DOI:
10.1039/D1RA06533E
(Paper)
RSC Adv., 2021,
11, 34779-34787
A novel 3D terbium metal–organic framework as a heterogeneous Lewis acid catalyst for the cyanosilylation of aldehyde†
Received
31st August 2021
, Accepted 14th October 2021
First published on 27th October 2021
Abstract
A novel 3D lanthanide(III) metal–organic framework (MOF) (namely Tb-MOF), was synthesized by self-assembly from Tb(III) ion nitrate and the rigid organic ligand H2sbdc (H2sbdc = 5,5-dioxo-5H-dibenzo[b,d]thiophene-3,7-dicarboxylic acid), and could work as an efficient heterogeneous catalyst for the cyanosilylation of aromatic aldehydes at room temperature. The obtained Tb-MOF has been characterized and analysed in detail by single crystal X-ray diffraction, powder X-ray diffraction, thermogravimetric analysis and so on. The pores of Tb-MOF provided a microenvironment that was beneficial for the substrates to be close to the Lewis acid catalytic sites. The IR spectrogram and the fluorescence titration proved that the substrates could be activated inside the channel of Tb-MOF. The heterogeneous Tb-MOF catalyst with fine catalytic efficiency exhibited a high TON (TON = 460), and could be recycled at least three times without significantly reducing its activity.
Introduction
Two- or three-dimensional porous metal–organic frameworks (MOFs) are an important kind of functional materials, which have an inherent advantage of being readily self-assembled via suitable organic linkers and inorganic metal ions/clusters.1 Due to the unlimited coordinated bonds through the metal ions/clusters and the organic linkers, they usually appear as infinite multiple dimension networks, which endow the MOFs with diversified structures and functional applications.2 Meanwhile they usually have high porosity, large surface area and considerable capacity,3 which offer a wide range of potential applications in gas storage,4 gas capture,5 separation,6 chemical catalysis,7 luminescence,8 magnetism,9 drug delivery,10 and so on.
One special type of MOF, the lanthanide MOFs (Ln-MOFs),11 have attracted extensive attention. Firstly, Ln-MOFs have unique luminescence properties12 such as linear emission, high color purity, long fluorescence lifetime and so on. Secondly, the high coordination numbers and the flexible coordination geometries endow the Ln-MOFs with abundant topological structures.13 In addition, the organic linkers can be designed and selected to synthesize MOFs, which can be used as target materials.14 Thirdly, inside the channels of the MOFs, it contains functional sites such as open metal sites, hydrogen bonding sites and organic coordinate sites, which are highly ordered.15 Some of the Ln-MOFs are stable and even more stable than their transition-metal-based analogues,16 which are easy to activate the substrates without its framework collapse.17 In addition, Ln-MOFs as attractive Lewis acid catalysts have well-proportioned Lewis acid sites within its pores/channels which afford a platform to activate the substrates and make the catalyst reaction continue.18 Compared to the homogeneous catalysts, the heterogeneous Ln-MOFs can provide uniformly dispersed microenvironment, which is beneficial for the substrates to be close to the functional sites and easy to be separated.19 Compared to the other heterogenous materials such as zeolites,20 which have no organic moiety and are generally inactive for reactions in water, the Ln-MOFs can be well designed and tuned.21
On the other hand, cyanohydrins are very important for chemistry, which could be widely used for starting materials for the synthesis of many kinds of pivotal intermediates in chemistry, such as α-amino acids, β-amino alcohols, α-hydroxy acids, α-hydroxy ketones, and so on.22 The product of cyanosilylation of aldehydes with cyanides, such as trimethylsilyl cyanide (Me3SiCN or TMSCN), which allows the cyanohydrins to be prepared as the corresponding trimethylsilyl ether, could be readily converted into cyanohydrins.23 As a result, cyanosilylation between aldehydes and Me3SiCN has attracted a lot of attention from the scientists in the past few years.
Thermally stable MOFs (for example MIL-47 (V), MIL-53 (Al), MIL-101 (Cr), and UiO-66 (Zr)) have been used for catalyzing the cyanosilylation based on their Lewis acid sites.24 In this paper, the luminescent lanthanide ion Tb(III) was selected to assemble with the rigid ligand H2sbdc with bi-carboxyl acid group to construct a stable 3D porous MOF, and the Tb-MOF with coordinative unsaturated Tb centers are able to sever as an efficient heterogeneous catalyst for the cyanosilylation of a series of aldehyde substrates.25
Experimental section
Materials and instrumentation
All the chemicals were purchased from commercial sources and used without any further purification. 1H-NMR spectra were recorded with BRUKER AVANCE III 500 NMR instrument (CDCl3 solution) at 500 MHz. The single-crystal structure was tested on a D8 Venture SMART-CCD instrument with Mo-Kα radiation designed by Bruker from Germany. X-ray powder diffraction (XRD) measurements were performed with Cu-Kα radiation source (λ = 0.15406 nm) at 40 kV voltage and 25 mA current. IR spectra were performed on an IS50 FT-IR instrument. Thermogravimetric analysis was finished on a SDTQ600 thermogravimetric analysis instrument under N2 condition. The content of C, H and N was analysed by vario EL III elemental analysis instrument designed by Elementar Company from Germany.
Synthesis of Tb-MOF
0.3 mmol (0.091 g) H2sbdc and 0.2 mmol (0.091 g) Tb(NO3)3·6H2O were mixed in a 9 mL bottle with 5 mL EtOH and 1 mL H2O stirring at room temperature for 3 h. Then sealed and placed in a pre-heated oven, and kept it at 140 °C for 4 days. The product was cooled to room temperature in 2 days. The crystals were formed under static conditions for 10 days. The resulting solid was filtered, washed with methanol and diethyl ether. Finally, the colorless block crystals were obtained and dried in vacuum at room temperature for one day (elemental analysis C: 37.31%; H: 2.679%).
The method of catalysis by Tb-MOF
The typical experiment was performed as the following condition: substrate (0.5 mmol), trimethylsilyl cyanide (1.2 mmol), catalyst (12.79 mg, 4% mol of the substrate, based on the metal ions), and DCM (2 mL) in a 6 mL glass bottle in Ar environment at room temperature under continuously stirring. The corresponding yields of targeted products were successfully monitored by applying the NMR approach, which was added 0.5 mmol 1,3,5-trimethoxybenzene as an internal standard substance.
