DOI:
10.1039/D0SC06557A
(Review Article)
Chem. Sci., 2021,
12, 3130-3145
Intelligent stimuli-responsive nano immunomodulators for cancer immunotherapy
Received
30th November 2020
, Accepted 1st February 2021
First published on 10th February 2021
Abstract
Cancer immunotherapy is a revolutionary treatment method in oncology, which uses a human's own immune system against cancer. Many immunomodulators that trigger an immune response have been developed and applied in cancer immunotherapy. However, there is the risk of causing an excessive immune response upon directly injecting common immunomodulators into the human body to trigger an immune response. Therefore, the development of intelligent stimuli-responsive immunomodulators to elicit controlled immune responses in cancer immunotherapy is of great significance. Nanotechnology offers the possibility of designing smart nanomedicine to amplify the antitumor response in a safe and effective manner. Progress relating to intelligent stimuli-responsive nano immunomodulators for cancer immunotherapy is highlighted as a new creative direction in the field. Considering the clinical demand for cancer immunotherapy, we put forward some suggestions for constructing new intelligent stimuli-responsive nano immunomodulators, which will advance the development of cancer immunotherapy.
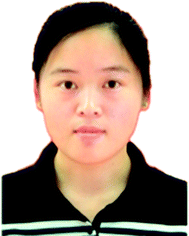 Yanhua Li | Yanhua Li is a PhD student at the Shandong Normal University. Her current research focuses on tumor-microenvironment-responsive nanomedicine for drug delivery systems and cancer immunotherapy. |
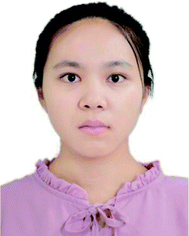 Xia Zhang | Xia Zhang is a Master's student at the Shandong Normal University. Her current research focuses on nanomedicine for drug delivery systems and cancer therapy. |
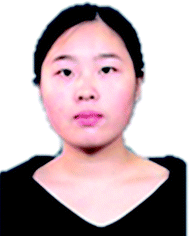 Xiaohan Liu | Xiaohan Liu is a PhD student at the Shandong Normal University. Her current research focuses on the detection of tumor markers and cancer immunotherapy. |
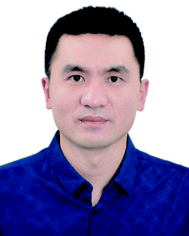 Wei Pan | Wei Pan is an associate professor at the Shandong Normal University. His current research focuses on the detection of tumor markers and the development of drug delivery systems. He has published more than 50 papers in SCI journals, such as Adv. Funct. Mater., Chem. Sci. Anal. Chem., and Chem. Commun. |
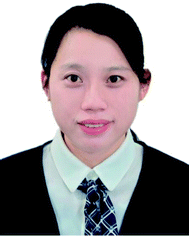 Na Li | Na Li is currently a professor at Shandong Normal University (SDNU). She received her bachelor's degree in chemistry in 2004 and a Ph.D. in physical chemistry in 2009 from Shandong University. Then she continued her research at McMaster University as a postdoctoral research fellow from 2009 to 2010 and joined SDNU in 2010. Her main research interests are advanced nanomaterials for cancer theranostics. |
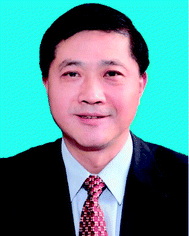 Bo Tang | Prof. Bo Tang received his Ph.D. from Nankai University in 1994. He is currently a professor in Analytical Chemistry at Shandong Normal University (SDNU) and was president of SDNU (2014–2018). He won the National Fund for Outstanding Young Scientists in 2007 and chief scientist for 973 Program in 2012. He has published more than 300 journal articles, reviews, and book chapters. His research interests are in the field of biological chemistry, especially the accurate diagnosis of cancer and efficient cancer therapeutics. |
1. Introduction
Cancer is derived from the mutation of normal cells and results in severe mortality worldwide.1–3 Once the mutant cells cannot be detected and destroyed by the immune system in the early stages, cells will keep undergoing uncontrolled cell division to invade and destroy adjacent tissues and organs. A variety of treatments have been flourishing in human anticancer therapy.4–7 Among them, cancer immunotherapy has become the hope for curing cancer because the most powerful forces against cancer are not from drugs, surgery or radiation but from a person's own immune system.8,9
Cancer immunotherapy has achieved significant therapeutic effects against cancers in recent years.10–13 The 2018 Nobel Prize in Physiology or Medicine was awarded jointly to James P. Allison and Tasuku Honjo for their contributions to cancer immunotherapy by inhibition of negative immune regulation.14,15 Various cancer immunotherapy strategies that can prime antitumor immune responses have been designed to promote systemic immune surveillance and further eliminate local and distant tumors.16–19 In addition, the long-term immune memory established by immunotherapy has the ability to protect the body from cancer recurrence.20–22 Many immunomodulators that aim to trigger an immune response have been applied in cancer immunotherapy over the past few years. For instance, Toll-like receptor (TLR) agonists, as vital immunomodulators, have been used to activate the Toll receptors of immune cells, which further primed the immune response for fighting diseases. However, systemic exposure to Toll-like receptor agonists can certainly activate the immune cells whether the body is diseased or not, which would cause an inevitably excessive immune response and do harm to normal tissue. Therefore, it is risky to cause certain side effects by directly injecting the common immunomodulators into the human body to enhance specific anti-tumor effects.23–26 Furthermore, the development of intelligent stimuli-responsive immunomodulators to elicit controlled immune responses for cancer immunotherapy is of great significance to reduce unwanted side effects. Nanotechnology offers the possibility to prepare smart nanomedicine to amplify the antitumor response in a safe and effective manner. Progress in intelligent stimuli-responsive nano immunomodulators for cancer immunotherapy is highlighted as a new creative direction in the field.
In this review, we focus on introducing cancer cells and their tumor microenvironment, as well as intelligent stimuli-responsive nano immunomodulators for bio-applications in cancer immunotherapy. The types of response conditions in the tumor microenvironment are summarized (Fig. 1). Considering the demand for cancer immunotherapy in clinic, we also put forward some suggestions for constructing new intelligent stimuli-responsive nano immunomodulators, which will advance the development of cancer immunotherapy to enhance specific anti-tumor effects with milder side effects.
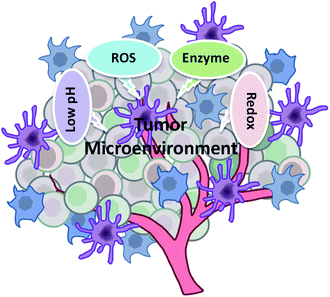 |
| Fig. 1 A summary of the types of response conditions in the tumor microenvironment. | |
2. Cancer cells and the tumor microenvironment
Cancer cells and their microenvironment are a functional entity, in which the cancer cells serve as “seeds” and the microenvironment as the “soil”. They coevolve and influence each other to promote the occurrence and growth of tumors.27–29 This complex integrated system is known as the tumor microenvironment and can be divided into the immune microenvironment dominated by immune cells and the non-immune microenvironment dominated by fibroblasts.30 Additionally, the tumor microenvironment, which has been recognized as a key contributor for cancer metastasis and drug resistance, plays a vital role in the diagnosis, therapy and prognosis of tumors.31,32 The characteristics of the tumor microenvironment are described in detail as below.
2.1. Acidity
Studies have demonstrated that the tumor microenvironment is more acidic than normal tissue, which is a result of the special structure and metabolism of tumors.32,33 In detail, cancer cells grow faster than normal cells with a high glucose uptake and active glycolysis, which induces the production and accumulation of lactic acid in the tumor microenvironment.34 Moreover, the excess lactic acid cannot be cleared due to the abnormal blood vessels and poor lymphatic drainage of the tumor,35 which further increases the accumulation of acidity in the tumor microenvironment. On the other hand, CO2 from respiration is catalyzed to H2CO3 by the carbonic anhydrase overexpressed in tumors, which is another source of acidification in the tumor microenvironment.36 As a critical characteristic of the tumor microenvironment, low pH provides a favorable condition for tumor growth and affects the immune surveillance of cancer cells, which may result in the immune escape of the tumor.
2.2 Higher levels of reactive oxygen species (ROS)
ROS are crucial molecules during the occurrence and development of cancer.37 Most cancer cells produce higher levels of ROS than normal cells through the pathways involving mitochondrial respiratory chain (MRC) and NADPH (nicotinamide adenine dinucleotide phosphate) oxidase.38 In addition, the changes of the genetic molecules and energy metabolism patterns in cancer cells can also promote the production of ROS.39,40
2.3 Overexpressed enzymes
Many enzymes, such as common matrix metalloproteinases (MMPs) and hyaluronidase (HAase), are overexpressed in the tumor microenvironment. MMPs are a class of proteases that can degrade the extracellular matrix with the assistance of Ca2+, Zn2+ and other metal ions as cofactors.41 The expression of MMPs is regulated by various cytokines and growth factors at the transcriptional level, and the activity can be activated by precursor zymogens or inhibited by tissue inhibitors of metalloproteinases (TIMPs).42,43 Due to the different scenarios of the above factors, MMPs basically maintain a low expression level and weak activity in normal tissue, and excessive accumulation and potent activity in tumor tissue. Therefore, strategies of integrating MMP-cleavable peptide sequences into nanocarriers have been extensively researched. HAase is another enzyme that is overexpressed in the tumor microenvironment and is associated with tumor development and metastasis.44 The expression level of HAase in metastasis is significantly higher than that in primary tumors, indicating that it is related to the deterioration of tumors.45 HAase has the capability to specifically hydrolyze hyaluronic acid (HA) in the extracellular matrix to regulate proliferation and metastasis of cancer cells. Hence, HAase can be used as a potential therapeutic marker and target.
2.4 Overexpressed glutathione (GSH)
GSH plays an important role in regulating the redox balance in organisms by converting between the oxidation and reduction state in vivo.46 The GSH concentration in cancer cells is as high as 0.5–10 mM, four times higher than that of normal cells,47 due to the fact that excessive ROS is needed to be consumed by GSH to avoid damage to cancer cells. So rapid depletion of GSH can enhance the effect of ROS-mediated antitumor immunotherapy. Additionally, the disulfide bond48 is the most common bond that reacts with GSH, which is always introduced into nanocarriers by physical or chemical methods to format the stimuli-responsive drug delivery system.
