.1002/jcp.26623.
- Y. Yao, W. Liao, R. Yu, Y. Du, T. Zhang and Q. Peng, Potentials of combining nanomaterials and stem cell therapy in myocardial repair, Nanomedicine, 2018, 13(13), 1623–1638, DOI:10.2217/nnm-2018-0013.
- S. Su, C. Zhang and L. Yuwen, et al. Creating SERS hot spots on MoS2 nanosheets with in situ grown gold nanoparticles, ACS Appl. Mater. Interfaces, 2014, 6(21), 18735–18741, DOI:10.1021/am5043092.
- X. R. Shao, X. Q. Wei and X. Song, et al. Independent effect of polymeric nanoparticle zeta potential/surface charge, on their cytotoxicity and affinity to cells, Cell Proliferation, 2015, 48(4), 465–474, DOI:10.1111/cpr.12192.
- F. Danhier, E. Ansorena, J. M. Silva, R. Coco, A. Le Breton and V. Préat, PLGA-based nanoparticles: An overview of biomedical applications, J. Controlled Release, 2012, 161(2), 505–522, DOI:10.1016/j.jconrel.2012.01.043.
- F. Meng, Y. Zhong, R. Cheng, C. Deng and Z. Zhong, PH-sensitive polymeric nanoparticles for tumor-targeting doxorubicin delivery: Concept and recent advances, Nanomedicine, 2014, 9(3), 487–499, DOI:10.2217/nnm.13.212.
- X. R. Shao, X. Q. Wei and S. Zhang, et al. Effects of Micro-environmental pH of Liposome on Chemical Stability of Loaded Drug, Nanoscale Res. Lett., 2017, 12(1), 504, DOI:10.1186/s11671-017-2256-9.
- K. Zabielska-Koczywąs and R. Lechowski, The use of liposomes and nanoparticles as drug delivery systems to improve cancer treatment in dogs and cats, Molecules, 2017, 22(12), 1–14, DOI:10.3390/molecules22122167.
- E. Masoudipour, S. Kashanian, N. Maleki, A. Karamyan and K. Omidfar, A novel intracellular pH-responsive formulation for FTY720 based on PEGylated graphene oxide nano-sheets, Drug Dev. Ind. Pharm., 2018, 44(1), 99–108, DOI:10.1080/03639045.2017.1386194.
- K. H. Son, J. H. Hong and J. W. Lee, Carbon nanotubes as cancer therapeutic carriers and mediators, Int. J. Nanomed., 2016, 11, 5163–5185, DOI:10.2147/IJN.S112660.
- J. Joshi and C. Kothapalli, Nanofibers Based Tissue Engineering and Drug Delivery Approaches for Myocardial Regeneration, Curr. Pharm. Des., 2015, 21(15), 2006–2020, DOI:10.2174/1381612821666150302153138.
- Y. T. Ho, B. Poinard and J. C. Y. Kah, Nanoparticle drug delivery systems and their use in cardiac tissue therapy, Nanomedicine, 2016, 11(6), 693–714, DOI:10.2217/nnm.16.6.
- J. H. Traverse, Using biomaterials to improve the efficacy of cell therapy following acute myocardial infarction, Journal of Cardiovascular Translational Research, 2012, 5(1), 67–72, DOI:10.1007/s12265-011-9330-y.
- M. Kharaziha, A. Memic, M. Akbari, D. A. Brafman and M. Nikkhah, Nano-Enabled Approaches for Stem Cell-Based Cardiac Tissue Engineering, Adv. Healthcare Mater., 2016, 5(13), 1533–1553, DOI:10.1002/adhm.201600088.
- C. E. Semino, Self-assembling peptides: From bio-inspired materials to bone regeneration, J. Dent. Res., 2008, 87(7), 606–616, DOI:10.1177/154405910808700710.
- L. Saludas, E. Garbayo and M. Mazo, et al. Long-term engraftment of human cardiomyocytes combined with biodegradable microparticles induces heart repair, J. Pharmacol. Exp. Ther., 2019, 370(3), 761–771, DOI:10.1124/jpet.118.256065.