Crystallography
X-ray intensities of the complexes were collected on a Bruker D8 Venture CCD diffractometer with graphite-monochromated Mo-Kα (λ = 0.71073 Å) using the SMART and SAINT programs. The structures were solved by direct methods and refined on F2 by full-matrix least-squares methods with SHELXTL-2018.
Crystal data for Tb-MOF. [Tb2(sbdc)3(H2O)3], M = 1278.63, triclinic, space group P
, colorless block, a = 13.907 (1) Å, b = 14.243 (1) Å, c = 14.994 (1) Å, α = 118.151 (1), β = 99.052 (2), γ = 103.126 (2), V = 2426.3 (3) Å3, Z = 2, Dc = 1.750 g cm−3, μ(Mo-Kα) = 0.71073 Å, T = 296 (2) K. 52
159 unique reflections [Rint = 0.0603], final R1 [with I > 2σ(I)] = 0.0337, wR2 (all data) = 0.0928, GOOF = 1.079. CCDC number: 2100650.†In the structural refinement of Tb-MOF, except the partly occupied parts, the other non-hydrogen atoms were refined anisotropically and hydrogen atoms were fixed geometrically at calculated distances and allowed to ride on the parent non-hydrogen atoms. The highly disordered solvent molecules could not be located, and hence in the final refinement, the electron density was treated with the SQUEEZE routine in the PLATON program package.
Results and discussion
Three-dimensional (3D) Tb-MOF was prepared using a rigid ligand 5,5-dioxo-5H-dibenzo[b,d]thiophene-3,7-dicarboxylic acid (namely H2sbdc) and Tb(NO3)3·6H2O by using a solvothermal method to synthesize a novel material.26 Tb-MOF can be considered as a highly efficient heterogeneous catalyst which was anticipated to catalyze the cyanosilylation of aldehyde based on its Lewis acid sites and porous structure.
The single crystal X-ray diffraction data showed that the obtained Tb-MOF crystallizes in P
space group (CCDC 2100650†). The asymmetric unit of Tb-MOF contained two Tb(III) ions, three H2sbdc organic ligands, and three coordinated H2O molecules. As seen in Fig. 1a, Tb(1) and Tb(2) had two different coordination modes, of which coordinated numbers were both eight. Tb(1) was coordinated with eight oxygen atoms (Tb–O = 2.27–2.52 Å) from seven di-monodentate carboxyl groups in different ligands, and one water molecule. Tb(2) was coordinated with eight oxygen atoms (Tb–O = 2.26–2.53 Å) from four di-monodentate carboxyl groups in different ligands, one bi-dentate carboxyl group, and two water molecules, which was similar to some other works of Tb-MOFs reported before.27 Tb(1) and Tb(2) were linked through two di-monodentate carboxyl groups and on μ2-oxygen atom of the didentate carboxy group. The two adjacent Tb(1) centers were bridged by four di-monodentate carboxyl groups, forming a paddle wheel dinuclear Tb(2) core by which the two Tb(1)–Tb(2) units were linked to form a zigzag tetra-nuclear unit. And the tetra-nuclear unit was further extended by the bi-carboxyl ligands, resulting in a 3D framework having channels along the a and b direction with the area of the pore was about 7.5 × 5.0 Å2 and 9.0 × 5.5 Å2 respectively, which were available for guest accommodation and exchange. The metal centers having removable water molecules were well-positioned in the channels. The results obviously demonstrated that Tb-MOF not only had lots of open metal Tb(III) sites but also contained lots of cavities as Lewis acids sites simultaneously, resulting in its potential applications in the catalytic field. The PXRD profile of the synthesized solid sample (Fig. 2) was carried out at ambient temperature and 200 °C, respectively. The characteristic diffraction lines of prepared Tb-MOF and Tb-MOF heated to 200 °C were similar with the simulated from the single crystal data of Tb-MOF, revealing the purity and stability of the product Tb-MOF.
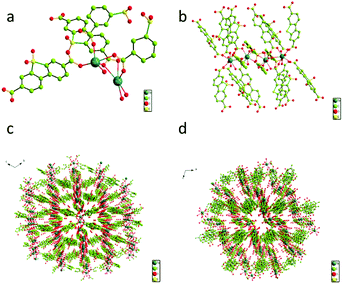 |
| Fig. 1 (a) The asymmetric unit of Tb-MOF; (b) Tb4 units in Tb-MOF; (c) view of the 3D structure along a direction; (d) view of the 3D structure along b direction. Hydrogen atoms were omitted for clarity. | |
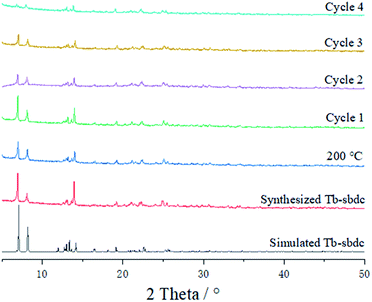 |
| Fig. 2 The PXRD spectra. (black bar) Simulated Tb-MOF; (red bar) Tb-MOF; (blue bar) Tb-MOF Heated to 200 °C; (green bar) cycle one for catalysis of m-methoxybenzaldehyde; (purple bar) cycle two for catalysis of m-methoxybenzaldehyde; (yellow bar) cycle three for catalysis of m-methoxybenzaldehyde; (light blue bar) cycle four for catalysis of m-methoxybenzaldehyde. | |
Thermogravimetric analysis (TGA) demonstrated that in the range of 25–800 °C which increased 10 °C per minute in N2 atmosphere. As obviously displayed in Fig. 3, the TGA result of the fresh synthesized Tb-MOF demonstrated a slow weight loss of 8% before 200 °C, corresponding to the loss of water and EtOH molecules. Tb-MOF became metal oxide and its structure collapsed when the temperature raised to 500 °C. It indicated that the framework of Tb-MOF was stable below 500 °C.