3. Intelligent stimuli-responsive nano immunomodulators
To avoid an excessive immune response of the body, intelligent stimuli-responsive immunomodulators have been developed to elicit controlled immune responses. Considering the characteristics of the tumor microenvironment, immunomodulators that response to tumor-specific substances are apt to enhance the controlled and specific anti-tumor effects. In particular, multifunctional nanomaterials offer the possibility to prepare smart nanomedicine in a safe and effective manner. As a consequence, progress in intelligent stimuli-responsive nano immunomodulators for cancer immunotherapy is highlighted in this review (Table 1).
Table 1 A summary of the stimuli-responsive systems mentioned in this review
Type |
The released immunomodulators |
Type of tumor treated |
Method to achieve responsivity to stimuli |
Ref. |
pH-Cleavable responsive systems |
HAase |
Mastadenoma |
Introduction of pH-labile group, 3-(bromomethyl)-4-methyl-2,5-furandione |
49
|
Antigens and adjuvants |
Mastadenoma and melanoma |
Introduction of pH-cleavable bond, hydrazone bond |
50
|
Protein vaccine |
— |
Introduction of pH-sensitive group, poly(acyclic orthoester)s |
51
|
Dendrigraft poly-L-lysines and zoledronic acid |
Mastadenoma |
Introduction of pH-responsive linker, 1,6-bis(4-formylbenzoyloxy) hexane |
52
|
TLR 7/8 agonist |
— |
Introduction of pH-cleavable bond, ketal bond |
53
|
DNA vaccine and DOX |
Melanoma |
Introduction of pH-responsive group, piperazine and diethylenetriamine |
54
|
PD-L1-siRNA and photosensitizers |
Melanoma |
Introduction of pH-cleavable bond, amide bond |
55
|
Catalase and Ce6 |
Mastadenoma |
Introduction of pH-sensitive group, 2,3-dimethylmaleic anhydride |
56
|
TLR 7/8 agonist |
Mastadenoma |
Introduction of pH-cleavable bond, benzoic-imine bond |
57
|
pH-Protonated responsive systems |
PD-L1-siRNA and photosensitizers |
Melanoma |
Introduction of acid-protonated group, tertiary amines |
58
|
Pt prodrug and BLZ-945 |
Mastadenoma |
Introduction of pH-protonated group, polyamidoamine |
59
|
MEK inhibitors |
Melanoma |
Introduction of acid-protonated group, polyacrylic acid |
60
|
pH-Soluble responsive systems |
Ce6 and DOX |
Mastadenoma |
Introduction of acid-soluble inorganic material, MnO2 |
61
|
PI3Kγ inhibitors |
Mastadenoma |
Introduction of acid-soluble inorganic material, MnO2 |
62
|
Adjuvants and IDOi |
Mastadenoma and melanoma |
Introduction of pH-soluble inorganic material, CaCO3 |
63
|
Antigens |
— |
Introduction of pH-soluble inorganic material, NH4HCO3 |
64
|
Other pH-responsive systems |
Antibodies or the Fc segments |
Mastadenoma and melanoma |
Introduction of the pH low insertion peptide |
65
|
Endogenous ROS-responsive systems |
Anti-PD-L1 and IDOi |
Melanoma |
Introduction of H2O2-sensitive sulfoether group |
66
|
Anti-PD-1 and anti-CD47 |
Melanoma |
Introduction of the ROS-responsive cross-linker |
67
|
Antigens |
— |
Introduction of H2O2-responsive peroxalate ester bond |
68
|
Antigens |
— |
Introduction of H2O2-responsive peroxalate ester bond |
69
|
Exogenous ROS-responsive systems |
IDOi |
Mastadenoma |
Introduction of a singlet oxygen (1O2) cleavable linker |
70
|
Ce6 and sorafenib |
Mastadenoma |
Introduction of the ROS-labile thioketal |
71
|
Ce6 and DOX |
Mastadenoma |
Introduction of the ROS-sensitive thioketal |
72
|
MMP-responsive systems |
Anti-PD-1 and adjuvants |
Melanoma |
Introduction of the MMPs-responsive TGMS with ester bonds |
73
|
DOX and Pd NPs |
Colorectal cancer |
Introduction of MMP-responsive TGMS |
74
|
DOX |
Mastadenoma |
Introduction of MMP-2-sensitive PEG2000-peptide |
75
|
ICG and IDOi |
Melanoma |
Introduction of the MMP-2-cleavable peptide PVGLIG |
76
|
HAase-responsive systems |
Anti-PD-1 and IDOi |
Melanoma |
Use HAase-sensitive HA to modify IDOi |
77
|
Anti-PD-L1 and Ce6 |
Melanoma |
Use HAase-sensitive HA to conjugate Ce6 |
78
|
Granzyme B |
Mastadenoma |
PMPC-modified HA as nanocarriers can be degraded by HAase |
79
|
GSH-responsive systems |
Photosensitizers and IDOi |
Mastadenoma |
Introduction of GSH-responsive disulfide bond into liposomes |
80
|
Dihydroartemisinin and oxaliplatin |
Colorectal cancer |
Introduction of GSH-sensitive disulfide bond |
81
|
ER-targeted photosensitizers |
Mastadenoma |
Introduction of GSH-responsive disulfide bond |
82
|
Antigens and adjuvants |
— |
Use of disulfide bond to attach the antigens to the MOF |
83
|
IDOi and DOX |
Mastadenoma |
Introduction of GSH-responsive disulfide bond into nanoparticles |
84
|
pH + enzyme responsive systems |
IDOi and DPPA-1 |
Melanoma |
Introduction of pH-protonated DEAP and peptide substrate of MMP-2 |
85
|
pH + GSH responsive systems |
DOX |
Triple negative breast cancer |
Introduction of acid-protonated Bz-COOH and GSH-responsive Fc+ |
86
|
IDOi and curcumin |
Melanoma |
Introduction of pH-cleavable amide bond and GSH-responsive disulfide bond |
87
|
IDOi and oxaliplatin |
Breast and colorectal cancer |
Introduction of acid-soluble amide bond and GSH-responsive disulfide bond |
88
|
GSH + ROS responsive systems |
Oxaliplatin, photosensitizers and IDOi |
Mastadenoma |
Introduction of ROS-responsive thioketal bond and GSH-sensitive disulfide bond |
89
|
pH + ROS responsive systems |
Plasmid DNA |
Melanoma |
Introduction of pH-responsive cis aconitic anhydride and H2O2-cleavable HPBA |
90
|
Anti-PD-1 and zebularine |
Melanoma |
Introduction of acid-soluble CaCO3 NPs and ROS-sensitive TSPBA linker |
91
|
— |
Breast and colorectal cancer |
Introduction of pH-sensitive acetal bond and H2O2-responsive 4-formylphenylborate pinacol ester |
92
|
GSH + enzyme responsive systems |
DOX and IDOi |
Glioblastoma |
Introduction of GSH-responsive disulfide bond and enzyme-sensitive KDEVD peptide |
93
|
Photosensitizers and IDOi |
Colorectal cancer |
Introduction of GSH-cleavable disulfide bond and HAase-responsive HA |
94
|
Photosensitizers and IDOi |
Breast and colorectal cancer |
Introduction of GSH-sensitive disulfide bond and MMP-2-activatable peptide |
95
|
ROS + enzyme responsive systems |
Cinnamaldehyde, paclitaxel and anti-PD-L1 peptide |
Mastadenoma |
Introduction of ROS-responsive thioketal linker and HAase-sensitive HA |
96
|
pH + GSH + enzyme responsive systems |
Photosensitizers and IDOi |
Colorectal cancer |
Introduction of MMP-responsive peptide, GSH-sensitive disulfide bond and pH-activated 2-(diisopropylamino) ethyl methacrylate |
97
|
3.1 pH-Responsive nano immunomodulators
3.1.1 pH-Responsive nano immunomodulators with acid-cleavable groups.
For the weakly acidic environment of the tumor microenvironment, many pH-responsive nano immunomodulators with acid-cleavable groups have been developed to trigger the tumor-specific immune response. For instance, Liu et al.49 designed a nanomedicine with HAase linked to dextran (DEX) with a pH-labile bond, 3-(bromomethyl)-4-methyl-2,5-furandione, to enhance photodynamic-immunotherapy (Fig. 2A). Because of the acidic environment, the HAase was released to decompose HA in the dense extracellular matrix, increasing the subsequent input of photosensitizer and enhancing the cancer immunotherapy. Xing et al.50 utilized an acid-activatable polymer with a common pH-labile bond, the hydrazone bond, to present antigens and adjuvants to antigen-presenting cells (APCs) for eliciting tumor-specific immunity. In another study, Guo and co-workers51 developed acid-labile nanoparticles with the acid-sensitive poly(acyclic orthoester)s to specifically release a protein vaccine to enhance the immune responses. In addition, Jiang et al.52 designed size-variable nanoparticles to mediate the polarization of the macrophage to reshape the tumor immune microenvironment for enhanced immunotherapy (Fig. 2B). In these nanoparticles, the dendritic poly-L-lysines acted as the basic unit and were integrated with the pH-responsive 1,6-bis(4-formylbenzoyloxy) hexane and a macrophage polarization inducer (zoledronic acid). The acidic tumor microenvironment weakened the capture function of the linkers, resulting in the redispersion of poly-L-lysine and the release of zoledronic acid for immune regulation. In the De Geest group's work,53 a pH-sensitive immunomodulator with a TLR 7/8 agonist and hydrophobic polymers was synthesized, which had high stability under physiological pH and low stability in acid conditions due to the hydrolyzation of the ketal bond (Fig. 2C). This change improved the pharmacokinetics of the immunomodulator and promoted the activity of the immune cells. Lee et al.54 reported a pH-responsive amphiphilic cationic polymer using acid-sensitive organic groups (piperazine and diethylenetriamine) to deliver a DNA vaccine and doxorubicin (DOX) to improve chemoimmunotherapy.