- Q. Ma, J. Yang and X. Huang, et al. Poly(Lactide-Co-Glycolide)-Monomethoxy-Poly-(Polyethylene Glycol) Nanoparticles Loaded with Melatonin Protect Adipose-Derived Stem Cells Transplanted in Infarcted Heart Tissue, Stem Cells, 2018, 36(4), 540–550, DOI:10.1002/stem.2777.
- R. Yokoyama, M. Ii and M. Masuda, et al. Cardiac Regeneration by Statin-Polymer Nanoparticle-Loaded Adipose-Derived Stem Cell Therapy in Myocardial Infarction, Stem Cells Transl. Med., 2019, 8(10), 1055–1067, DOI:10.1002/sctm.18-0244.
- Y. Li, Y. Yao, Z. Sheng, Y. Yang and G. Ma, Dual-modal tracking of transplanted mesenchymal stem cells after myocardial infarction, Int. J. Nanomed., 2011, 6, 815–823, DOI:10.2147/ijn.s17611.
- D. H. Kim, Kshitiz and R. R. Smith, et al. Nanopatterned cardiac cell patches promote stem cell niche formation and myocardial regeneration, Integr. Biol., 2012, 4(9), 1019–1033, 10.1039/c2ib20067h.
- J. Han, B. Kim and J. Y. Shin, et al. Iron oxide nanoparticle-mediated development of cellular gap junction crosstalk to improve mesenchymal stem cells' therapeutic efficacy for myocardial infarction, ACS Nano, 2015, 9(3), 2805–2819, DOI:10.1021/nn506732n.
- T. Hao, J. Li and F. Yao, et al. Injectable Fullerenol/Alginate Hydrogel for Suppression of Oxidative Stress Damage in Brown Adipose-Derived Stem Cells and Cardiac Repair, ACS Nano, 2017, 11(6), 5474–5488, DOI:10.1021/acsnano.7b00221.
- M. Ziegler, X. Xu, M. L. Yap, H. Hu, J. Zhang and K. Peter, A Self-Assembled Fluorescent Nanoprobe for Imaging and Therapy of Cardiac Ischemia/Reperfusion Injury, Adv. Ther., 2019, 2(3), 1800133, DOI:10.1002/adtp.201800133.
- A. Blocki, S. Beyer and J. Y. Dewavrin, et al. Microcapsules engineered to support mesenchymal stem cell (MSC) survival and proliferation enable long-term retention of MSCs in infarcted myocardium, Biomaterials, 2015, 53, 12–24, DOI:10.1016/j.biomaterials.2015.02.075.
- Z. Huang, Y. Shen and A. Sun, et al. Magnetic targeting enhances retrograde cell retention in a rat model of myocardial infarction, Stem Cell Res. Ther., 2013, 4(6), 1–13, DOI:10.1186/scrt360.
- A. C. Vandergriff, T. M. Hensley and E. T. Henry, et al. Magnetic targeting of cardiosphere-derived stem cells with ferumoxytol nanoparticles for treating rats with myocardial infarction, Biomaterials, 2014, 35(30), 8528–8539, DOI:10.1016/j.biomaterials.2014.06.031.
- F. Chen, E. R. Zhao and G. Hableel, et al. Increasing the Efficacy of Stem Cell Therapy via Triple-Function Inorganic Nanoparticles, ACS Nano, 2019, 13, 6605–6617, DOI:10.1021/acsnano.9b00653.
- J. Chen, Y. Song and Z. Huang, et al. Modification with CREKA Improves Cell Retention in a Rat Model of Myocardial Ischemia Reperfusion, Stem Cells, 2019, 37(5), 663–676, DOI:10.1002/stem.2983.
- W. Dai, S. L. Hale, G. L. Kay, A. J. Jyrala and R. A. Kloner, Delivering stem cells to the heart in a collagen matrix reduces relocation of cells to other organs as assessed by nanoparticle technology, Regener. Med., 2009, 4(3), 387–395, DOI:10.2217/rme.09.2.