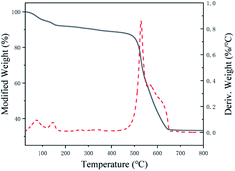 |
| Fig. 3 The TGA spectra of Tb-MOF. | |
After treating 10 mg Tb-MOF with 2′,7′-dichlorofluorescein in DCM for 24 h, the product was filtered and washed with DCM. Then HCl was used to destroy the structure of Tb-MOF so that the quantity of 2′,7′-dichlorofluorescein in the channels of Tb-MOF could be tested by UV-vis adsorption. The result in Fig. 4 showed that Tb-MOF can adsorb 6.3% 2′,7′-dichlorofluorescein of its weight, which could prove that Tb-MOF had enough pores and a good ability to adsorb small molecules.28
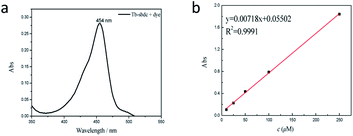 |
| Fig. 4 (a) The UV-vis absorption spectra of released 2′,7′-dichlorofluorescein from Tb-MOF; (b) the concentration of 2′,7′-dichlorofluorescein was determined by comparing the UV-vis absorption with a standard curve. | |
Since the addition of cyanide to a carbonyl compound to form a cyanohydrin was one of the fundamental C–C bond-forming reactions in organic chemistry and had frequently been at the forefront of advances in chemical transformations,29 the catalytic ability of Tb-MOF for the cyanosilylation of the aromatic aldehyde was further studied in the detail due to the uniformly dispersed Lewis acid sites and the size of its pores in the whole structure.30
Therefore, p-methoxybenzaldehyde with TMSCN catalyzed by Tb-MOF was studied in different solutions. As we could see in Table 1, Tb-MOF had no catalytic ability in EtOH for this reaction, even the mixture was stirred for 12 h. And the yield of the reaction in MeOH was much lower than those in DCM, THF, and CH3CN. Considering the stability of the MOF catalyst and the separation of the product, DCM was chosen as the solvent of the reaction for further study. Consequently, the reaction condition was optimized as the addition of 2.4 equivalent of TMSCN (Fig. S63†) and 12 h (Fig. S64†) under Ar at room temperature.
Table 1 The yields in different solutions (0.5 mmol p-methoxybenzaldehyde, 1.2 mmol TMSCN, 4 mol% Tb-MOF, in 2 mL solution, under Ar condition)
Entry |
Solvent |
Conv. (%) |
1 |
MeCN |
85 |
2 |
THE |
82 |
3 |
DCM |
78 |
4 |
MeOH |
14 |
5 |
EtOH |
0 |
Therefore, different aromatic aldehydes, which had different electronic effect, spatial effect, and fatty aldehyde were selected. Table 2 showed the results based on 12 different aldehyde substrates and trimethylsilyl cyanide in the presence of Tb-MOF. Most of them had a higher yield. Even, the yield of 1-naphthalene formaldehyde reached 77%. Table 3 showed the catalytic cyanosilylation of o-, m- and p-methoxybenzaldehyde in different conditions. The final yield of 3-methoxybenzaldehyde and 4-methoxybenzaldehyde were 85% and 81% after reacting 12 h. The reaction was monitored after reacting 4 h. The yield of o-, m-, and p-methoxybenzaldehyde was 28%, 30%, and 41%, respectively. The corresponding initial TOF was about 1.8 h−1, 1.9 h−1, and 2.6 h−1, respectively. The catalyst Tb-MOF was filtered after reacting 4 h and let the reaction continue. The yield of 4-methoxybenzaldehyde increased a little to 47% after reacting 12 h, of which the catalyst Tb-MOF was removed after reacting 4 h. The yield of this reaction was 0 without any catalyst for 12 h. The yield of the reaction catalyzed by the ligand without Tb-MOF was 0 either. The yield had no significant change when the catalyst was doubled. These consequences demonstrated that the ligand couldn't catalyze this reaction and Tb-MOF was necessary for this reaction. The Lewis acid sites in the channel of Tb-MOF could activate the substrates, which could make the reaction continue. Furthermore, as the yields of o-, m- and p-methoxybenzaldehyde after 12 h are 82%, 79%, 78%, catalyst Tb-MOF had no prominent electronic effect on this reaction. However, the yield of p-benzyloxybenzaldehyde and 4-(diethylamino)salicylaldehyde were 66% and 57%, which were lower than other substrates. It seemed that the substrates which had larger sizes didn't fit the pores of Tb-MOF well, indicating that the activation of substrates should be occurred in the pores of Tb-MOF. Table 2 also showed that all the TON of the 12 product were about 20, which were not at a high level. Consequently, 5 mmol p-methoxybenzaldehyde, 12 mmol TMSCN, and 0.2% mmol Tb-MOF with no solvent were stirred at room temperature under Ar condition. Notably, the yield of solvent free condition was about 92%, and the TON could reach about 460 with the MOF catalyst being 0.2 mol%, which demonstrated that Tb-MOF had a good ability of catalyzing cyanosilylation. The results above demonstrated that the Tb-MOF had a good ability for catalyzing most kinds of aldehydes for higher yields as a heterogeneous catalyst.
Table 2 Catalytic cyanosilylation of different substrates in the presence of Tb-MOF. Reaction conditions: (CH3)3SiCN: 1.2 mmol, aldehyde: 0.5 mmol, Tb-MOF catalysts (4 mol%), room temperature under Ar for 12 h
Table 3 Catalytic cyanosilylation o-, m- and p-methoxybenzaldehyde in different conditions
Substrate |
12 h |
4 h |
4–12 h |
Double catalyst |
Without catalyst |
Ligand |
 |
82 |
28 |
46 |
81 |
0 |
0 |
 |
79 |
30 |
43 |
83 |
0 |
0 |
 |
78 |
41 |
47 |
80 |
0 |
0 |
To test the recyclability of the catalyst Tb-MOF, four cycles of cyanosilylation reaction in DCM were performed. Tb-MOF was separated by filtration from the reaction mixture after 12 hours, washed with DCM, dried under vacuum overnight, and reused in the next reaction run. The second, the third, and the forth cycles were completed under the same condition as the first cycle. From the PXRD measurements of the recovered catalyst in Fig. 2, Tb-MOF retained its structural framework even after three cycling reaction process. However, the decrease of the PXRD could be found in the forth cycle. As shown in Fig. 5, reactions catalyzed by Tb-MOF showed a slight decrease after three cycles, which demonstrated that the heterogeneous Tb-MOF had a better advantage than the homogeneous catalyst. In the forth cycle, the yields decreased more than the first three cycles, which fitted the PXRD results. TON of o-, m-, and p-methoxybenzaldehyde for all the four cycles were 80, 74, and 69, respectively. As a result, Tb-MOF had a good ability for recyclable catalyzing cyanosilylation for at least three times without the yields' going down.