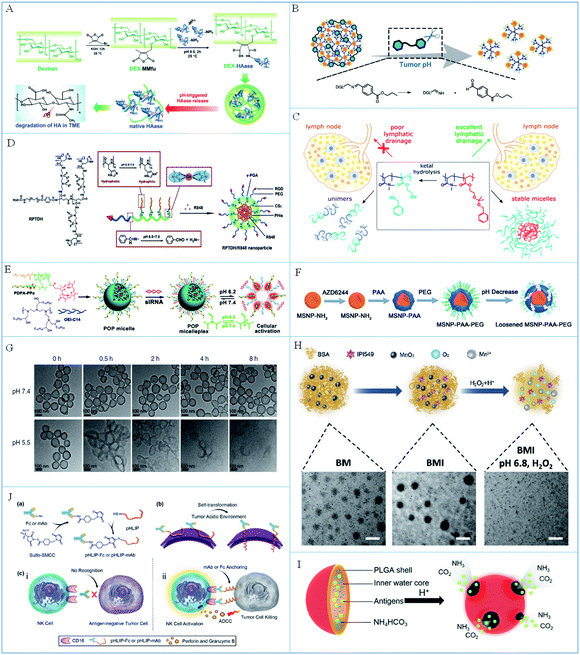 |
| Fig. 2 (A) A schematic illustration of the synthesis and pH-triggered disintegration of DEX-HAase NPs. Reproduced from ref. 49 with permission from WILEY-VCH Verlag GmbH & Co. KGaA, Weinheim, copyright 2019. (B) A schematic illustration of the degradation of SEN/ZOL NPs in the acidic tumor microenvironment. Reproduced from ref. 52 with permission from WILEY-VCH Verlag GmbH & Co. KGaA, Weinheim, copyright 2019. (C) The principle of the hydrolysis of micelles in lymph nodes. Reproduced from ref. 53 with permission from American Chemical Society, copyright 2018. (D) A schematic illustration of the synthesis of pH-responsive RPTDH/R848 NPs. Reproduced from ref. 57 with permission from Elsevier Ltd, copyright 2019. (E) A schematic illustration of micelle preparation and dissolution at low pH. Reproduced from ref. 58 with permission from the American Chemical Society, copyright 2016. (F) A schematic illustration of the synthesis and pH-induced degradation of MSNPs. Reproduced from ref. 60 with permission from WILEY-VCH Verlag GmbH & Co. KGaA, Weinheim, copyright 2019. (G) TEM images of hollow MnO2 at pH 7.4 and 5.5. Reproduced from ref. 61 with permission from Springer Nature, copyright 2019. (H) The process of BMI responsiveness and corresponding TEM images. Adapted from ref. 62 with permission from WILEY-VCH Verlag GmbH & Co. KGaA, Weinheim, copyright 2019. (I) A schematic illustration of the PLGA NP composition and response principle. Reproduced from ref. 64 with permission from the American Chemical Society, copyright 2015. (J) A schematic illustration of the preparation and working mechanism of pHLIP-Fc. Reproduced from ref. 65 with permission from WILEY-VCH Verlag GmbH & Co. KGaA, Weinheim, copyright 2018. | |
The transformation from the negative to the positive charge state can improve the uptake of nanoparticles by cancer cells and permeation into deep tumors. Based on this, Cai et al.55 synthesized an acid-responsive micelleplex loaded with PD-L1-siRNA and photosensitizer for photodynamic-improved PD-L1 immunotherapy against cancer. In this system, the charge and size of the micelleplex could be transformed due to cleavage of the amide bond in the acidic tumor microenvironment to increase permeation into cancer cells. Liu et al.56 reported a smart pH-responsive nanoreactor for delivery of catalase and chlorin e6 (Ce6) into deep tumors to enhance the photodynamic-immunotherapy of cancer. When reached the tumor microenvironment, the nanoreactor changed to positive charge due to the decomposition of 2,3-dimethylmaleic anhydride in acid, which improved the internalization in cancer cells for enhanced cancer therapy. Moreover, changes of hydrophilicity and hydrophobicity can also lead to the rupture of nanoparticles to control the drug release. For example, Wang et al.57 reported the pH-responsive nanoparticle with a copper chelating agent and TLR 7/8 agonist for anti-angiogenesis and cancer immunotherapy (Fig. 2D). In these nanoparticles, the RGD peptide and PEG chain were connected with poly-γ-glutamic acid (γ-PGA) via a low-pH-cleavable benzoic-imine bond. The copper chelating agent was linked with poly-L-histidine (PHis), which could change from hydrophobic to hydrophilic in acidic conditions to dissolve the nanoparticles and release the drugs.
To sum up, the functional substances (such as vaccines or adjuvants) of immunotherapy can be released in a controlled way by breaking the pH-labile chemical bonds in the acidic tumor microenvironment to improve the controllability of immunotherapy. Compared with the vaccines or adjuvants carried by nanocarriers, their free counterparts are easily cleared, resulting in a low utilization rate. Moreover, the vaccines or adjuvants could be released more rapidly from pH-responsive nano immunomodulators due to the superior size and permeability of H+, which will increase the chance of vaccines or adjuvants interacting with immune cells in immune synapses and improve the efficiency of immune regulation. Therefore, it is advantageous for a drug delivery system to achieve excellent drug delivery and utilization efficiency by changing the form of the nanoparticles through breaking low-pH-cleavable bonds, which is also useful for stimulating the immune response on demand. However, the response accuracy is hard to control due to the fact that the lysosome is more acidic than the tumor microenvironment in the body. It is a significant topic to study the release accuracy of functional substances and improve the controllability of the response in the future.
3.1.2 pH-Responsive nano immunomodulators with acid-protonated groups.
Organic nanoparticles (including micelles, lipidosomes, etc.) with acid-protonated groups can break up under acidic conditions to release functional substances for cancer immunotherapy. Li et al.58 developed a pH-responsive and multifunctional micelle consisting of a pH-sensitive diblock copolymer, photosensitizer and PD-L1 small interfering RNA (siRNA) (Fig. 2E). This micelle broke down due to protonation of the tertiary amines to release the photosensitizer and siRNA for photodynamic immunotherapy in the acidic tumor microenvironment. Wang et al.59 introduced the acid-protonated polyamidoamine into nanocarriers to specifically deliver drugs to tumor tissue for cancer chemoimmunotherapy. The Pt prodrug was firstly conjugated to the amphiphilic copolymer, which then self-assembled with the hydrophobic BLZ-945 to form nanoparticles. When they reached the tumor, the nanoparticles changed from hydrophobic to hydrophilic due to the rapid protonation of polyamidoamine in the amphiphilic copolymer to release drugs for collaborative cancer treatment. In addition, Shi's group60 developed a nanocarrier that could sufficiently release MEK inhibitors (MEKi) due to the protonation of polyacrylic acid in the acid environment to avoid the restrictions on the immunity of the cytotoxic T-lymphocyte and synergize with PD-1 blockade immunotherapy (Fig. 2F).
These protonated nanomaterials are basically organic nanoparticles formed by self-assembly of polymers containing nitrogen or oxygen functional groups. When the functional groups are protonated under acidic conditions, the changes of hydrophilicity and hydrophobicity or the electric charge of polymer chains, will directly lead to the rupture of organic nanoparticles, thus releasing the loaded vaccines or adjuvants, etc. Compared with non-responsive nanoparticles, the smaller size or the change of charge from negative to positive resulting from protonation can increase the uptake rate of nanoparticles by cells, thus increasing the utilization rate of the vaccines or adjuvants. These protonated strategies can release immunomodulators in a controlled manner to reduce the risk of an unexpected immune response, which plays a vital role in improving cancer immunotherapy. Despite this, some polymer chains with a high protonation efficiency (e.g. polyethyleneimine) are toxic, which would cause serious side effects during cancer immunotherapy.
3.1.3 pH-Responsive nano immunomodulators with acid-soluble inorganic material.
Inorganic nanomaterials (e.g., MnO2 and CaCO3) can react with H+ under acidic conditions to cause a collapse of structure to release the drugs. Liu et al.61 synthesized hollow MnO2 loaded with Ce6 and DOX for the purpose of combining chemotherapy, photodynamic therapy and immunotherapy. MnO2 can not only react with H+ from the tumor microenvironment to generate Mn2+ to release drugs, but also catalyze endogenous H2O2 to generate O2 to reverse the tumor microenvironment to promote photodynamic immunotherapy (Fig. 2G). Shuai and co-workers62 also constructed a nano-system utilizing MnO2 (Fig. 2H). They used BSA as a carrier to deliver MnO2 nanoparticles and the phosphoinositide 3-kinase (PI3Kγ) inhibitor IPI549. In the acidic tumor microenvironment, the MnO2 nanoparticles disintegrated to release IPI549, inhibiting the PI3Kγ molecules on the myeloid-derived suppressor cells (MDSCs) and further bringing down the expression of PD-L1 to enhance immunotherapy. Our group63 designed a CaCO3-based nano-immunomodulator to specifically deliver and release immune agents to the tumor for cancer immunotherapy. As the nano-immunomodulator entered the acidic tumor microenvironment, the CaCO3 dissolved to release CpG ONDs to activate the dendritic cells and indoleamine-2,3-dioxygenase inhibitor (IDOi) to inhibit the indoleamine-2,3-dioxygenase (IDO) enzyme and attenuate the immunosuppression microenvironment. Interestingly, the released Ca2+ played a vital role in improving the activity of T cells for suppressing the growth of breast cancer. Besides, NH4HCO3 can react with H+ to generate gas, which will promote the rapid release of drugs in the tumor microenvironment. Based on this, Ma et al.64 developed PLGA nanoparticles loading with NH4HCO3 to specifically and rapidly release antigens to promote the immune response in the tumor microenvironment (Fig. 2I). These acid-soluble inorganic nanomaterials can be dissolved in acidic conditions without additional chemical modifications, which reduces the experimental steps and is beneficial for promoting the application of nanocarriers.