- H. Zhang, H. Qiao and A. Bakken, et al. Utility of dual-modality bioluminescence and MRI in monitoring stem cell survival and impact on post myocardial infarct remodeling, Acad. Radiol., 2011, 18(1), 3–12, DOI:10.1016/j.acra.2010.09.006.
- B. Zheng, M. P. Von See and E. Yu, et al. Quantitative magnetic particle imaging monitors the transplantation, biodistribution, and clearance of stem cells in vivo, Theranostics, 2016, 6(3), 291–301, DOI:10.7150/thno.13728.
- S. V. Rojas, M. Meier and R. Zweigerdt, et al. Multimodal Imaging for In Vivo Evaluation of Induced Pluripotent Stem Cells in a Murine Model of Heart Failure, Artif. Organs, 2017, 41(2), 192–199, DOI:10.1111/aor.12728.
- M. Lu, S. Zhao and Q. Liu, et al. Transplantation with autologous mesenchymal stem cells after acute myocardial infarction evaluated by magnetic resonance imaging: An experimental study, J. Thorac. Imag., 2012, 27(2), 125–135, DOI:10.1097/RTI.0b013e31820446fa.
- A. P. Hnatiuk, S. G. Ong and F. D. Olea, et al. Allogeneic mesenchymal stromal cells overexpressing mutant human Hypoxia-inducible factor 1-α (HIF1-α) in an ovine model of acute myocardial infarction, J. Am. Heart Assoc., 2016, 5(7), 1–20, DOI:10.1161/JAHA.116.003714.
- K. Zhu, M. Wu and H. Lai, et al. Nanoparticle-enhanced generation of gene-transfected mesenchymal stem cells for in vivo cardiac repair, Biomaterials, 2016, 74, 188–199, DOI:10.1016/j.biomaterials.2015.10.010.
- H. W. Kim, S. Jiang, M. Ashraf and K. H. Haider, Stem cell-based delivery of Hypoxamir-210 to the infarcted heart: Implications on stem cell survival and preservation of infarcted heart function, J. Mol. Med., 2012, 90(9), 997–1010, DOI:10.1007/s00109-012-0920-1.
- A. Paul, M. Nayan, A. A. Khan, D. Shum-Tim and S. Prakash, Angiopoietin-1-expressing adipose stem cells genetically modified with baculovirus nanocomplex: Investigation in rat heart with acute infarction, Int. J. Nanomed., 2012, 7, 663–682, DOI:10.2147/IJN.S26882.
- A. Paul, A. Hasan and H. A. Kindi, et al. Injectable graphene oxide/hydrogel-based angiogenic gene delivery system for vasculogenesis and cardiac repair, ACS Nano, 2014, 8(8), 8050–8062, DOI:10.1021/nn5020787.
- J. C. Adams, Cell-matrix contact structures, Cell. Mol. Life Sci., 2001, 58(3), 371–392, DOI:10.1007/PL00000864.
- T. Dvir, B. P. Timko and M. D. Brigham, et al. Nanowired three-dimensional cardiac patches, Nat. Nanotechnol., 2011, 6(11), 720–725, DOI:10.1038/nnano.2011.160.
- D. H. Kim, E. A. Lipke and P. Kim, et al. Nanoscale cues regulate the structure and function of macroscopic cardiac tissue constructs, Proc. Natl. Acad. Sci. U. S. A., 2010, 107(2), 565–570, DOI:10.1073/pnas.0906504107.
- H. Hosseinkhani, M. Hosseinkhani, S. Hattori, R. Matsuoka and N. Kawaguchi, Micro and nano-scale in vitro 3D culture system for cardiac stem cells, J. Biomed. Mater. Res., Part A, 2010, 94(1), 1–8, DOI:10.1002/jbm.a.32676.
- D. H. Kim, P. Kim and I. Song, et al. Guided three-dimensional growth of functional cardiomyocytes on polyethylene glycol nanostructures, Langmuir, 2006, 22(12), 5419–5426, DOI:10.1021/la060283u.