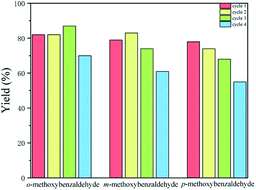 |
| Fig. 5 The yields for three cycles (0.5 mmol p-methoxybenzaldehyde, 1.2 mmol TMSCN, 0.01 mmol Tb-MOF, in 2 mL DCM at room temperature under Ar condition). | |
To test whether Tb-MOF could adsorb the substrate, 0.01 mmol Tb-MOF, 3 mmol substrate with 2 mL DCM in a 6 mL glass bottle were under continuously stirring for 24 h. Then the solid was filtered, washed by DCM and dried in vacuum. Fig. 6 was the IR spectrogram, which showed the differences between Tb-MOF and Tb-MOF with the substrate inside. The peak of the C
O bond of 2-naphthaldehyde was 1701 cm−1, while the peak moved to 1710 after 2-naphthaldehyde was treated with Tb-MOF. It demonstrated that the substrate could be absorbed by the pores of Tb-MOF and had interaction with Tb-MOF.
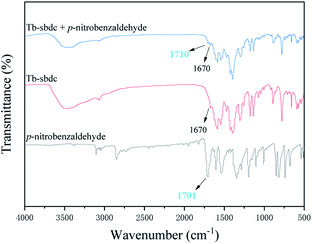 |
| Fig. 6 The IR spectra of Tb-MOF with different p-nitrobenzaldehyde. | |
To test the ability for activating the substrates of Tb-MOF, 2 mg Tb-MOF with 2-naphthaldehyde from 0 to 0.001 M was treated. A significant quenching of the fluorescence intensity could be seen in Fig. 7. It was important to note that the sorption of 2-naphthaldehyde caused the luminescence quenching. The value of I0/I and the concentration exhibited a linear relationship at a lower concentration range (0–0.5 mM), which fitted the Stern–Volmer (SV) equation, I0/I = Ksv[M] + 1 (where I0 represented the initial intensity of the peak at 615 nm, I represented the intensity at different concentrations of 2-naphthaldehyde suspension, [M] represented the concentration of 2-naphthaldehyde, and Ksv represented the quenching constant (M−1)), with a correlation coefficient (R2) of 0.98.31 The KSV value for 2-naphthaldehyde was counted to be 1.26 × 103 M−1.32 The high KSV allowed us to easily identify the existence of a small amount of aldehyde in solution. As previously mentioned, the relationship between I0/I and the concentration of substrate could be well fitted into the SV equation at lower concentrations, suggesting the interaction with quenching substrates, forming complexes and influencing the luminescence spectra of luminescent substances.33 The fluorescence titration proved that the catalysis of the reaction was in the channel of Tb-MOF, which could also recognize the substrates. The Lewis-acid sites played a dormant role in the cyanosilylation. In this case, the luminescence behavior of Tb-MOF suggested the sorption of the substrate into the MOFs and the possible interaction between the aldehyde substrate and the Tb centers. Based on the experimental results above and the works reported before, a possible reaction mechanism was presented to explain the reaction process of cyanosilylation catalyzed by Tb-MOF: the substrates were activated by the coordinatively unsaturated Tb centres to react with TMSCN, which was shown in Fig. S65.† The products were removed by other substrates, meanwhile, Tb-MOF continued to activate the substrates in the next catalytic turn.34
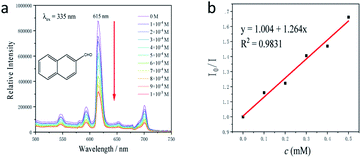 |
| Fig. 7 (a)Luminescent spectra of Tb-MOF emulsion in DCM upon addition of substrates up to 0.001 M (excited at 335 nm). (b) The plot of I0/I vs. the lower concentration (0–0.5 mM) of 2-naphthaldehyde which showed intensities at 615 nm as a function of substrates (excited at 335 nm). | |
Conclusions
In conclusion, a novel Tb(III)-based metal–organic framework was successfully synthesized, which could be considered as an efficient heterogeneous catalyst for the cyanosilylation of different aldehydes at room temperature because of its pores and the activation ability for the substrates of its Lewis acid sites. Meanwhile, Tb-MOF exhibited a high TON (TON = 460) in solvent free condition and a good recyclability for these reactions in solvent condition at least three times. Compared to other transition metal organic frameworks,24 which were designed for catalyzing cyanosilylation in solvent free condition, Tb-MOF had a higher TON. As a result, Tb-MOF was considered as a good heterogeneous catalyst for cyanosilylation and had a good prospect for further study. We hope that this work can offer a useful and meaningful method to use such ligands to synthesize MOFs for sensing or absorbing organic molecules towards environmental and catalyzing different reactions.
Conflicts of interest
There are no conflicts to declare.
Acknowledgements
We gratefully acknowledge financial support from the National Natural Science Foundation of China (U1608224).