Acid-soluble inorganic nanoparticles are mostly metal oxides, which can dissolve and release vaccines or adjuvants under acidic conditions without complex modifications. The released metal ions often possess catalytic and other biological properties for synergistic vaccines or adjuvants to promote the immune response. However, the acid dissolution efficiency of some inorganic nanoparticles is not high enough, which discourages the rapid interaction of vaccines or adjuvants with immune cells. Therefore, it is suggested that inorganic nanoparticles be modified with other pH-labile chemical bonds to synergistically respond to acid to improve the release efficiency. In addition, the metabolism of inorganic nanomaterials in vivo should be studied in the future to advance their clinical application.
3.1.4 Other pH-responsive nano immunomodulators.
The pH low insertion peptide (pHLIP) is a type of pH-responsive peptide that can insert into the cell membrane due to its conformation change from random coil to α-helix in acid conditions. Based on this, Nie et al.65 modified the antibodies or their Fc segments that could activate natural killer (NK) cells on the surface of cancer cells utilizing the pHLIP, which gave the cancer cells the ability to activate the immune cells adequately for enhanced NK cell immunotherapy (Fig. 2J).
Compared with other acid-responsive chemical bonds, such peptides are pure biological macromolecules with better biocompatibility and less influence on the normal physiological functions of cells, which is better suited to modify cells in cancer immunotherapy. However, when the pHLIP is injected intravenously, the free peptide might activate NK cells and be cleared by the immune system during the circulation process. Therefore, it is suggested that the pHLIP be preliminarily protected first. When it reaches the tumor microenvironment, the pHLIP will be exposed and undergo conformational changes to modify the cancer cells. This will be of more research value and will have wider applications.
3.2 ROS-responsive nano immunomodulators
3.2.1 Endogenous ROS-responsive nano immunomodulators.
ROS are crucial components of the immunosuppressive tumor microenvironment so developing ROS-modulating nanoparticles is of importance for cancer immunotherapy. Gu et al.66 constructed a ROS-sensitive hydrogel for the transportation of anti-PD-L1 and IDOi for an enhanced immune checkpoint blockade (Fig. 3A). In the presence of tumorous H2O2, the sulfoether groups of the hydrogel were oxidized to sulfoxide/sulfone, which resulted in the degradation of the hydrogel to release anti-PD-L1 and IDOi to inhibit the PD-1/PD-L1 pathway and the activity of IDO enzyme to prevent the tumor immune escape. Gu's group further67 reported a nano-complex with an ROS-responsive linker that released anti-CD47 and anti-PD-1 via consuming ROS from the tumor microenvironment to enhance immunotherapy (Fig. 3B). Moreover, ROS consumption can reverse the immunosuppressed tumor microenvironment and further improve the immune response. Yang et al.68 developed a ROS-responsive nanocarrier with a peroxalate ester bond that could react with H2O2 from the tumor microenvironment to deliver antigens to activate immune cells. Since then, their group69 applied HA instead of PEI to modify the above nanoparticles to specifically recognize CD44 on the surface of cancer cells and dendritic cells (Fig. 3C). The nanoparticles were disintegrated by H2O2 to release the antigens and activate the host immune response for cancer immunotherapy.
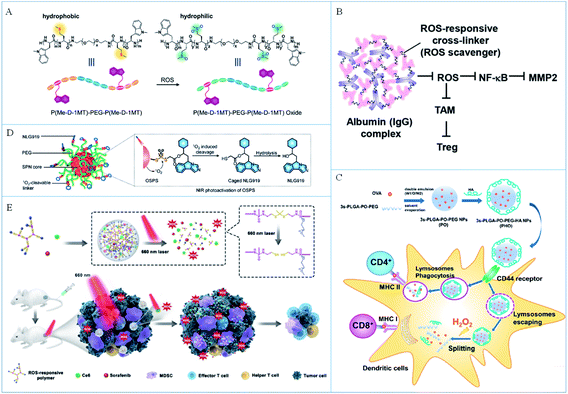 |
| Fig. 3 (A) A schematic illustration of ROS-sensitive molecules and the ROS-induced hydrophilicity/hydrophobicity transition. Reproduced from ref. 66 with permission from WILEY-VCH Verlag GmbH & Co. KGaA, Weinheim, copyright 2018. (B) The working mechanism of a ROS-responsive complex for immunotherapy. Reproduced from ref. 67 with permission from the American Chemical Society, copyright 2019. (C) A schematic illustration of the preparation of PHO NPs and the ROS-triggered release of antigens for immunotherapy. Reproduced from ref. 69 with permission from the American Chemical Society, copyright 2018. (D) The composition and response principle of organic semiconducting nanoparticles. Reproduced from ref. 70 with permission from Wiley-VCH Verlag GmbH & Co. KGaA, Weinheim, copyright 2019. (E) A schematic illustration of the ROS-induced release of drugs for enhanced photo-immunotherapy. Reproduced from ref. 71 with permission from Elsevier Ltd, copyright 2020. | |
On the one hand, the dissolution of nanomaterials induced by endogenous ROS caused controllable drug release, which improved the safety of cancer immunotherapy. On the other hand, the consumption of ROS in tumor tissues can reverse the immunosuppressive microenvironment of the tumor and improve the effect of immunotherapy. However, the endogenous ROS concentration is not high enough, so the response rate of the nano immunomodulators is limited, which may affect the release efficiency of the antigens or adjuvants. In addition, the inflammatory environment also expresses a high level of ROS, so the specificity of the response needs to be further studied. Therefore, endogenous ROS responsive strategies should be combined with other specific tumor-targeted functional groups to play a better role in cancer immunotherapy.
3.2.2 Exogenous ROS-responsive nano immunomodulators.
In addition to endogenous ROS, photosensitizers with the stimulation of external laser irradiation can also produce ROS to trigger the release of immunomodulators. Pu et al.70 designed singlet oxygen (1O2)-responsive organic semiconducting nanoparticles to selectively release the immunostimulant IDOi under laser irradiation to inhibit the IDO enzyme and down-regulate the regulatory T cells for enhanced cancer immunotherapy (Fig. 3D). Sun and co-workers71 designed a ROS-responsive nanocarrier for transporting the photosensitizer Ce6 and immunosuppressive cells inhibitor sorafenib for cancer immunotherapy (Fig. 3E). The ROS generated from Ce6 under laser irradiation could not only boost the immunogenic cell death (ICD) of cancer cells, but could also break the ROS-labile thioketal to cause the nanoparticles to collapse and quickly release sorafenib to inhibit myeloid-derived suppressor cells. Yang's group72 also designed ROS-responsive core–shell nanoparticles with thioketal to convey two types of ICD inducer (DOX and Ce6) to elevate the anti-tumor immune response of anti-PD-L1. Irradiation of 660 nm impelled Ce6 to produce a large amount of ROS, which cleaved the thioketal bond to break the nanoparticles to release DOX. This cascade chemo-photodynamic therapy also induced the exposure of CRT proteins as “eat me” signals to provoke the immune response. Furthermore, anti-PD-L1 fostered these immune responses by blocking the inhibition of cancer cells to activate T cells.
In these strategies, exogenous ROS are always produced by photosensitizers so the amount of ROS can be several times more than that of endogenous ROS, which makes the chemical bonds more efficiently responsive to ROS and leads to a more thorough drug release. In addition, the generated ROS can also induce the ICD of cancer cells to increase the tumor immunogen and further promote the occurrence of the immune response to improve the efficiency of cancer immunotherapy. However, the introduction of an additional photosensitizer increased the complexity and security risk in the application. Considering that the laser irradiation is required to activate the photosensitizers to produce ROS, the penetration depth of light is perhaps the greatest limitation during the in vivo application. Moreover, excessive ROS can also trigger a strong inflammatory response in the body, which is detrimental to cancer immunotherapy. Therefore, it will be a future research focus to develop strategies to control the levels of produced ROS not only to break chemical bonds and induce the appropriate ICD, but also to avoid a severe inflammatory response.
3.3 Enzyme-responsive nano immunomodulators
3.3.1 MMP-responsive nano immunomodulators.
Plenty of MMPs exist in inflammatory environments, including tumor microenvironments after surgery. Therefore, MMP-responsive nano immunomodulators were extensively researched. Gu's group73 developed a DNA “nano-cocoon” (DNC) for delivery of CpG and anti-PD1 to enhance cancer immunotherapy (Fig. 4A). The CpG sequences and HhaI enzyme were packaged in triglycerol monostearate nanoparticles (TGMS NPs). In the tumor microenvironment, TGMS NPs could be dissociated with the assistance of MMPs to liberate HhaI and further break down the DNA network to release the anti-PD1 and CpG adjuvant to inhibit cancer recurrence and metastasis. The MMP-responsive TGMS NPs were also used in Liu's design74 to load DOX and palladium nanoparticles to induce ICD of cancer cells to enhance the immune checkpoint blockade therapy. Gong et al.75 reported liposome-based nanoparticles (PEG–FA–Lip) with the MMP-sensitive PEG2000-peptide to expose FA to improve the uptake of nanoparticles by cancer cells and M2-type macrophages in the tumor microenvironment (Fig. 4B). After the peptide fractured, the encapsulated chemotherapeutic drug DOX could eliminate M2 macrophages to relieve the immunosuppressed tumor microenvironment and induce ICD of cancer cells to release tumor-related antigens. Photothermal therapy (PTT) can also induce apoptosis/necrosis of cancer cells to release tumor-associated antigens to stimulate the maturation of dendritic cells. The inhibition of the IDO pathway can achieve a good synergistic effect with PTT in cancer treatment by preventing the immune escape of tumor. On this basis, Ma et al.76 synthesized MMP-responsive nanoparticles to convey PTT reagents and IDOi to enhance immunotherapy (Fig. 4C). In these nanoparticles, the MMP-2-cleavable peptide PVGLIG was used to connect the PEG chain and IDOi, which could be fractured in the tumor microenvironment with MMP-2. Then the nanoparticle fractured to release drugs for synergistic treatment.
