DOI:
10.1039/D1SC02075G
(Edge Article)
Chem. Sci., 2021,
12, 8859-8864
Tandem aza-Heck Suzuki and carbonylation reactions of O-phenyl hydroxamic ethers: complex lactams via carboamination†
Received
13th April 2021
, Accepted 27th May 2021
First published on 27th May 2021
Abstract
The palladium-catalysed tandem aza-Heck–Suzuki and aza-Heck–carbonylation reactions of O-phenyl hydroxamic ethers are reported. These formal alkene carboamination reactions provide highly versatile access to wide range complex, stereogenic secondary lactams and exhibit outstanding functional group tolerance and high diastereoselectivity.
Introduction
Nitrogen-containing heterocycles are versatile moieties for the synthesis of natural products and bioactive molecules.1 In particular, γ-lactams are both highly prevalent and also extremely valuable intermediates for the synthesis of a variety of other heterocycles.2 Simultaneously, the incorporation of stereocenters into drug-like compounds has been shown important for improvement of selectivity and other properties.3 These facts have combined to create high demand for new methods that can rapidly access stereogenic γ-lactams and other nitrogen heterocycles.
Aza-Heck cyclizations,4,5 which employ an umpolung strategy involving palladium-catalysed activation of an electrophilic nitrogen followed by cyclization onto a pendant alkene, have proven to be a powerful method for construction of stereogenic nitrogen heterocycles. When sequenced with other cross-coupling steps,6 tandem aza-Heck cyclizations present a powerful opportunity to prepare complex heterocycles in a highly convergent and modular fashion. While several of these formal carboamination reactions have been reported,7 all have been limited to the use of oxime esters as the nitrogen electrophile and only deliver cyclic ketimines.8,9 The feasibility of using other nitrogen electrophiles in tandem aza-Heck reactions has not yet been explored.
We now report that aza-Heck cyclizations of O-phenyl hydroxamic ethers5r can be sequenced with both palladium-catalysed Suzuki reactions and palladium-catalysed carbonylative amidation reactions to effect formal alkene carboamination. These reactions provide rapid access to complex, stereogenic γ-lactams with a variety of pendant functional groups (Fig. 1). In some cases, we also demonstrate that, as a result of suprafacial aminopalladation of the alkene, two stereogenic centers can arise from the process. These tandem reactions result in rapid increases of molecular complexity, providing highly valuable lactams that are rich in heteroatom content and stereogenic complexity. These reactions also open new vistas for the possibility of other classes of tandem aza-Heck reactions that are not reliant on oxime electrophiles and the myriad of heterocycles that can result.
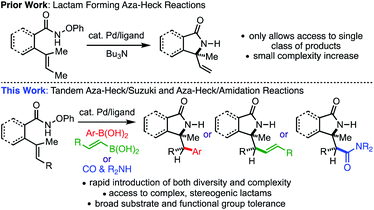 |
| Fig. 1 Carboamination via tandem aza-Heck cyclization of O-phenyl hydroxamic ethers. | |
Results and discussion
At the outset, the tandem aza-Heck/Suzuki reaction involving N-phenoxy-benzamide (1) and phenylboronic acid (2) was chosen as the model system for condition development (Table 1). Starting with conditions from our prior work on O-phenyl hydroxamic ether cyclization [(COD)Pd(CH2SiMe3)2, tris(2,2,2-trifluoroethyl) phosphite, Bu3N in MeCN],5r we were pleased to see small a amount of desired product 3, however the yield was low (18%) and significant a amount of uncyclized primary amide 4 was also observed (Table 1, entry 1). Gratifyingly, omission of the base, which was critical for good yields in prior aza-Heck reactions of this class of electrophiles,5r resulted in significantly higher yield of 3 (74%) and less byproduct 4 (entry 2). The use of other solvents was explored, but none proved superior to MeCN.10,11 Based on data presented below, it is particularly notable that dioxane was far inferior in this regard (entry 3). Addition of 4 Å molecular sieves (4 Å MS) further decreased the production of 4 and improved the yield of 3 (entry 4). Other desiccants were less effective.10 Finally, as (COD)Pd(CH2SiMe3)2 has limitations with respect to commercial supply and thermal stability, we sought to identify a more general Pd-precatalyst. While several were found to work,10 Pd(OAc)2 proved the most effective (entry 5). Under these conditions, catalyst loading could be halved, and the reaction concentration increased, without negative impact (entry 6). We believe that the reason that Pd(OAc)2 is effective in this transformation, and was not in the earlier aza-Heck reaction is due to the presence of phenyl boronic acid 2. Aryl boronic acids have been shown to be an effective reductant for Pd(II) precatalysts.12
Table 1 Optimization of Aza-Heck–Suzuki reactiona

|
Entry |
Pd catalyst [mol%] |
Solvent |
Additive |
Yield 3b [%] |
Unless otherwise noted, reactions run with 0.2 mmol 1, 0.6 mmol 2, 10 mmol% Pd catalyst and 30 mmol% P(OCH2CF3)3 at 0.05 M.