Notes and references
-
(a) H. Raza, et al., Synthesis of a 2D copper(II)-carboxylate framework having ultrafast adsorption of organic dyes, J. Colloid Interface Sci., 2021, 602, 43–54 CrossRef CAS PubMed;
(b) Q. F. Lin, et al., Three 3D Lanthanide coordination polymers: Synthesis, luminescence and magnetic properties, J. Mol. Struct., 2021, 1234, 130167 CrossRef CAS;
(c) J. Wang, et al., Two 3D supramolecular isomeric Zn(II)-MOFs as photocatalysts for photodegradation of methyl violet dye, Dyes Pigm., 2021, 190, 109285 CrossRef CAS;
(d) J. D. Yi, et al., Conductive Two-Dimensional Phthalocyanine-based Metal–Organic Framework Nanosheets for Efficient Electroreduction of CO2, Angew. Chem., Int. Ed., 2021, 60, 2–9 CrossRef;
(e) Y. Liu, et al., The Synthesis of Hexaazatrinaphthylene-Based 2D Conjugated Copper Metal-Organic Framework for Highly Selective and Stable Electroreduction of CO2 to Methane, Angew. Chem., Int. Ed., 2021, 60, 1–8 CrossRef CAS;
(f) Y. H. Luo, et al., 2D hydrogen-bonded organic frameworks: in-site generation and subsequent exfoliation, Chem. Commun., 2021, 57, 5901–5904 RSC;
(g) Q. Bi, et al., Hierarchical core-shell 2D MOF nanosheet hybrid arrays for high-performance hybrid supercapacitors, Dalton Trans., 2021, 50, 8179–8188 RSC.
-
(a) X. Ren, et al., Diversified strategies based on nanoscale metal-organic frameworks for cancer therapy: The leap from monofunctional to versatile, Coord. Chem. Rev., 2021, 431, 213676 CrossRef CAS;
(b) L. Fan, et al., A Series of Metal-Organic Framework Isomers Based on Pyridinedicarboxylate Ligands: Diversified Selective Gas Adsorption and the Positional Effect of Methyl Functionality, Inorg. Chem., 2021, 60, 2704–2715 CrossRef CAS PubMed;
(c) M. A. Gordillo, et al., The Advent of Electrically Conducting Double-Helical Metal-Organic Frameworks Featuring Butterfly-Shaped Electron-Rich π-Extended Tetrathiafulvalene Ligands, ACS Appl. Mater. Interfaces, 2020, 12, 12955–12961 CrossRef CAS PubMed;
(d) D. Sun, et al., From Mixed-Metal MOFs to Carbon-Coated Core-Shell Metal Alloy@Metal Oxide Solid Solutions: Transformation of Co/Ni-MOF-74 to CoxNi1-x@CoyNi1-yO@C for the Oxygen Evolution Reaction, Inorg. Chem., 2017, 56, 5203–5209 CrossRef CAS PubMed;
(e) Y. F. Niu, et al., Solvent-mediated secondary building units (SBUs) diversification in a series of MnII-based metal-organic frameworks (MOFs), J. Solid State Chem., 2016, 241, 18–25 CrossRef CAS.
-
(a) J. Kim, et al., Enhancement of hydrogen storage capacity and hydrostability of metal-organic frameworks (MOFs) with surface-loaded platinum nanoparticles and carbon black, Microporous Mesoporous Mater., 2015, 202, 8–15 CrossRef CAS;
(b) J. L. C. Rowsell and O. M. Yaghi, Metal–organic frameworks: a new class of porous materials, Microporous Mesoporous Mater., 2004, 73, 3–14 CrossRef CAS.
-
(a) D. Ursueguía, et al., Metal-Organic Frameworks (MOFs) as methane adsorbents: From storage to diluted coal mining streams concentration, Sci. Total Environ., 2021, 790, 148211 CrossRef PubMed;
(b) P. A. S. Vavazos, et al., Fluorinated MIL-101 for carbon capture utilization and storage: uptake and diffusion studies under relevant industrial conditions, RSC Adv., 2021, 11, 13304–13310 RSC;
(c) C. Altintas, et al., Machine Learning Meets with Metal Organic Frameworks for Gas Storage and Separation, J. Chem. Inf. Model., 2021, 61, 2131–2146 CrossRef CAS PubMed;
(d) F. E. Chen, et al., Biocompatible metal–organic frameworks for the storage and therapeutic delivery of hydrogen sulfide, Chem. Sci., 2021, 12, 7848–7857 RSC;
(e) S. Lin, et al., An Isomeric Copper-Diisophthalate Framework Platform for Storage and Purification of C2H2 and Exploration of the Positional Effect of the Methyl Group, Eur. J. Inorg. Chem., 2021, 2070–2077 CrossRef CAS.
-
(a) J. X. Liu, et al., 3.Al-Based Metal-Organic Framework MFM-300 and MIL-160 for SO2 Capture: A Molecular Simulation Study, Fluid Phase Equilib., 2021, 536, 112963 CrossRef CAS;
(b) X. Dong, et al., Recycling Plastic Waste for Environmental Remediation in Water Purification and CO2 Capture, ACS Appl. Polym. Mater., 2020, 2, 2586–2593 CrossRef CAS;
(c) Z. Hu, et al., CO2 Capture in Metal-Organic Framework Adsorbents: An Engineering Perspective, Adv. Sustainable Syst., 2019, 3, 1800080 CrossRef;
(d) L. A. Darunte, et al., Moving Beyond Adsorption Capacity in Design of Adsorbents for CO2 Capture from Ultradilute Feeds: Kinetics of CO2 Adsorption in Materials with Stepped Isotherms, Ind. Eng. Chem. Res., 2019, 58, 366–377 CrossRef CAS.
-
(a) Y. Zeng, et al., Molecular simulations for adsorption and separation of thiophene and benzene in Cu-BTC and IRMOF-1 metal–organic frameworks, Sep. Purif. Technol., 2012, 95, 149–156 CrossRef CAS;
(b) X. Han, et al., Fine-tuning the pore structure of metal–organic frameworks by linker substitution for enhanced hydrogen storage and gas separation, CrystEngComm, 2021, 23, 3026–3032 RSC;
(c) X. P. Fu, et al., Metal-organic frameworks for C2H2/CO2 separation, Dalton Trans., 2020, 49, 16598–16607 RSC;
(d) Z. Ju, et al., Dynamic metal-organic frameworks for the separation of hydrogen isotopes, Dalton Trans., 2020, 49, 16617–16622 RSC;
(e) Y. Zhao, et al., Design of thin and tubular MOFs-polymer mixed matrix membranes for highly selective separation of H2 and CO2, Sep. Purif. Technol., 2019, 220, 197–205 CrossRef CAS;
(f) S. Yang, et al., Elucidating Charge Separation Dynamics in a Hybrid Metal-Organic Framework Photocatalyst for Light-Driven H2 Evolution, J. Phys. Chem. C, 2018, 122, 3305–3311 CrossRef CAS.