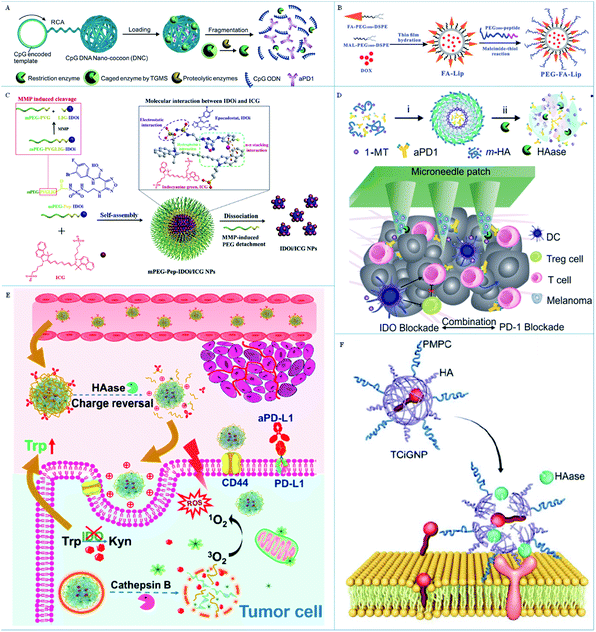 |
| Fig. 4 (A) A schematic illustration of the delivery and enzyme-responsive release system. Reproduced from ref. 73 with permission from WILEY-VCH Verlag GmbH & Co. KGaA, Weinheim, copyright 2016. (B) A schematic illustration of the preparation of liposome-based NPs. Reproduced from ref. 75 with permission from WILEY-VCH Verlag GmbH & Co. KGaA, Weinheim, copyright 2019. (C) A schematic illustration of the preparation and response principle of MMP-responsive nanoparticles. Reproduced from ref. 76 with permission from Elsevier Ltd, copyright 2020. (D) A schematic illustration of the TME-responsive nanoplatform for anticancer immunotherapy. Reproduced from ref. 77 with permission from the American Chemical Society, copyright 2016. (E) A schematic illustration of the enzyme-responsive delivery system for the step-by-step release of antitumor drugs. Adapted from ref. 78 with permission from the American Chemical Society, copyright 2019. (F) A schematic illustration of the TME-response principle of TCiGNPs. Adapted from ref. 79 with permission from Ivyspring International Publisher Pty Ltd, copyright 2019. | |
These MMP-sensitive peptides are pure biological molecules with low toxicity and can respond specifically to MMP, which is insusceptible to other enzymes or active substances. In theory, it is beneficial to design the responsive nano immunomodulators by using MMP-sensitive peptides to regulate the anti-tumor immune response as required. However, MMPs, a kind of macromolecule, are difficult to get into the nanoparticles and cut off the peptides, which will decrease the release efficiency of the vaccines or adjuvants. Therefore, it is suggested that the peptides be modified to the surface of nanoparticles when designing nano immunomodulators to improve the contact probability of the peptide and MMPs.
3.3.2 HAase-responsive nano immunomodulators.
HA, a biocompatible polysaccharide, can specifically bind to CD44 on the cancer surface and be broken down by the overexpressed HAase in the tumor microenvironment. In recent years, HA has been widely applied in the field of cancer therapy as a drug delivery carrier for targeted drug delivery. Gu et al.77 designed a specific drug delivery nanocapsule by the self-assembly of HA-modified IDOi and anti-PD1 (Fig. 4D). After accumulating in tumor tissues, HA was decomposed by HAase to release drugs and enhance cancer immunotherapy. Luan et al.78 developed a HAase-responsive nanoweapon that consisted of HA-Ce6 complexes and anti-PD-L1 (Fig. 4E). With the overexpressed HAase in the tumor microenvironment, the charge of the nanoparticles changed from negative to positive, accelerating the cancer cells' uptake of the drug and further promoting the release of the anti-PD-L1 to perform the immune checkpoint blockade therapy. Since the apoptosis mediated by perforin and granzyme B (GrB) is the critical mechanism for T cells to fight cancer cells, Kang et al.79 established a HAase-responsive nanoparticle with modified HA to convey GrB to simulate immune cell function in the tumor microenvironment for cancer immunotherapy (Fig. 4F).
These nano immunomodulators based on HA have several merits for applications in cancer immunotherapy compared with conventional nanomedicine. HA molecules can be easily modified to nanomaterials by the active functional groups to decrease the side effects. In addition, HA has a specific targeting ability to tumor tissue, which can improve the tumor enrichment of the nanomaterials. Significantly, HA can be specifically broken down by HAase to release drug molecules controllably to induce the required immune response. Therefore, it is suggested that the nano immunomodulators should be fabricated with the vaccines or adjuvant inside and HA on the surface of the nanocarrier. These formed nano immunomodulators can not only protect the functional molecules from degradation, but can also improve the biocompatibility and the blood circulation time in vivo. Moreover, such nano immunomodulators can increase the chance of contact between the HAase and the HA so as to improve the response rate and probability of the enzyme to rapidly activate the body's immune response.
3.4 GSH-responsive nano immunomodulators
The level of GSH in tumors is significantly higher than that in normal tissue due to the necessary redox equilibrium in the tumor microenvironment. Therefore, it is feasible to design GSH-responsive drug delivery systems to enhance the immunomodulatory therapeutic effect. Disulfide bonds are usually introduced to construct these GSH-responsive systems. For example, Wang et al.80 developed a GSH-sensitive liposome with a disulfide bond for PDT-induced immunotherapy (Fig. 5A). In this system, the disulfide linkage broke due to the high GSH level in the tumor microenvironment to induce the dissociation of liposomes and release IDOi for cancer immunotherapy. Lin and partners81 designed GSH-responsive core–shell nanoparticles to deliver ICD inducers (dihydroartemisinin and oxaliplatin) to a tumor (Fig. 5B). Dihydroartemisinin was bound to cholesterol by a disulfide bond in the shell of the nanoparticles and could be released with high GSH levels in the tumor microenvironment to produce ROS and induce oxidative stress. Combining with oxaliplatin, the ICD of cancer cells was induced in situ to activate immune cells. Recently, Chen et al.82 designed reduction-sensitive nanoparticles containing a disulfide bond and ER-targeting photosensitizers for cancer immunotherapy (Fig. 5C). In this work, the nanoparticles dissociated with high GSH levels in the tumor microenvironment to release ER-targeted photosensitizers to induce ER stress and amplify ICD of cancer cells. In addition, Xue's group83 utilized a GSH-responsive MOF for delivery of antigens and adjuvant to the same immune cell. These GSH-sensitive nanoparticles can prevent the early release of antigens and enhanced the OVA-specific immunity. Li et al.84 designed a GSH-responsive amphipathic PEG–NLG copolymer to deliver IDOi and DOX to improve chemo-immunotherapy (Fig. 5D). IDOi was bound to the polymer backbone by a disulfide bond, which could be broken with high GSH levels in the tumor microenvironment to release IDOi and DOX and enhance the synergistic treatment.
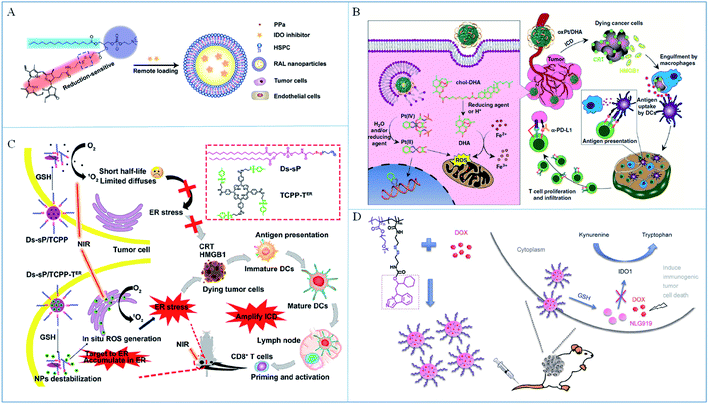 |
| Fig. 5 (A) A schematic illustration of self-assembly for GSH-sensitive liposomes. Adapted from ref. 80 with permission from the American Chemical Society, copyright 2019. (B) A schematic illustration of the response and immune mechanisms of the core–shell nanoparticles. Reproduced from ref. 81 with permission from Springer Nature, copyright 2019. (C) The structure of the NPs and their application to amplify ICD. Reproduced from ref. 82 with permission from the American Chemical Society, copyright 2020. (D) The preparation and anticancer mechanism of GSH-responsive nano immunomodulators. Reproduced from ref. 84 with permission from Springer Nature, copyright 2017. | |
Although more GSH exists in tumor tissue than in normal tissue, drug molecules are inevitably released in normal tissue due to GSH also being expressed there. In addition, there is also a high level of GSH in the blood so it is risky that drug molecules will be released during blood circulation and cause serious side effects. Therefore, a single GSH-responsive nano immunomodulator could not significantly reduce the non-specific drug release during treatment. It is suggested for multi-responsive nano immunomodulators to be designed to further synergistically control drug release.
3.5 Multi-responsive nano immunomodulators
Considering the complexity of the tumor microenvironment, the development of multi-responsive nano immunomodulators can improve the degree of controlled drug release to reduce unwanted side-effects. We summarized several types of hybrid responsive designs for immune regulation.
3.5.1 pH + enzyme responsive nano immunomodulators.
Low pH and overexpressed enzyme are two prominent features of the tumor microenvironment. Nano immunomodulators with both pH- and enzyme-responsive properties would improve the efficiency of immune modulation more powerfully in tumor issue. For instance, Nie et al.85 synthesized a pH + MMP-2 responsive nano-prodrug consisting of IDOi, amphiphilic peptides with 3-diethylaminopropyl isothiocyanate (DEAP), a peptide substrate of MMP-2, and a short D-peptide antagonist of PD-L1 (DPPA-1). The weak acidity can induce the protonation of DEAP and lead to the expanding of the nanoparticles. In addition, the highly expressed MMP-2 in the tumor cleaved the peptide substrate, which further caused the nanoparticles to collapse and release the immunosuppressant, enhancing immunotherapy.
3.5.2 pH + GSH responsive nano immunomodulators.