- M. E. Davis, P. C. H. Hsieh and T. Takahashi, et al. Local myocardial insulin-like growth factor 1 (IGF-1) delivery with biotinylated peptide nanofibers improves cell therapy for myocardial infarction, Proc. Natl. Acad. Sci. U. S. A., 2006, 103(21), 8155–8160, DOI:10.1073/pnas.0602877103.
- J. F. Lau, S. A. Anderson, E. Adler and J. A. Frank, Imaging approaches for the study of cell-based cardiac therapies, Nat. Rev. Cardiol., 2010, 7(2), 97–105, DOI:10.1038/nrcardio.2009.227.
- K. Zhu, J. Li, Y. Wang, H. Lai and C. Wang, Nanoparticles-assisted stem cell therapy for ischemic heart disease, Stem Cells Int., 2016, 2016(1384658), 1–9, DOI:10.1155/2016/1384658.
- R. Hachani, M. Lowdell, M. Birchall and N. T. K. Thanh, Tracking stem cells in tissue-engineered organs using magnetic nanoparticles, Nanoscale, 2013, 5(23), 11362–11373, 10.1039/c3nr03861k.
- A. Saraswathy, S. S. Nazeer and M. Jeevan, et al. Citrate coated iron oxide nanoparticles with enhanced relaxivity for in vivo magnetic resonance imaging of liver fibrosis, Colloids Surf., B, 2014, 117, 216–224, DOI:10.1016/j.colsurfb.2014.02.034.
- A. Saraswathy, S. S. Nazeer, N. Nimi, S. Arumugam, S. J. Shenoy and R. S. Jayasree, Synthesis and characterization of dextran stabilized superparamagnetic iron oxide nanoparticles for in vivo MR imaging of liver fibrosis, Carbohydr. Polym., 2014, 101(1), 760–768, DOI:10.1016/j.carbpol.2013.10.015.
- L. Li, W. Jiang and K. Luo, et al. Superparamagnetic iron oxide nanoparticles as MRI contrast agents for non-invasive stem cell labeling and tracking, Theranostics, 2013, 3(8), 595–615, DOI:10.7150/thno.5366.
- E. Bull, S. Y. Madani, R. Sheth, A. Seifalian, M. Green and A. M. Seifalian, Stem cell tracking using iron oxide nanoparticles, Int. J. Nanomed., 2014, 9(1), 1641–1653, DOI:10.2147/IJN.S48979.
- N. Ma, H. Cheng and M. Lu, et al. Magnetic Resonance Imaging with Superparamagnetic Iron Oxide Fails to Track the Long-term Fate of Mesenchymal Stem Cells Transplanted into Heart, Sci. Rep., 2015, 5(9058), 1–9, DOI:10.1038/srep09058.
- Z. Huang, C. Li and S. Yang, et al. Magnetic resonance hypointensive signal primarily originates from extracellular iron particles in the long-term tracking of mesenchymal stem cells transplanted in the infarcted myocardium, Int. J. Nanomed., 2015, 10, 1679–1690, DOI:10.2147/IJN.S77858.
- S. N. Ebert, D. G. Taylor and H.-L. Nguyen, et al. Noninvasive Tracking of Cardiac Embryonic Stem Cells In Vivo Using Magnetic Resonance Imaging Techniques, Stem Cells, 2007, 25(11), 2936–2944, DOI:10.1634/stemcells.2007-0216.
- L. A. Tran, R. Krishnamurthy and R. Muthupillai, et al. Gadonanotubes as magnetic nanolabels for stem cell detection, Biomaterials, 2010, 31(36), 9482–9491, DOI:10.1016/j.biomaterials.2010.08.034.
- J. Guenoun, G. A. Koning and G. Doeswijk, et al. Cationic Gd-DTPA liposomes for highly efficient labeling of mesenchymal stem cells and cell tracking with MRI, Cell Transplant., 2012, 21(1), 191–205, DOI:10.3727/096368911X593118.
- M. Mohseni, S. Shojaei, B. Mehravi and E. Mohammadi, Natural polymeric nanoparticles as a non-invasive probe for mesenchymal stem cell labelling, Artif. Cells, Nanomed., Biotechnol., 2020, 48(1), 770–776, DOI:10.1080/21691401.2020.1748641.