-
(a) Y. B. N. Tran, et al., Series of M-MOF-184 (M = Mg, Co, Ni, Zn, Cu, Fe) Metal-Organic Frameworks for Catalysis Cycloaddition of CO2, Inorg. Chem., 2020, 59, 16747–16759 CrossRef CAS PubMed;
(b) U. Patel, et al., Zn(II)/Cd(II)-Based Metal-Organic Frameworks as Bifunctional Materials for Dye Scavenging and Catalysis of Fructose/Glucose to 5-Hydroxymethylfurfural, Inorg. Chem., 2021, 60, 9181–9191 CrossRef CAS PubMed;
(c) R. R. Tuttle, Copper Metal-Organic Framework Surface Catalysis: Catalyst Poisoning, IR Spectroscopic, and Kinetic Evidence Addressing the Nature and Number of the Catalytically Active Sites En Route to Improved Applications, ACS Appl. Mater. Interfaces, 2020, 12, 39043–39055 CrossRef CAS PubMed;
(d) Y. Gao, et al., In Situ Synthesis of Defect-Engineered MOFs as a Photoregenerable Catalytic Adsorbent: Understanding the Effect of LML, Adsorption Behavior, and Photoreaction Process, ACS Appl. Mater. Interfaces, 2020, 12, 12706–12716 CrossRef CAS PubMed;
(e) J. N. Hall and P. Bollini, Metal-Organic Framework MIL-100 Catalyzed Acetalization of Benzaldehyde with Methanol: Lewis or Brønsted Acid Catalysis?, ACS Catal., 2020, 10, 3750–3763 CrossRef CAS;
(f) C. Wang, et al., Metal-Organic Frameworks in Solid-Gas Phase Catalysis, ACS Catal., 2019, 9, 130–146 CrossRef CAS;
(g) L. Yang, et al., A Versatile Porous Silver-Coordinated Material for the Heterogeneous Catalysis of Chemical Conversion with Propargylic Alcohols and CO2, Nanomaterials, 2019, 1566 CrossRef PubMed.
-
(a) Y. Shi, et al., A novel terbium metaleorganic framework for luminescence sensing of pyridine: Synthesis, structure, selectivity, sensitivity and recyclability, J. Rare Earths, 2020, 38, 1231–1236 CrossRef CAS;
(b) G. E. Gomez and F. Ronocaroli, Photofunctional metal-organic framework thin films for sensing, catalysis and device fabrication, Inorg. Chim. Acta, 2020, 513, 119926 CrossRef CAS;
(c) É. Whenlan, et al., Tuning photoactive metal–organic frameworks for luminescence and photocatalytic applications, Coord. Chem. Rev., 2021, 437, 213757 CrossRef.
-
(a) S. Pandey, et al., Electronic structures and magnetism of Zr-, Th-, and U-based metal-organic frameworks (MOFs) by density functional theory, Comput. Mater. Sci., 2020, 184, 109903 CrossRef CAS;
(b) M. N. Ahamad, et al., Cu(II) MOFs Based on Bipyridyls: Topology, Magnetism, and Exploring Sensing Ability toward Multiple Nitroaromatic Explosives, ACS Omega, 2019, 4, 7738–7749 CrossRef CAS PubMed;
(c) K. Iman, et al., Topology, magnetism and dye adsorption properties of metal organic frameworks (MOFs) synthesized from bench chemicals, CrystEngComm, 2019, 21, 5299–5309 RSC;
(d) C. Qiao etal, Pore-size-tuned host–guest interactions in Co-MOFs via in situ microcalorimetry: adsorption and magnetism, J. Mater. Chem. C, 2017, 5, 1064–1073 RSC;
(e) S. I. Vasylevs’kyy, et al., 1,2,4-Triazolyl-Carboxylate-Based MOFs Incorporating Triangular Cu(II)-Hydroxo Clusters: Topological Metamorphosis and Magnetism, Inorg. Chem., 2014, 53, 3642–3654 CrossRef PubMed;
(f) N. Zhang, et al., Solvent-controlled structural diversity observed in three Cu(II) MOFs with a 2,2’-dinitro-biphenyl-4,4’-dicarboxylate ligand: synthesis, structures and magnetism, RSC Adv., 2015, 5, 70772–70780 RSC.
-
(a) M. Abedi, et al., Hierarchical mesoporous zinc-imidazole dicarboxylic acid MOFs: Surfactant-directed synthesis, pH-responsive degradation, and drug delivery, Int. J. Pharm., 2021, 602, 120685 CrossRef CAS PubMed;
(b) A. Ringaci, et al., Metal-organic frameworks for simultaneous gene and small molecule delivery in vitro and in vivo, Chem. Eng. J., 2021, 418, 129386 CrossRef CAS;
(c) M. N. Hasan, et al., Sensitization of nontoxic MOF for their potential drug delivery application against microbial infection, Inorg. Chim. Acta, 2021, 523, 120381 CrossRef CAS;
(d) S. Sharma, et al., Copper-Gallic Acid Nanoscale Metal-Organic Framework for Combined Drug Delivery and Photodynamic Therapy, ACS Appl. Bio Mater., 2019, 2, 2092–2101 CrossRef CAS;
(e) Y. Li, et al., MOF nanoparticles with encapsulated dihydroartemisinin as a controlled drug delivery system for enhanced cancer therapy and mechanism analysis, J. Mater. Chem. B, 2020, 8, 7382–7389 RSC;
(f) J. Zhao, et al., Development of a Tau-Targeted Drug Delivery System Using a Multifunctional Nanoscale Metal-Organic Framework for Alzheimer's Disease Therapy, ACS Appl. Mater. Interfaces, 2020, 12, 44447–44458 CrossRef CAS PubMed.