Yield calculated by 1H NMR with 1,3,5-trimethoxybenzene as internal standard.
5 mol% Pd(OAc)2 and 15 mmol% P(OCH2CF3)3 at 0.1 M.
|
1 |
(COD)Pd(CH2SiMe3)2 [10] |
MeCN |
Bu3N |
18 |
2 |
(COD)Pd(CH2SiMe3)2 [10] |
MeCN |
— |
74 |
3 |
(COD)Pd(CH2SiMe3)2 [10] |
Dioxane |
— |
15 |
4 |
(COD)Pd(CH2SiMe3)2 [10] |
MeCN |
4 Å MS |
81 |
5 |
Pd(OAc)2 [10] |
MeCN |
4 Å MS |
81 |
6c |
Pd(OAc)2 [5] |
MeCN |
4 Å MS |
81 |
The scope of reaction was next explored, and the model substrate 3 was obtained in 88% isolated yield (Scheme 1). High yields were observed with a wide range of functional groups on the phenylboronic acid (5–10). Notably, this includes aryl bromides and chlorides, as well as nitroarenes,13 all of which are also competent electrophiles in cross-coupling reactions. Vinylboronic acids also can be used, leading to products with a variety of alkene substitutions (11–13). It is also notable that these alkenyl products are one carbon homologs of the alkenes that can be prepared by direct aza-Heck reaction. Heterocyclic boronic esters were also well tolerated. With the exception of unencumbered pyridines, a wide variety of such compounds could be coupled in good yields, leading to polyheterocyclic compounds (14–22). These include protic groups (14). Also as exemplified by 14, the use of (COD)Pd(CH2SiMe3)2 as precatalyst was preferred in these cases.
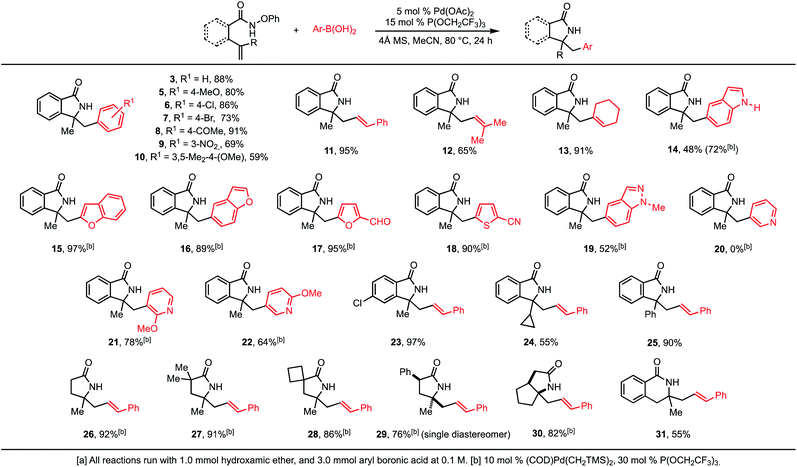 |
| Scheme 1 Substrate scope of Aza-Heck–Suzuki reaction.a | |
The nature of the hydroxamic ether could also be varied. This includes substitution on the aromatic ring (23), and modulation of the alkene substituents (24–25), as well as more dramatic changes (26–31). In the cases of 29 and 30, single diastereomers of product were observed. NMR studies confirmed the relative stereochemistry as shown in Scheme 1.10 Finally, in some cases, 6-exo aza-Heck cyclizations can also be included in the tandem sequence (31).