To avoid the early drug release of single GSH-responsive nano immunomodulators, pH + GSH responsive strategies have been fabricated to cooperatively control drug release to trigger the required immune response. Zhang et al.86 designed a pH and GSH responsive mesoporous silica nanocarrier to break the barrier between chemotherapy and immunotherapy. The Bz-COOH protonated due to the low pH, and Fc+ reduced to Fc due to the high GSH level, which resulted in the controlled release of the drug to enhance antitumor immunotherapy. Moreover, the pH + GSH sensitive nanovectors can also be used for the co-delivery of IDOi and chemotherapeutic drugs. Yang et al.87 synthesized pH + GSH responsive nanoparticles with a transformable size and surface charge to simultaneously convey IDOi and curcumin for chemotherapy-improved immunotherapy. The disulfide and amide bonds of the nanoparticles broke in the acidic and GSH-rich tumor microenvironment, resulting in collapse of the micelle and release of the chemotherapeutic drug (curcumin) and immunoregulator (IDOi) for enhanced synergistic cancer treatment. Li et al.88 designed a core–shell nanoparticle that could respond to the tumorous acidic and reductive environment to deliver IDOi and oxaliplatin (OXA) drugs at the same time. In these nanoparticles, two molecules of IDOi were connected by disulfide bonds and self-assembled into the hydrophobic core. Then the acid-sensitive shell was formed by an amphiphilic block polymer containing the OXA prodrugs. Responding to the low pH and excess GSH in the tumor microenvironment, OXA was released to induce the ICD of cancer cells, and IDOi to down-regulate regulatory T cells to synergistically modulate the immune tumor microenvironment.
3.5.3 GSH + ROS responsive nano immunomodulators.
In addition, Li et al.89 also designed GSH- and ROS-responsive nanoparticles to deliver ICD inducer (oxaliplatin drugs and photosensitizer pheophorbide A) and immune modulators IDOi. The ROS-responsive thioketal bonds in the outer amphiphilic block polymer were cleavable by ROS generated under the irradiation to expose the kernel. Then the disulfide bond in the kernel was broken down by GSH to release IDOi for cancer immunotherapy.
The consumption of GSH or ROS will upset the REDOX balance to affect the growth of cancer cells. However, it may be a bad strategy to consume both GSH and ROS, which may be conducive to restore the REDOX balance and thus be detrimental to treatment. Therefore, GSH + ROS responsive nano immunomodulators are not popular in cancer immunotherapy.
3.5.4 pH + ROS responsive nano immunomodulators.
Nano immunomodulators that simultaneously respond to pH and ROS have also been developed. Blocking PD-L1 expressed on cancer cells is an effective strategy to enhance immunotherapy. However, PD-L1 is also expressed on normal cells. In view of this, it is significant to develop a vector for specific accumulation of the PD-L1-targeted CRISPR/Cas13a system in tumors. Thus, Liu and partners90 designed nanoparticles that require simultaneous conditions of H2O2 and low pH to completely release plasmid DNA. The low pH and excess H2O2 of the tumor microenvironment led to the decomposition of cis-aconitic anhydride and separation of 4-(hydroxymethyl)phenylboronic acid (HPBA) from PEI releasing plasmid DNA to disrupt the PD-1/PD-L1 pathway. Compared to the simplex pH-responsive and H2O2-responsive system, this dual-responsive system obviously increased the enrichment of plasmid DNA in cancer cells to improve the efficient cancer immunotherapy.
In the Gu group's design,91 the immunomodulators anti-PD1 and zebularine (Zeb) were released in tumor issue with the help of the acidic and ROS-rich tumor microenvironment. Firstly, anti-PD1 was encapsulated in CaCO3 nanoparticles (aPD1-NPs) utilizing the interaction between poly(ethylene glycol)–poly(glutamic acid) (PEG–P(Glu)) block copolymers with Ca2+ and CO32−. Then a hydrogel loaded with aPD1-NPs and Zeb was synthesized through crosslinking polyvinyl alcohol (PVA) with ROS-sensitive N1-(4-boronobenzyl)-N3-(4-boronophenyl)-N1,N1,N3,N3-tetramethylpropane-1,3-diaminium (TSPBA). The final material Zeb–aPD1-NPs-Gel was synthesized from Zeb and aPD1-NPs, which could be fully degraded in the presence of ROS and low pH to release anti-PD1, interdicting the PD1/PD-L1 pathway to increase the T cell-mediated antitumor effect. Chen et al.92 designed a nontoxic pH + ROS responsive polymer prodrug by the conjugation of phenylboronic acid (involving cinnamaldehyde (CA) derivatives) with PLG-g-mPEG to induce ICD of cancer cells by enhancing oxidative stress to boost antitumor immunity. When they reached the tumor microenvironment, the low pH triggered CA derivatives to produce CA and subsequently with the generation of quinone methide by H2O2 to consume GSH to increase the oxidative stress and ICD of cancer cells.
3.5.5 GSH + enzyme responsive nano immunomodulators.
For GSH- and enzyme-responsive nano immunomodulators, He and colleagues93 synthesized a GSH + caspase-3 responsive system for the controlled release of a chemotherapeutic drug (DOX) and IDOi for chemo-immunotherapy. In the tumor tissue, excessive GSH cleaved the disulfide bond to release DOX and kill cancer cells. In addition, active caspase-3 disintegrated the KDEVD peptide segment to release IDOi to inhibit the IDO enzyme and reverse the immunosuppressed state. Yu et al.94 designed a HAase and GSH responsive nanoplatform based on the host–guest interaction, inducing ICD and inhibiting the IDO-1 pathway to stimulate the antitumor immune response. This nanoplatform located in the tumor by binding with CD44 receptor and then disassembled under the breakage of HA and disulfide bond in the HAase- and GSH-rich tumor microenvironment. The released photosensitizer and IDOi functioned synergistically against cancer. Subsequently, they further95 reported a GSH and MMP-2 responsive vesicle, which consisted of photosensitizers with MMP-2-activatable peptide and IDOi prodrug with a GSH-sensitive bond. The high level of MMP-2 in the tumor resulted in stroma mediated peptide lysis and made the vesicle penetrate deeply into the tumor. The high GSH further induced the release of IDOi to inhibit the IDO pathway, promoting the activity of the effector T cells.
3.5.6 ROS + enzyme responsive nano immunomodulators.
For the ROS- and enzyme-responsive nano immunomodulators, Gao et al.96 designed HAase and ROS double-responsive core–shell nanoparticles to simultaneously initiate ICD and an immune checkpoint block against breast cancer. HA was hydrolyzed by HAase to expose the core of the nanoparticles. Then ROS produced by the photosensitizer under illumination can not only induce the ICD of cancer cells, but can also destroy the thioketal linker in the core to release cinnamaldehyde, paclitaxel and anti-PD-L1 peptide for synergetic treatment of breast cancer.
3.5.7 pH + GSH + enzyme responsive nano immunomodulators.
In addition to the above dual-responsive nano immunomodulators, triple-stimuli responsive nanoparticles for cancer immunotherapy were constructed by Yu's group.97 In these nanoparticles, three biologically stimuli-responsive linkers including the MMP-responsive peptide, GSH-sensitive disulfide bond and pH-activated 2-(diisopropylamino)ethyl methacrylate were introduced to control the release of the photosensitizer and IDOi, inducing ICD and regulating the immunosuppressive tumor microenvironment. This multi-response strategy achieved the specific and efficient delivery of immune modulators to tumor tissues.
Compared with single-responsive nano immunomodulators, these multi-responsive immunomodulators can indeed improve the accuracy of drug release and further improve biosecurity. However, the complex steps could increase the difficulty of synthesis and varieties of intermediate substances, which could increase the toxicity, lead to immune clearance, reduce the utilization rate of vaccines or adjuvants, and further increase the risk of the potential application. Moreover, the response and release efficiency of multi-responsive nano immunomodulators are also worth improving. For instance, in the triple-responsive systems, the low response rate of any factor will affect the overall release efficiency of the vaccines or adjuvants, which will decrease the chance of the vaccines or adjuvants interacting with immune cells and influencing the role of immune regulation. Therefore, it is not necessary to blindly pursue multifunctional materials but to construct reasonable responsive nano immunomodulators as required to promote the development of cancer immunotherapy.
4. Conclusions
There has been substantial progress in intelligent stimuli-responsive nano immunomodulators that utilize a unique tumor microenvironment or external stimuli to improve controlled drug-release strategies and reduce the side effects of materials on the body. However, it is still challenging to apply the above intelligent stimuli-responsive nano immunomodulators and realize their therapeutic potential clinically.
Multiple factors should be taken into account when designing stimuli-responsive nano immunomodulators and promoting their corresponding clinical application. Firstly, the properties of the nanomaterials are crucial to their impact on the immune system. Therefore, we suggest that biocompatible or biomimetic nanomaterials, especially FDA-approved nanocarriers, should be developed, which could facilitate the clinical application of such nanomedicine more easily. Secondly, responsive bonds or groups should be introduced to stimuli-responsive nano immunomodulators, which would increase the synthetic complexity and safety risks. Therefore, simplifying the synthesis steps and evaluating biosafety are also critical considerations. Thirdly, the large-scale production of synthesized nano immunomodulators is another key factor that directly affects clinical transformation. Hence, the repeatability and amplified production of stimuli-responsive nano immunomodulators should be well studied before their biological application. Fourthly, the selection of animal models is another crucial factor to advance the clinical application of stimuli-responsive nano immunomodulators. There are great differences between rodents and humans, so the results from rodents cannot be used to infer the results of human experiments directly. In addition, nude mice are usually immunodeficient and their tumor progression is much different from that of human beings, so the obtained experimental results are not convincing for the promotion of clinical biological applications. Therefore, it is suggested that animal models that are really similar to human tumor models should be established, which can advance the clinical development of stimuli-responsive nano immunomodulators and human tumor immunological studies. Fifthly, personalized treatment is one of the most important considerations during cancer immunotherapy. Immunotherapy does not work for everyone, so it is necessary to stratify and differentiate patients to preselect the right patients and ensure good therapeutic performance. It is expected that the application of appropriate stimuli-responsive nano immunomodulators in suitable patients for personalized treatment will greatly promote the application of stimuli-responsive nano immunomodulators in clinical practice.