- N. Nimi, A. Saraswathy, S. S. Nazeer, N. Francis, S. J. Shenoy and R. S. Jayasree, Multifunctional hybrid nanoconstruct of zerovalent iron and carbon dots for magnetic resonance angiography and optical imaging: An In vivo study, Biomaterials, 2018, 171, 46–56, DOI:10.1016/j.biomaterials.2018.04.012.
- X. Liu, Z. Yang, J. Sun, T. Ma, F. Hua and Z. Shen, A brief review of cytotoxicity of nanoparticles on mesenchymal stem cells in regenerative medicine, Int. J. Nanomed., 2019, 14, 3875–3892, DOI:10.2147/IJN.S205574.
- T. Kim, N. Lee and D. R. Arifin, et al. In Vivo Micro-CT Imaging of Human Mesenchymal Stem Cells Labeled with Gold-Poly- L-Lysine Nanocomplexes, Adv. Funct. Mater., 2017, 27(3), 1604213, DOI:10.1002/adfm.201604213.
- L. Zhao, A. Kutikov, J. Shen, C. Duan, J. Song and G. Han, Stem cell labeling using polyethylenimine conjugated(α-NaYbF4: Tm3+)/CaF2 upconversion nanoparticles, Theranostics, 2013, 3(4), 249–257, DOI:10.7150/thno.5432.
- S. Jooken, Y. De Coene and O. Deschaume, et al. Quantum Dot-Functionalized Extracellular Matrices for in Situ Monitoring of Cardiomyocyte Activity, ACS Appl. Nano Mater., 2020, 3(6), 6118–6126, DOI:10.1021/acsanm.0c01358.
- C. Yan, X.-J. Quan and Y.-M. Feng, Nanomedicine for Gene Delivery for the Treatment of Cardiovascular Diseases, Curr. Gene Ther., 2018, 19(1), 20–30, DOI:10.2174/1566523218666181003125308.
- T. Deuse, C. Peter and P. W. M. Fedak, et al. Hepatocyte growth factor or vascular endothelial growth factor gene transfer maximizes mesenchymal stem cell-based myocardial salvage after acute myocardial infarction, Circulation, 2009, 120(suppl. 1), S247–S254, DOI:10.1161/circulationaha.108.843680.
- H. H. Moon, M. K. Joo and H. Mok, et al. MSC-based VEGF gene therapy in rat myocardial infarction model using facial amphipathic bile acid-conjugated polyethyleneimine, Biomaterials, 2014, 35(5), 1744–1754, DOI:10.1016/j.biomaterials.2013.11.019.
- M. Ruponen, P. Honkakoski, S. Rönkkö, J. Pelkonen, M. Tammi and A. Urtti, Extracellular and intracellular barriers in non-viral gene delivery, in Journal of Controlled Release, Elsevier, 2003, vol. 93, pp. 213–217, DOI:10.1016/j.jconrel.2003.08.004.
- R. J. Mumper, J. G. Duguid, K. Anwer, M. K. Barron, H. Nitta and A. P. Rolland, Polyvinyl derivatives as novel interactive polymers for controlled gene delivery to muscle, Pharm. Res., 1996, 13(5), 701–709, DOI:10.1023/A:1016039330870.
- B. Sternberg, K. Hong, W. Zheng and D. Papahadjopoulos, Ultrastructural characterization of cationic liposome-DNA complexes showing enhanced stability in serum and high transfection activity in vivo, Biochim. Biophys. Acta, Biomembr., 1998, 1375(1–2), 23–35, DOI:10.1016/S0005-2736(98)00129-1.
- O. Boussif, F. LezoualC'H and M. A. Zanta, et al. A versatile vector for gene and oligonucleotide transfer into cells in culture and in vivo: Polyethylenimine, Proc. Natl. Acad. Sci. U. S. A., 1995, 92(16), 7297–7301, DOI:10.1073/pnas.92.16.7297.