In general, stability of the putative alkyl palladium intermediate dictates which substrates are compatible with the tandem aza-Heck/Suzuki reaction. In substrates where the initial cyclization results in an alkyl palladium complex that can rapidly undergo β-hydride elimination, the tandem sequence was not successful. For example, when substrate 32 was subjected to the tandem aza-Heck/Suzuki conditions, the only cyclization product that was observed was alkene 33 (eqn (1)).14
In the cases shown in Table 1, β-hydride elimination is prevented by the formation of neopentylic palladium alkyl intermediates that lack β-hydrogen atoms. However, success can also be realized in cases where β-hydride elimination is possible but the alkyl palladium intermediate is stabilized against it. Examples are shown in Scheme 2. First, in the case of the cyclization of norbornene 35, a single diastereomer of the polycyclic product 36 was observed (Scheme 2, top). The stereochemistry of the product (established by X-ray crystallographic analysis) indicates that the reaction proceeds via suprafacial aminopalladation followed by stereoretentive cross-coupling, which is consistent with an aza-Heck/Suzuki pathway. In this case, β-hydride elimination from alkyl palladium intermediate 37 is disfavored, as elimination of Ha would be forced to proceed via an antarafacial pathway and elimination of Hb would result in an anti-Bredt olefin.
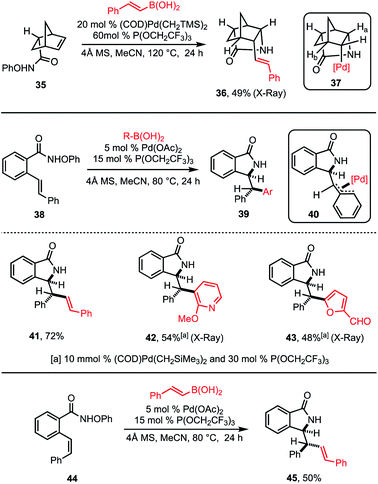 |
| Scheme 2 Formation of vicinal stereocenters via Aza-Heck–Suzuki reaction. | |
In the second example, styrenyl substate 38 was also found to participate in a tandem aza-Heck–Suzuki reaction without competing β-hydride elimination to provide a single diastereoisomer of 39 in which two new stereogenic centers are formed (Scheme 2, middle). As in the previous case, the stereochemistry of the observed products (also established by X-ray crystallographic analysis) is consistent with suprafacial aminopalladation followed by stereoretentive cross-coupling. In this case, the stabilization of the alkyl palladium intermediate afforded by the benzallyl fragment in intermediate 40 appears sufficient to allow transmetallation by the aryl boronic acid to out compete β-hydride elimination. It is notable that both alkenyl and (hetero)aryl boronic acids were capable of participating in this transformation (41–43). Finally, it is likewise notable that substrate 44, which bears the opposite alkene configuration, led to the diastereomeric product 45. This result is also consistent with the proposed aza-Heck–Suzuki pathway.
In addition to tandem aza-Heck–Suzuki reactions, we were interested in exploring other classes of tandem reactivity. Tandem aza-Heck–carbonylation reactions were very attractive in this regard due to the versatility and variety of products that such a reaction could afford. To explore the possibility, the reaction of model substrate 1 and a variety of simple amines under an atmosphere of CO was explored (Table 2). We were initially pleased to find that when morpholine was used as the nucleophile, the conditions developed for tandem Suzuki reactions provided high yields of the amidolactam product 46 (entry 1). However, the reaction yield proved to be highly dependent on the nucleophile employed; other simple amines provided unacceptably low yields under these conditions (entries 2–3). Fortunately, we found that by switching the solvent from MeCN to dioxane, uniformly good yields of products were observed (entries 4–6). This is notable, as dioxane was a particularly poor solvent for the tandem Suzuki reactions (see Table 1).15 In addition, when using dioxane, molecular sieves proved both unnecessary and slightly deleterious. As such, they were omitted from the tandem carbonylation reactions.