In summary, the design of stimuli-responsive nano immunomodulators with high biosafety according to the specific needs of patients is needed to advance their clinical application. Moreover, in-depth research into the mechanism of the tumor microenvironment to find more gene expression support will be more conducive to the development of the design of intelligent stimuli-responsive nano immunomodulators in the future.
Author contributions
The manuscript was written through contributions from all authors. All authors have given approval to the final version of the manuscript.
Conflicts of interest
The authors declare no competing financial interests.
Acknowledgements
This work was supported by the Key Research and Development Program of Shandong Province (2018YFJH0502), National Natural Science Foundation of China (21927811, 21874086, and 21775094), National Key R&D Program of China (2019YFA0210100), and Youth Innovation Science and Technology Program of Higher Education Institution of Shandong Province (2019KJC022).
References
- D. Hanahan and R. A. Weinberg, Cell, 2011, 144, 646–674 CrossRef CAS.
- R. L. Siegel, K. D. Miller and A. Jemal, Ca-Cancer J. Clin., 2019, 69, 7–34 CrossRef.
- L. Galluzzi, O. Kepp, M. G. Vander Heiden and G. Kroemer, Nat. Rev. Drug Discovery, 2013, 12, 829–846 CrossRef CAS.
- Y. J. Liu, P. Bhattarai, Z. F. Dai and X. Y. Chen, Chem. Soc. Rev., 2019, 48, 2053–2108 RSC.
- W. P. Fan, P. Huang and X. Y. Chen, Chem. Soc. Rev., 2016, 45, 6488–6519 RSC.
- Z. Y. Shen, J. B. Song, B. C. Yung, Z. J. Zhou, A. G. Wu and X. Y. Chen, Adv. Mater., 2018, 30, 1704007 CrossRef.
- D. N. Khalil, E. L. Smith, R. J. Brentjens and J. D. Wolchok, Nat. Rev. Clin. Oncol., 2016, 13, 273–290 CrossRef CAS.
- C. W. Shields, L. L. W. Wang, M. A. Evans and S. Mitragotri, Adv. Mater., 2019, 32, 1901633 CrossRef.
- I. Mellman, G. Coukos and G. Dranoff, Nature, 2011, 480, 480–489 CrossRef CAS.
- A. Ribas and J. D. Wolchok, Science, 2018, 359, 1350–1355 CrossRef CAS.
- U. Sahin and Ö. Türeci, Science, 2018, 359, 1355–1360 CrossRef CAS.
- C. H. June, R. S. O'Connor, O. U. Kawalekar, S. Ghassemi and M. C. Milone, Science, 2018, 359, 1361–1365 CrossRef CAS.
- M. F. Sanmamed and L. P. Chen, Cell, 2018, 175, 313–326 CrossRef CAS.
- P. Hokland, M. Hokland and F. Cotter, Br. J. Haematol., 2018, 183, 698–700 CrossRef.
- J. Kaiser and J. Couzin-Frankel, Science, 2018, 362, 13 CrossRef CAS.
- X. P. Duan, C. Chan and W. B. Lin, Angew. Chem., Int. Ed., 2019, 58, 670–680 CrossRef CAS.
- J. Zhang, S. Endres and S. Kobold, Immunotherapy, 2019, 11, 201–213 CrossRef CAS.
- Y. Mi, C. T. Hagan, B. G. Vincent and A. Z. Wang, Adv. Sci., 2019, 6, 1801847 CrossRef.
- J. W. Kleinovink, M. F. Fransen, C. W. Löwik and F. Ossendorp, Cancer Immunol. Res., 2017, 5, 832–838 CrossRef CAS.
- S. M. Kaech and W. G. Cui, Nat. Rev. Immunol., 2012, 12, 749–761 CrossRef CAS.
- J. Xu, L. Xu, C. Wang, R. Yang, Q. Zhuang, X. Han, Z. Dong, W. Zhu, R. Peng and Z. Liu, ACS Nano, 2017, 11, 4463–4474 CrossRef CAS.
- L. K. Ferrarelli, Sci. Signaling, 2018, 11, 8122 CrossRef.
- D. J. Byun, J. D. Wolchok, L. M. Rosenberg and M. Girotra, Nat. Rev. Endocrinol., 2017, 13, 195–207 CrossRef CAS.
- J. Weiden, J. Tel and C. G. Figdor, Nat. Rev. Immunol., 2018, 18, 212–219 CrossRef CAS.
- T. T. Hansel, H. Kropshofer, T. Singer, J. A. Mitchell and A. J. T. George, Nat. Rev. Drug Discovery, 2010, 9, 325–338 CrossRef CAS.
- M. A. Postow, R. Sidlow and M. D. Hellmann, N. Engl. J. Med., 2018, 378, 158–168 CrossRef CAS.
- T. L. Whiteside, Oncogene, 2008, 27, 5904–5912 CrossRef CAS.
- J. C. Becker, M. H. Andersen, D. Schrama and P. T. Straten, Cancer Immunol. Immunother., 2013, 62, 1137–1148 CrossRef CAS.
- T. F. Gajewski, Y. R. Meng, C. Blank, I. Brown, A. Kacha, J. Kline and H. Harlin, Immunol. Rev., 2006, 213, 131–145 CrossRef CAS.
- S. Y. Han, K. Q. Huang, Z. P. Gu and J. Wu, Nanoscale, 2020, 12, 413–436 RSC.
- A. Gulzar, J. T. Xu, C. Wang, F. He, D. Yang, S. L. Gai, P. P. Yang, J. Lin, D. Y. Jin and B. G. Xing, Nano Today, 2019, 26, 16–56 CrossRef CAS.
- C. Roma-Rodrigues, R. Mendes, P. V. Baptista and A. R. Fernandes, Int. J. Mol. Sci., 2019, 20, 840 CrossRef CAS.
- P. Vaupel, Semin. Radiat. Oncol., 2004, 14, 198–206 CrossRef.
- J. Kim and C. V. Dang, Cancer Res., 2006, 66, 8927–8930 CrossRef CAS.
- W. Gao, J. M. Chan and O. C. Farokhzad, Mol. Pharm., 2010, 7, 1913–1920 CrossRef CAS.
- I. Bhme and A. K. Bosserhoff, Pigm. Cell Melanoma Res., 2016, 29, 508–523 CrossRef.
- F. Weinberg, N. Ramnath and D. Nagrath, Cancers, 2019, 11, 1191 CrossRef CAS.
- P. P. Jia, C. Y. Dai, P. H. Cao, D. Sun, R. Z. Ouyang and Y. Q. Miao, RSC Adv., 2020, 10, 7740–7750 RSC.
- P. Rai, J. J. Young, D. G. A. Burton, M. G. Giribaldi, T. T. Onder and R. A. Weinberg, Oncogene, 2011, 30, 1489–1496 CrossRef CAS.
- V. Sosa, T. Moliné, R. Somoza, R. Paciucci, H. Kondoh and M. E. LLeonart, Ageing Res. Rev., 2013, 12, 376–390 CrossRef CAS.
- E. Hadler-Olsen, J. O. Winberg and L. Uhlin-Hansen, Tumor Biol., 2013, 34, 2041–2051 CrossRef CAS.
- M. Egeblad and Z. Werb, Nat. Rev. Cancer, 2002, 2, 161–174 CrossRef CAS.
- Q. Yao, L. F. Kou, Y. Tu and L. Zhu, Trends Pharmacol. Sci., 2018, 39, 766–781 CrossRef CAS.
- J.-X. Tan, X.-Y. Wang, H.-Y. Li, X.-L. Su, L. Wang, L. Ran, K. Zheng and G.-S. Ren, Int. J. Cancer, 2011, 128, 1303–1315 CrossRef CAS.
- B. Delpech, A. Laquerriere, C. Maingonnat, P. Bertrand and P. Freger, Anticancer Res., 2002, 22, 2423–2427 Search PubMed.
- R. A. Cairns, I. S. Harris and T. W. Mak, Nat. Rev. Cancer, 2011, 11, 85–95 CrossRef CAS.
- L. Wang, M. Huo, Y. Chen and J. Shi, Adv. Healthcare Mater., 2017, 7, 1701156 CrossRef.
- S. Uthaman, K. M. Huh and I. K. Park, Biomater. Res., 2018, 22, 22 CrossRef.
- H. R. Wang, X. Han, Z. L. Dong, J. Xu, J. Wang and Z. Liu, Adv. Funct. Mater., 2019, 29, 1902440 CrossRef.
- H. C. Chang, Z. Z. Zou, Q. H. Wang, J. Li, H. Jin, Q. X. Yin and D. Xing, Adv. Sci., 2020, 7, 1900069 CrossRef CAS.
- Y. M. Xing, Z. K. Xu, T. Liu, L. Q. Shi, D. Kohane and S. T. Guo, Angew. Chem., Int. Ed., 2020, 59, 1–6 CrossRef.
- Q. Guo, X. He, C. Li, Y. Q. He, Y. Y. Peng, Y. Zhang, Y. F. Lu, X. L. Chen, Y. J. Zhang, Q. J. Chen, T. Sun and C. Jiang, Adv. Sci., 2019, 6, 1901430 CrossRef CAS.
- S. Van Herck, K. Deswarte, L. Nuhn, Z. Zhong, J. P. P. Catani, Y. Li, N. N. Sanders, S. Lienenklaus, S. De Koker, B. N. Lambrecht, S. A. David and B. G. De Geest, J. Am. Chem. Soc., 2018, 140, 14300–14307 CrossRef CAS.
- H. T. T. Duong, T. Thambi, Y. Yin, J. E. Lee, Y. K. Seo, J. H. Jeong and D. S. Lee, ACS Appl. Mater. Interfaces, 2019, 11, 13058–13068 CrossRef CAS.
- L. L. Dai, K. Li, M. H. Li, X. J. Zhao, Z. Luo, L. Lu, Y. F. Luo and K. Y. Cai, Adv. Funct. Mater., 2018, 28, 1707249 CrossRef.
- G. Yang, L. Xu, J. Xu, R. Zhang, G. Song, Y. Chao, L. Feng, F. Han, Z. Dong, B. Li and Z. Liu, Nano Lett., 2018, 18, 2475–2484 CrossRef CAS.