- B. Sharma, W. Ma, I. M. Adjei, J. Panyam, S. Dimitrijevic and V. Labhasetwar, Nanoparticle-mediated p53 gene therapy for tumor inhibition, Drug Delivery Transl. Res., 2011, 1(1), 43–52, DOI:10.1007/s13346-010-0008-9.
- D. Mason, Y. Z. Chen, H. V. Krishnan and S. Sant, Cardiac gene therapy: Recent advances and future directions, J. Controlled Release, 2015, 215, 101–111, DOI:10.1016/j.jconrel.2015.08.001.
- J. Liu, C. Gu and E. B. Cabigas, et al. Functionalized dendrimer-based delivery of angiotensin type 1 receptor siRNA for preserving cardiac function following infarction, Biomaterials, 2013, 34(14), 3729–3736, DOI:10.1016/j.biomaterials.2013.02.008.
- F. Abedi-Gaballu, G. Dehghan and M. Ghaffari, et al. PAMAM dendrimers as efficient drug and gene delivery nanosystems for cancer therapy, Applied Materials Today, 2018, 12, 177–190, DOI:10.1016/j.apmt.2018.05.002.
- Y. W. Won, A. N. McGinn, M. Lee, K. Nam, D. A. Bull and S. W. Kim, Post-translational regulation of a hypoxia-responsive VEGF plasmid for the treatment of myocardial ischemia, Biomaterials, 2013, 34(26), 6229–6238, DOI:10.1016/j.biomaterials.2013.04.061.
- Y. Wang, Y. Bai and C. Price, et al. Combination of Electroporation and DNA/Dendrimer Complexes Enhances Gene Transfer into Murine Cardiac Transplants, Am. J. Transplant., 2001, 1(4), 334–338, DOI:10.1034/j.1600-6143.2001.10408.x.
- H. Zhao, R. Ding and X. Zhao, et al. Graphene-based nanomaterials for drug and/or gene delivery, bioimaging, and tissue engineering, Drug Discovery Today, 2017, 22(9), 1302–1317, DOI:10.1016/j.drudis.2017.04.002.
- L. Zhang, Z. Lu, Q. Zhao, J. Huang, H. Shen and Z. Zhang, Enhanced Chemotherapy Efficacy by Sequential Delivery of siRNA and Anticancer Drugs Using PEI-Grafted Graphene Oxide, Small, 2011, 7(4), 460–464, DOI:10.1002/smll.201001522.
- T. Huang, X. Song and J. Jing, et al. Chitosan-DNA nanoparticles enhanced the immunogenicity of multivalent DNA vaccination on mice against Trueperella pyogenes infection, J. Nanobiotechnol., 2018, 16(1), 8, DOI:10.1186/s12951-018-0337-2.
- F. Kong, G. Liu, S. Zhou, J. Guo, S. Chen and Z. Wang, Superior transfection efficiency of phagocytic astrocytes by large chitosan/DNA nanoparticles, Int. J. Biol. Macromol., 2017, 105(Pt 2), 1473–1481, DOI:10.1016/j.ijbiomac.2017.06.061.
- I. Pfeifer Dalla Picola, Q. Shi and J. Cesar Fernandes, et al. Chitosan derivatives for gene transfer: effect of phosphorylcholine and diethylaminoethyl grafts on the in vitro transfection efficiency, J. Biomater. Sci., Polym. Ed., 2016, 27(16), 1611–1630, DOI:10.1080/09205063.2016.1225333.
- G. Shilakari Asthana, A. Asthana, D. V. Kohli and S. P. Vyas, Mannosylated chitosan nanoparticles for delivery of antisense oligonucleotides for macrophage targeting, BioMed Res. Int., 2014, 2014(526391), 1–17, DOI:10.1155/2014/526391.
- Z. Wang, Z. Chang and M. Lu, et al. Shape-controlled magnetic mesoporous silica nanoparticles for magnetically-mediated suicide gene therapy of hepatocellular carcinoma, Biomaterials, 2018, 154, 147–157, DOI:10.1016/j.biomaterials.2017.10.047.
Footnote |
† Contributed equally. |
|
This journal is © The Royal Society of Chemistry 2021 |
Click here to see how this site uses Cookies. View our privacy policy here.