Table 2 Optimization of Aza-Heck–carbonylation reactiona

|
Entry |
Solvent |
Amine |
Additive |
Yield 46b [%] |
Unless otherwise noted, reactions run with 0.2 mmol 1 and 0.3 mmol amine.
Yields calculated by 1H NMR with 1,3,5-trimethoxybenzene as internal standard.
|
1 |
MeCN |
Morpholine |
4 Å MS |
81 |
2 |
MeCN |
n-Bu2NH |
4 Å MS |
36 |
3 |
MeCN |
BnNH2 |
4 Å MS |
12 |
4 |
Dioxane |
Morpholine |
— |
87 |
5 |
Dioxane |
n-Bu2NH |
— |
82 |
6 |
Dioxane |
BnNH2 |
— |
80 |
With optimized conditions in hand, we then explored the scope of the tandem aza-Heck–carbonylation reaction (Scheme 3). A wide variety of primary, cyclic secondary, and acyclic secondary amines participate in the reaction and afford amidolactams in good to excellent yields. Aryl fluorides, chlorides, and bromides are all tolerated (50 and 51), as are ethers and esters (51 and 52). Heterocycles were also highly compatible (47, 55–59). Aniline could be used as the nucleophile (53), but in this case specialized conditions were required.10 Unfortunately, the attempted use of various alcohols led to mixtures of products containing both the desired esters and phenolic esters resulting from the incorporation of the co-generated PhOH in poor yield (not shown). However, acyl hydrazines did prove effective alternatives to amines (54).16 In some cases, substates that could potentially undergo β-hydride elimination after the aza-Heck step could be utilized (55). In these cases, increased pressure of CO was required for migratory insertion to outcompete alkene formation. Finally, as with the tandem Suzuki reactions above, the structure of the hydroxamic ether could be varied (56–59), although slightly elevated temperatures were required. Overall, this provides rapid and efficient entry to diverse amidolactams.
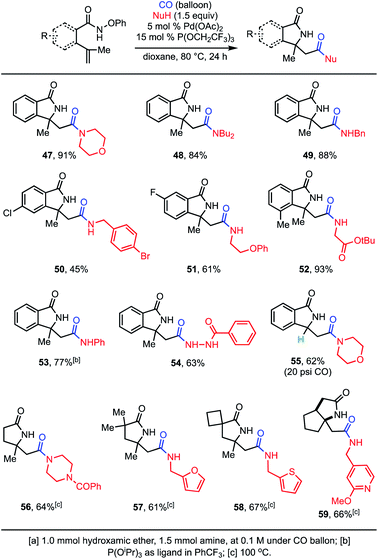 |
| Scheme 3 Scope of Aza-Heck–carbonylation reaction.a | |
Based upon the data presented herein, we believe these reactions proceed via the mechanism outlined in Fig. 2. Initial oxidative addition by an active Pd(0) complex results in Pd(II) complex 61. Capture of this intermediate, boronic acid (Schemes 1 and 2) or CO/amine (Scheme 3), and subsequent reductive elimination then results in the product. Notably, in cases where two stereocenters are formed, diastereoselectivity is consist with suprafacial aminopalladation and stereoretentive transmetallation, consistent with a Heck-type mechanism.
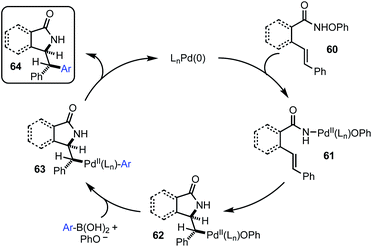 |
| Fig. 2 Proposed mechanism. | |
Conclusions
In summary, we have developed effective conditions for lactam-forming tandem aza-Heck–Suzuki and aza-Heck–carbonylation reactions. The scope for both of these formal carboamination reaction is large, and the reactions lead to a variety of complex stereogenic γ-lactams that are relevant to the preparation of bioactive molecules. All observations in this study, including the relative stereochemistry in cases where multiple stereocenters were formed, are consistent with a Heck-type mechanism in which the alkyl palladium intermediate is captured in a subsequent cross-coupling reaction. These transformations also demonstrate the feasibility of non-oxime aza-Heck reactions to participate in tandem processes. Further studies in our laboratory are underway to expand these processes to other cases of complex hetereocycles.