-
(a) Q. Tang, et al., Color Tuning and White Light Emission via in Situ Doping of Luminescent Lanthanide Metal-Organic Frameworks, Inorg. Chem., 2014, 53, 289–293 CrossRef CAS PubMed;
(b) Q. Yao, et al., Series of Highly Stable Isoreticular Lanthanide Metal-Organic Frameworks with Expanding Pore Size and Tunable Luminescent Properties, Chem. Mater., 2015, 27, 5332–5339 CrossRef CAS.
-
(a) R. M. Cedeno, et al., Bandgap Modulation in Zr-Based Metal-Organic Frameworks by Mixed-Linker Approach, Inorg. Chem., 2021, 60, 8908–8916 CrossRef CAS PubMed;
(b) É. Whelan, et al., Tuning photoactive metal-organic frameworks for luminescence and photocatalytic applications, Coord. Chem. Rev., 2021, 437, 213757 CrossRef.
-
(a) M. K. Matikolaei and E. Binaeian, Boosting Ammonia Uptake within Metal-Organic Frameworks by Anion Modulating Strategy, ACS Appl. Mater. Interfaces, 2021, 13, 27159–27168 CrossRef PubMed;
(b) S. E. Ashbrook, et al., 17O NMR spectroscopy of crystalline microporous materials, Chem. Sci., 2021, 12, 5016–5036 RSC.
- K. Y. A. Lin and F. K. Hsu, Magnetic iron/carbon nanorods derived from a metal organic framework as an efficient heterogeneous catalyst for the chemical oxidation process in water, RSC Adv., 2015, 5, 50790–50800 RSC.
-
(a) Q. G. Zhai, et al., Design of Pore Size and Functionality in Pillar-Layered Zn-TriazolateDicarboxylate Frameworks and Their High CO2/CH4 and C2 Hydrocarbons/CH4 Selectivity, Inorg. Chem., 2015, 54, 9862–9868 CrossRef CAS PubMed;
(b) T. He, et al., Competitive Coordination Strategy to Finely Tune Pore Environment of Zirconium-Based Metal-Organic Frameworks, ACS Appl. Mater. Interfaces, 2017, 9, 22732–22738 CrossRef CAS PubMed;
(c) S. A. A. Razavi, et al., Improvement of Methane-Framework Interaction by Controlling Pore Size and Functionality of Pillared MOFs, Inorg. Chem., 2017, 56, 2581–2588 CrossRef CAS PubMed.
-
(a) K. Roztoki, et al., Combining In Situ Techniques (XRD, IR, and 13C NMR) and Gas Adsorption Measurements Reveals CO2-Induced Structural Transitions and High CO2/CH4 Selectivity for a Flexible Metal-Organic Framework JUK-8, ACS Appl. Mater. Interfaces, 2021, 13, 28503–28513 CrossRef PubMed;
(b) A. Schlachter, et al., Porphyrin-Containing MOFs and COFs as Heterogeneous Photosensitizers for Singlet Oxygen-Based Antimicrobial Nanodevices, ACS Appl. Mater. Interfaces, 2021, 13, 26651–26672 CrossRef CAS PubMed;
(c) C. Yue, et al., Study on the Stability, Evolution of Physicochemical Properties, and Postsynthesis of Metal-Organic Frameworks in Bubbled Aqueous Ozone Solution, ACS Appl. Mater. Interfaces, 2021, 13, 26264–26277 CrossRef CAS PubMed.
- Y. Takashima, et al., In Situ Generation and Immobilization of an Activated Rh Complex Catalyst in a Metal-Organic Framework for Hydrogenation at Low H2 Pressure, Eur. J. Inorg. Chem., 2017, 5344–5349 CrossRef CAS.
-
(a) W. Cao, et al., Lanthanide metal-organic frameworks with nitrogen functional sites for the highly selective and sensitive detection of NADPH, Chem. Commun., 2020, 56, 10851–10854 RSC;
(b) H. Y. Li, et al., Functional metal-organic frameworks as effective sensors of gases and volatile compounds, Chem. Soc. Rev., 2020, 4, 6364–6401 RSC.
-
(a) Y. Liu, et al., Porous and Robust Lanthanide Metal-Organoboron Frameworks as Water Tolerant Lewis Acid Catalysts, Inorg. Chem., 2013, 52, 10286–10291 CrossRef CAS PubMed;
(b) C. Pagis, et al., Lanthanide-Based Metal Organic Frameworks: Synthetic Strategies and Catalytic Applications, ACS Catal., 2016, 6, 6063–6072 CrossRef CAS;
(c) A. Bavykina, et al., Metal-Organic Frameworks in Heterogeneous Catalysis: Recent Progress, New Trends, and Future Perspectives, Chem. Rev., 2020, 120, 8468–8535 CrossRef CAS PubMed.
-
(a) Z. Li, et al., Aperture-Opening Encapsulation of a Transition Metal Catalyst in a Metal-Organic Framework for CO2 Hydrogenation, J. Am. Chem. Soc., 2018, 140, 8082–8085 CrossRef CAS PubMed;
(b) Y. Chen, et al., Aluminum metal-organic framework as a new host for preparation of encapsulated metal complex catalysts, Catal. Commun., 2015, 64, 91–95 CrossRef CAS.
- G. Chen, et al., Fluorescence and electrochemical assay for bimodal detection of lead ions based on Metal–Organic framework nanosheets, Talanta, 2021, 232, 122405 CrossRef CAS PubMed.
- G. K. S. Prakash, H. Vaghoo, C. Panja, V. Surampudi, R. Kultyshev, T. Mathew and G. A. Olah, Effect of carbonates/phosphates as nucleophilic catalysts in dimethylformamide for efficient cyanosilylation of aldehydes and ketones, Proc. Natl. Acad. Sci. U. S. A., 2007, 104(9), 3026 CrossRef PubMed.
- M. North, D. L. Usanov and C. Young, Lewis Acid Catalyzed Asymmetric Cyanohydrin Synthesis, Chem. Rev., 2008, 108, 5146 CrossRef CAS PubMed.
- Z. Zhang, J. Chen, Z. Bao, G. Chang, H. Xing and Q. Ren, Insight into the catalytic properties and applications of metal–organic frameworks in the cyanosilylation of aldehydes, RSC Adv., 2015, 5, 79355–79360 RSC.