- P. Zhou, J. Q. Qin, C. Zhou, G. Y. Wan, Y. Y. Liu, M. M. Zhang, X. Y. Yang, N. Zhang and Y. S. Wang, Biomaterials, 2019, 195, 86–99 CrossRef CAS.
- D. Wang, T. Wang, J. Liu, H. Yu, S. Jiao, B. Feng, F. Zhou, Y. Fu, Q. Yin, P. Zhang, Z. Zhang, Z. Zhou and Y. Li, Nano Lett., 2016, 16, 5503–5513 CrossRef CAS.
- S. Shen, H.-J. Li, K.-G. Chen, Y.-C. Wang, X.-Z. Yang, Z.-X. Lian, J.-Z. Du and J. Wang, Nano Lett., 2017, 17, 3822–3829 CrossRef CAS.
- X. W. Liu, Y. L. Feng, G. C. Xu, Y. Chen, Y. Luo, J. N. Song, Y. Bao, J. Q. Yang, C. E. Yu, Y. N. Li, H. Y. Ye, B. W. Ke, B. Chen, J. P. Hu, J. Xu, H. Meng, H. Y. Zhang and H. B. Shi, Adv. Funct. Mater., 2019, 29, 1806916 CrossRef.
- G. Yang, L. Xu, Y. Chao, J. Xu, X. Sun, Y. Wu. R. Peng and Z. Liu, Nat. Commun., 2017, 8, 902 CrossRef.
- M. Yu, X. H. Duan, Y. J. Cai, F. Zhang, S. Q. Jiang, S. S. Han, J. Shen and X. T. Shuai, Adv. Sci., 2019, 6, 1900037 CrossRef.
- Y. Li, S. Gong, W. Pan, Y. Chen, B. Liu, N. Li and B. Tang, Chem. Sci., 2020, 11, 7429–7437 RSC.
- Q. Liu, X. Chen, J. Jia, W. Zhang, T. Yang, L. Wang and G. Ma, ACS Nano, 2015, 9, 4925–4938 CrossRef CAS.
- T. J. Ji, J. Y. Lang, B. Ning, F. F. Qi, H. Wang, Y. L. Zhang, R. F. Zhao, X. Yang, L. J. Zhang, W. Li, X. H. Shi, Z. H. Qin, Y. Zhao and G. J. Nie, Adv. Mater., 2019, 31, 1804395 Search PubMed.
- S. J. Yu, C. Wang, J. C. Yu, J. Q. Wang, Y. Lu, Y. Q. Zhang, X. D. Zhang, Q. Y. Hu, W. J. Sun, C. L. He, X. S. Chen and Z. Gu, Adv. Mater., 2018, 30, 1801527 CrossRef.
- Q. Chen, G. Chen, J. Chen, J. Shen, X. Zhang, J. Wang, A. Chan and Z. Gu, Nano Lett., 2019, 19, 4879–4889 CrossRef CAS.
- X. Liang, J. W. Duan, X. Li, X. Zhu, Y. Chen, X. Wang, H. Sun, D. Kong, C. Li and J. Yang, Nanoscale, 2018, 10, 9489–9503 RSC.
- X. Liang, X. Li, J. Duan, Y. Chen, X. Wang, L. Pang, D. Kong, B. Song, C. Li and J. Yang, Mol. Pharm., 2018, 15, 508–518 CrossRef CAS.
- J. C. Li, D. Cui, J. G. Huang, S. He, Z. B. Yang, Y. Zhang, Y. Luo and K. Y. Pu, Angew. Chem., Int. Ed., 2019, 58, 12680–12687 CrossRef CAS.
- X. Sun, Z. Y. Cao, K. R. Mao, C. X. Wu, H. M. Chen, J. L. Wang, X. Wang, X. X. Cong, Y. Li, X. Y. Meng, X. Z. Yang, Y. G. Yang and T. M. Sun, Biomaterials, 2019, 240, 119845 CrossRef.
- L. Q. Hua, Z. Y. Cao, L. L. Ma, Z. Q. Liu, G. C. Liao, J. X. Wang, S. Shen, D. D. Li and X. Z. Yang, Biomaterials, 2019, 223, 119469 CrossRef.
- C. Wang, W. J. Sun, G. Wright, A. Z. Wang and Z. Gu, Adv. Mater., 2016, 28, 8912–8920 CrossRef CAS.
- Y. Wen, X. Chen, X. Zhu, Y. Gong, G. Yuan, X. Qin and J. Liu, ACS Appl. Mater. Interfaces, 2019, 11, 43393–43408 CrossRef CAS.
- C. F. Deng, Q. Zhang, M. D. Jia, J. Zhao, X. Sun, T. Gong and Z. R. Zhang, Adv. Sci., 2019, 6, 1801868 CrossRef.
- Y. J. Liu, Y. Lu, X. H. Zhu, C. Li, M. M. Yan, J. Pan and G. L. Ma, Biomaterials, 2020, 242, 119933 CrossRef CAS.
- Y. Ye, J. Wang, Q. Hu, G. M. Hochu, H. Xin, C. Wang and Z. Gu, ACS Nano, 2016, 10, 8956–8963 CrossRef CAS.
- Q. Li, D. Zhang, J. Zhang, Y. Jiang, A. Song, Z. Li and Y. Luan, Nano Lett., 2019, 19, 6647–6657 CrossRef CAS.
- X. M. Qian, Z. D. Shi, H. Z. Qi, M. Zhao, K. Huang, D. L. Han, J. H. Zhou, C. Y. Liu, Y. Liu, Y. F. Lu, X. B. Yuan, J. Zhao and C. S. Kang, Theranostics, 2019, 9, 7616–7627 CrossRef CAS.
- D. Liu, B. Chen, Y. Mo, Z. Wang, T. Qi, Q. Zhang and Y. Wang, Nano Lett., 2019, 19, 6964–6976 CrossRef CAS.
- X. Duan, C. Chan, W. Han, N. Guo, R. R. Weichselbaum and W. Lin, Nat. Commun., 2019, 10, 1899 CrossRef.
- H. Deng, Z. Zhou, W. Yang, L.-s. Lin, S. Wang, G. Niu, J. Song and X. Chen, Nano Lett., 2020, 20, 1928–1933 CrossRef CAS.
- Y. Yang, Q. Chen, J.-P. Wu, T. B. Kirk, J. Xu, Z. Liu and W. Xue, ACS Appl. Mater. Interfaces, 2018, 10, 12463–12473 CrossRef CAS.
- J. Sun, Y. Chen, Y. Huang, W. Zhao, Y. Liu, R. Venkataramanan, B. Lu and S. Li, Acta Pharmacol. Sin., 2017, 38, 823–834 CrossRef CAS.
- K. Cheng, Y. Ding, Y. Zhao, S. Ye, X. Zhao, Y. Zhang, T. Ji, H. Wu, B. Wang, G. J. Anderson, L. Ren and G. Nie, Nano Lett., 2018, 18, 3250–3258 CrossRef CAS.
- D.-W. Zheng, J.-L. Chen, J.-Y. Zhu, L. Rong, B. Li, Q. Lei, J.-X. Fan, M.-Z. Zou, C. Li, S.-X. Cheng, Z. Xu and X.-Z. Zhang, Nano Lett., 2016, 16, 4341–4347 CrossRef CAS.
- L. L. Dai, X. Li, M. J. Yao, P. Y. Niu, X. C. Yuan, K. Li, M. W. Chen, Z. X. Fu, X. L. Duan, H. B. Liu, K. Y. Cai and H. Yang, Biomaterials, 2020, 241, 119901 CrossRef CAS.
- B. Feng, F. Y. Zhou, B. Hou, D. G. Wang, T. T. Wang, Y. L. Fu, Y. T. Ma, H. J. Yu and Y. P. Li, Adv. Mater., 2018, 30, 1803001 CrossRef.
- B. Feng, B. Hou, Z. A. Xu, M. Saeed, H. J. Yu and Y. P. Li, Adv. Mater., 2019, 31, 1902960 CrossRef CAS.
- Z. Z. Zhang, Q. X. Wang, Q. Liu, Y. D. Zheng, C. X. Zheng, K. K. Yi, Y. Zhao, Y. Gu, Y. Wang, C. Wang, X. Z. Zhao, L. Q. Shi, C. S. Kang and Y. Liu, Adv. Mater., 2019, 31, 1905751 CrossRef CAS.
- H. T. Ruan, Q. Y. Hu, D. Wen, Q. Chen, G. J. Chen, Y. F. Lu, J. Q. Wang, H. Cheng, W. Y. Lu and Z. Gu, Adv. Mater., 2019, 31, 1806957 CrossRef.
- S. Ma, W. Song, Y. Xu, X. Si, S. Lv, Y. Zhang, Z. Tang and X. Chen, Nano Lett., 2020, 20, 2514–2521 CrossRef CAS.
- J. Kuang, W. Song, J. Yin, X. Zeng, S. Han, Y. P. Zhao, J. Tao, C. J. Liu, X. H. He and X. Z. Zhang, Adv. Funct. Mater., 2018, 28, 1800025 CrossRef.
- X. L. Hu, B. Hou, Z. A. Xu, M. Saeed, F. Sun, Z. M. Gao, Y. Lai, T. Zhu, F. Zhang, W. Zhang and H. J. Yu, Adv. Sci., 2020, 7, 1903332 CrossRef CAS.
- A. Gao, B. Chen, J. Gao, F. Zhou, M. Saeed, B. Hou, Y. Li and H. Yu, Nano Lett., 2020, 20, 353–362 CrossRef CAS.
- W. Q. Yu, X. Q. He, Z. H. Yang, X. T. Yang, W. Xiao, R. Liu, R. Xie, L. Qin and H. L. Gao, Biomaterials, 2019, 217, 119309 CrossRef CAS.
- B. Hou, L. Zhou, H. Wang, M. Saeed, D. G. Wang, Z. A. Xu, Y. P. Li and H. J. Yu, Adv. Mater., 2020, 32, 1907210 CrossRef CAS.
Footnote |
† These authors contributed equally. |
|
This journal is © The Royal Society of Chemistry 2021 |
Click here to see how this site uses Cookies. View our privacy policy here.