Author contributions
R.-D. G. and S. A. S. contributed to the experimental work. All authors contributed to ideation and writing of the paper.
Conflicts of interest
There are no conflicts to declare.
Acknowledgements
The University of Delaware (UD) and the NIH NIGMS P20GM104316 are gratefully acknowledged for support. Dr Glenn Yap is thanked for help with crystallography. Data was acquired at UD on instruments obtained with the assistance of NSF and NIS funding (NSF CHE0421224, CHE0840401, and CHE1048367; NIH P20GM104316, P30 GM110758, S10 RR02692, and S10 OD016267).
References
-
(a) A. Gomtsyan, Chem. Heterocycl. Compd., 2012, 48, 7–10 CrossRef CAS;
(b)
J. A. Joule, in Advances in Heterocyclic Chemistry, ed. E. F. V. Scriven and C. A. Ramsden, Academic Press, 2016, vol. 119, pp. 81–106 Search PubMed;
(c)
J. A. Joule and K. Mills, Heterocyclic Chemistry, Wiley-Blackwell, Chichester, 5th edn, 2010 Search PubMed.
- J. Caruano, G. G. Muccioli and R. Robiette, Org. Biomol. Chem., 2016, 14, 10134–10156 RSC.
-
(a) F. Lovering, J. Bikker and C. Humblet, J. Med. Chem., 2009, 52, 6752–6756 CrossRef CAS PubMed;
(b) M. Aldeghi, S. Malhotra, D. L. Selwood and A. W. E. Chan, Chem. Biol. Drug Des., 2014, 83, 450–461 CrossRef CAS PubMed.
- For recent reviews of aza-Heck reactions see:
(a) K. Narasaka and M. Kitamura, Eur. J. Org. Chem., 2005, 2005, 4505–4519 CrossRef;
(b) N. J. Race, I. R. Hazelden, A. Faulkner and J. F. Bower, Chem. Sci., 2017, 8, 5248–5260 RSC;
(c) B. Vulovic and D. A. Watson, Eur. J. Org. Chem., 2017, 4996–5009 CrossRef CAS PubMed;
(d) K. M. Korch and D. A. Watson, Chem. Rev., 2019, 119, 8192–8228 CrossRef CAS PubMed.
-
(a) H. Tsutsui and K. Narasaka, Chem. Lett., 1999, 28, 45–46 CrossRef;
(b) H. Tsutsui and K. Narasaka, Chem. Lett., 2001, 30, 526–527 CrossRef;
(c) M. Kitamura, S. Chiba, O. Saku and K. Narasaka, Chem. Lett., 2002, 31, 606–607 CrossRef;
(d) H. Tsutsui, M. Kitamura and K. Narasaka, Bull. Chem. Soc. Jpn., 2002, 75, 1451–1460 CrossRef CAS;
(e) J. Helaja and R. Göttlich, Chem. Commun., 2002, 720–721 RSC;
(f) S. Chiba, M. Kitamura, O. Saku and K. Narasaka, Bull. Chem. Soc. Jpn., 2004, 77, 785–796 CrossRef CAS;
(g) S. Zaman, K. Mitsuru and A. D. Abell, Org. Lett., 2005, 7, 609–611 CrossRef CAS PubMed;
(h) M. Kitamura, H. Yanagisawa, M. Yamane and K. Narasaka, Heterocycles, 2005, 65, 273–277 CrossRef CAS;
(i) A. Fürstner, K. Radkowski and H. Peters, Angew. Chem., Int. Ed., 2005, 44, 2777–2781 CrossRef PubMed;
(j) M. Kitamura, D. Kudo and K. Narasaka, ARKIVOC, 2006, 148–162 Search PubMed;
(k) J. Ichikawa, R. Nadano and N. Ito, Chem. Commun., 2006, 4425–4427 RSC;