-
(a) W. Xu, et al., A Robust TbIII-MOF for Ultrasensitive Detection of Trinitrophenol: Matched Channel Dimensions and Strong Host-Guest Interactions, Inorg. Chem., 2019, 58, 8198–8207 CrossRef PubMed;
(b) P. Wu, et al., Lanthanide-Based Metal-Organic Frameworks Containing “V-Shaped” Tetracarboxylate Ligands: Synthesis, Crystal Structures, “Naked-Eye” Luminescent Detection, and Catalytic Properties, Inorg. Chem., 2020, 59, 264–273 CrossRef CAS PubMed;
(c) Y. Zhang, et al., Robust Bifunctional Lanthanide Cluster Based Metal-Organic Frameworks (MOFs) for Tandem Deacetalization-Knoevenagel Reaction, Inorg. Chem., 2018, 57, 2193–2198 CrossRef CAS PubMed.
-
(a) E. Neofotistou, et al., Unprecedented Sulfone-Functionalized Metal-Organic Frameworks and Gas-Sorption Properties, Chem.–Eur. J., 2009, 15, 4523–4527 CrossRef CAS PubMed;
(b) K. Kanaizuka, et al., Design and Characterization of a Polarized Coordination Polymer of a Zinc(II) Biphenyldicarboxylate Bearing a Sulfone Group, Chem. Lett., 2010, 39, 2829 CrossRef.
- H. M. Chai, et al., A Multifunctional Tb-MOF Detector for H2O2, Fe3+, Cr2O72-, and TPA Explosive Featuring Coexistence of Binuclear and Tetranuclear Clusters, ACS Omega, 2020, 5, 33039–33046 CrossRef CAS PubMed.
-
(a) X. Zhao, et al., The application of MOFs-based materials for antibacterials adsorption, Coord. Chem. Rev., 2021, 440, 213970 CrossRef CAS;
(b) P. Brandt, et al., Zirconium and Aluminum MOFs for Low-Pressure SO2 Adsorption and Potential Separation: Elucidating the Effect of Small Pores and NH2 Groups, ACS Appl. Mater. Interfaces, 2021, 13, 29137–29149 CrossRef CAS PubMed.
-
(a) D. Dang, P. Wu, C. He, Z. Xie and C. Duan, J. Am. Chem. Soc., 2010, 132, 14321 CrossRef CAS PubMed;
(b) M. North, D. L. Usanov and C. Young, Chem. Rev., 2008, 108, 5146 CrossRef CAS PubMed.
-
(a) P. Y. Wu, et al., Luminescent Sensing and Catalytic Performances of a Multifunctional Lanthanide-Organic Framework Comprising a Triphenylamine Moiety, Adv. Funct. Mater., 2011, 21, 2788–2794 CrossRef CAS;
(b) S. Horike, et al., Size-Selective Lewis Acid Catalysis in a Microporous Metal-Organic Framework with Exposed Mn2+ Coordination Sites, J. Am. Chem. Soc., 2008, 130, 5854–5855 CrossRef CAS PubMed;
(c) L. M. Aguirre-Diaz, et al., Toward understanding the structure-catalyst activity relationship of new indium MOFs as catalysts for solvent-free ketone cyanosilylation, RSC Adv., 2015, 5, 7058–7065 RSC.
- Z. Yao, G. Li, J. Xu, T. Hu and X. Bu, A Water-Stable Luminescent Zn(II) Metal-Organic Framework as Chemosensor for High-Efficiency Detection of Cr(VI)-Anions (Cr2O72- and CrO42-) in Aqueous Solution, Chem.–Eur. J., 2018, 24, 3192–3198 CrossRef CAS PubMed.
-
(a) Z. Hu, et al., Effective detection of mycotoxins by a highly luminescent metal-organic framework, J. Am. Chem. Soc., 2015, 137, 16209–16215 CrossRef CAS PubMed;
(b) N. D. Rudd, et al., Highly Efficient Luminescent Metal-Organic Framework for the Simultaneous Detection and Removal of Heavy Metals from Water, ACS Appl. Mater. Interfaces, 2016, 8, 30294–30303 CrossRef CAS PubMed;
(c) H. Xu, et al., Metalorganic framework nanosheets for fast response and highly sensitive luminescent sensing of Fe3+, J. Mater. Chem. A, 2016, 4, 10900–10905 RSC.
-
(a) S. Zhu and B. Yan, A novel sensitive fluorescent probe of S2O82-and Fe3+ based on covalent post-functionalization of a zirconium(IV) metal-organic framework, Dalton Trans., 2018, 47, 11586–11592 RSC;
(b) J. Dong, et al., Metal-Organic Frameworks with Tb4
Clusters as Nodes: Luminescent Detection of Chromium(VI) and Chemical Fixation of CO2, Inorg. Chem., 2017, 56, 6244–6250 CrossRef CAS PubMed;
(c) R. Goswami, et al., Guest-Induced Ultrasensitive Detection of Multiple Toxic Organics and Fe3+ Ions in a Strategically Designed and Regenerative Smart Fluorescent Metal-Organic Framework, ACS Appl. Mater. Interfaces, 2019, 11, 9042–9053 CrossRef CAS PubMed;
(d) C. Gogoi, M. Yousufuddin and S. Biswas, A new 3D luminescent Zn(II)-organic framework containing a quinoline-2,6-dicarboxylate linker for the highly selective sensing of Fe(III) ions, Dalton Trans., 2019, 48, 1766–1773 RSC.
-
(a) G. Kumar and R. Gupta, Inorg. Chem., 2013, 52, 10773 CrossRef CAS PubMed;
(b) J. A. Seckar and J. S. Thayer, Inorg. Chem., 1976, 15, 501 CrossRef CAS.
Footnote |
† Electronic supplementary information (ESI) available: Experimental and catalysis details, characterization data for the new compounds. CCDC 2100650. For ESI and crystallographic data in CIF or other electronic format see DOI: 10.1039/d1ra06533e |
|
This journal is © The Royal Society of Chemistry 2021 |
Click here to see how this site uses Cookies. View our privacy policy here.