(l) A. Faulkner and J. F. Bower, Angew. Chem., Int. Ed., 2012, 51, 1675–1679 CrossRef CAS PubMed;
(m) A. Faulkner, J. S. Scott and J. F. Bower, Chem. Commun., 2013, 49, 1521–1523 RSC;
(n) N. J. Race and J. F. Bower, Org. Lett., 2013, 15, 4616–4619 CrossRef CAS PubMed;
(o) A. Faulkner, J. S. Scott and J. F. Bower, J. Am. Chem. Soc., 2015, 137, 7224–7230 CrossRef CAS PubMed;
(p) I. R. Hazelden, X. Ma, T. Langer and J. F. Bower, Angew. Chem., Int. Ed., 2016, 55, 11198–11202 CrossRef CAS PubMed;
(q) N. J. Race, A. Faulkner, M. H. Shaw and J. F. Bower, Chem. Sci., 2016, 7, 1508–1513 RSC;
(r) S. A. Shuler, G. Yin, S. B. Krause, C. M. Vesper and D. A. Watson, J. Am. Chem. Soc., 2016, 138, 13830–13833 CrossRef CAS PubMed;
(s) N. J. Race, A. Faulkner, G. Fumagalli, T. Yamauchi, J. S. Scott, M. Rydén-Landergren, H. A. Sparkes and J. F. Bower, Chem. Sci., 2017, 8, 1981–1985 RSC;
(t) X. Bao, Q. Wang and J. Zhu, Angew. Chem., Int. Ed., 2017, 56, 9577–9581 CrossRef CAS PubMed;
(u) I. R. Hazelden, R. C. Carmona, T. Langer, P. G. Pringle and J. F. Bower, Angew. Chem., Int. Ed., 2018, 57, 5124–5128 CrossRef CAS PubMed;
(v) X. Ma, I. R. Hazelden, T. Langer, R. H. Munday and J. F. Bower, J. Am. Chem. Soc., 2019, 141, 3356–3360 CrossRef CAS PubMed;
(w) F. Xu, S. A. Shuler and D. A. Watson, Angew. Chem., Int. Ed., 2018, 57, 12081–12085 CrossRef CAS PubMed;
(x) F. Xu, K. M. Korch and D. A. Watson, Angew. Chem., Int. Ed., 2019, 58, 13448–13451 CrossRef CAS PubMed;
(y) H.-C. Shen, Y. Chen, Y. Zhang, H.-M. Jiang, W.-Q. Zhang, W.-A. Li, M. Sayed, X. Zhang, Y.-D. Wu and L.-Z. Gong, CCS Chem., 2021, 3, 421–430 CrossRef.
-
(a) T. Vlaar, E. Ruijter and R. V. A. Orru, Adv. Synth. Catal., 2011, 353, 809–841 CrossRef CAS;
(b)
L. F. Tietze and L. M. Levy, in The Mizoroki–Heck Reaction, 2009, pp. 281–344 Search PubMed.
-
(a)
J. P. Wolfe, in Synthesis of Heterocycles via Metal-Catalyzed Reactions that Generate One or More Carbon-Heteroatom Bonds, 2013, ch. 98, pp. 1–37 CrossRef;
(b) Z. J. Garlets, D. R. White and J. P. Wolfe, Asian J. Org. Chem., 2017, 6, 636–653 CrossRef CAS PubMed.
-
(a) M. Kitamura, S. Zaman and K. Narasaka, Synlett, 2001, 974–976 CrossRef CAS;
(b) S. Zaman, M. Kitamura and K. Narasaka, Bull. Chem. Soc. Jpn., 2003, 76, 1055–1062 CrossRef CAS;
(c) S. Zaman, M. Kitamura and A. D. Abell, Aust. J. Chem., 2007, 60, 624–626 CrossRef CAS;
(d) M. Kitamura, Y. Moriyasu and T. Okauchi, Synlett, 2011, 2011, 643–646 CrossRef;
(e) C. Chen, L. Hou, M. Cheng, J. Su and X. Tong, Angew. Chem., Int. Ed., 2015, 54, 3092–3096 CrossRef CAS PubMed;
(f) A. Faulkner, J. S. Scott and J. F. Bower, J. Am. Chem. Soc., 2015, 137, 7224–7230 CrossRef CAS PubMed.
- For related reactions that proceed via aza-radical intermediates see:
(a) M. Bingham, C. Moutrille and S. Z. Zard, Heterocycles, 2014, 88, 953–960 CrossRef CAS;
(b) T. Shimbayashi, D. Nakamoto, K. Okamoto and K. Ohe, Org. Lett., 2018, 20, 3044–3048 CrossRef CAS PubMed;
(c) J. Davies, N. S. Sheikh and D. Leonori, Angew. Chem., Int. Ed., 2017, 56, 13361–13365 CrossRef CAS PubMed;
(d) C. Chen, Y. Bao, J. Zhao and B. Zhu, Chem. Commun., 2019, 55, 14697–14700 RSC;
(e) C. Chen, J. Ding, Y. Wang, X. Shi, D. Jiao and B. Zhu, Tetrahedron Lett., 2019, 60, 151000 CrossRef CAS;
(f) L. Wang and C. Wang, J. Org. Chem., 2019, 84, 6547–6556 CrossRef CAS PubMed;
(g) Y. Wang, J. Ding, J. Zhao, W. Sun, C. Lian, C. Chen and B. Zhu, Org. Chem. Front., 2019, 6, 2240–2244 RSC;
(h) L. Feng, L. Guo, C. Yang, J. Zhou and W. Xia, Org. Lett., 2020, 22, 3964–3968 CrossRef CAS PubMed;
(i) J.-L. Tu, J.-L. Liu, W. Tang, M. Su and F. Liu, Org. Lett., 2020, 22, 1222–1226 CrossRef CAS PubMed;
(j) Y. Zhang, Z. Yin, H. Wang and X.-F. Wu, Chem. Commun., 2020, 56, 7045–7048 RSC;
(k) X. Shen, C. Huang, X.-A. Yuan and S. Yu, Angew. Chem., Int. Ed., 2021, 60, 9672–9679 CrossRef CAS PubMed;
(l) M. Zhang, Z. Zhang, Y. He, T. Zou, Z. Qi, Q. Fu, J. Wei, J. Lu, S. Wei and D. Yi, Adv. Synth. Catal., 2021, 363, 2110–2116 CrossRef CAS.
- See ESI†.
- Other ligands were also explored but none prove effective.
- M. Moreno-Mañas, M. Pérez and R. Pleixats, J. Org. Chem., 1996, 61, 2346–2351 CrossRef.
-
(a) F. Inoue, M. Kashihara, M. R. Yadav and Y. Nakao, Angew. Chem., Int. Ed., 2017, 56, 13307–13309 CrossRef CAS PubMed;
(b) M. R. Yadav, M. Nagaoka, M. Kashihara, R.-L. Zhong, T. Miyazaki, S. Sakaki and Y. Nakao, J. Am. Chem. Soc., 2017, 139, 9423–9426 CrossRef CAS PubMed.
- It is notable that in this case, the yield of 33 is significantly lower than what we have previously observed in the absence of the boronic acid, and is due to the formation of significant formation of primary amide. This is possibly due to the formation of water from dehydration of the boronic acid via boroxine formation. See: Y. Tokunaga, H. Ueno, Y. Shimomura and T. Seo, Heterocycles, 2002, 57, 787–790 CrossRef CAS.
- As the solubilities of CO in dioxane and MeCN are reported to be similar, we speculate that this difference is likely due to the better activation of the boronic acid in the more polar MeCN. See:
(a) E. Veleckis and D. S. Hacker, J. Chem. Eng. Data, 1984, 29, 36–39 CrossRef CAS;
(b) Z. K. Lopez-Castillo, S. N. V. K. Aki, M. A. Stadtherr and J. F. Brennecke, Ind. Eng. Chem. Res., 2006, 45, 5351–5360 CrossRef CAS.
- It is notable that N–N bond cleavage was not observed under these conditions.
Footnote |
† Electronic supplementary information (ESI) available: Experimental details, crystallographic and characterization data. CCDC 2071160–2071162. For ESI and crystallographic data in CIF or other electronic format see DOI: 10.1039/d1sc02075g |
|
This journal is © The Royal Society of Chemistry 2021 |
Click here to see how this site uses Cookies. View our privacy policy here.