High-performance near-infrared (NIR) polymer light-emitting diodes (PLEDs) based on bipolar Ir(III)-complex-grafted polymers†
Received
14th September 2020
, Accepted 11th November 2020
First published on 13th November 2020
Abstract
Despite the cost-effective and large-area scalable advantages of NIR-PLEDs based on iridium(III)-complex-doped polymers, the intrinsic phase-separation issue leading to inferior device performance is difficult to address. In this study, taking the vinyl-functionalized [Ir(iqbt)2(vb-ppy)] (Hqibt = 1-(benzo[b]-thiophen-2-yl)-isoquinoline; vb-Hppy = 2-(4′-vinylbiphenyl-4-yl)pyridine) as the polymerized complex monomer, two series of Ir(III)-complex-grafted polymers Poly(NVK-co-[Ir(iqbt)2(vb-ppy)]) and Poly((vinyl-PBD)-co-NVK-co-[Ir(iqbt)2(vb-ppy)]) (NVK = N-vinyl-carbazole; vinyl-PBD = 2-(4-(tert-butyl)phenyl)-5-(4′-vinyl-[1,1′-biphenyl]-4-yl)-2,5-dihydro-1,3,4-oxadiazole) are obtained, respectively. Moreover, by using the bipolar Ir(III)-complex-grafted polymer further doped or grafted with an electron-transport unit as the emitting layer (EML), reliable NIR-PLEDs are realized. In particular based on the concurrent covalent-linkages of both the Ir(III)-complex and the vinyl-PBD towards the carrier-balanced NIR-PLED-III, the achievement of an almost negligible (<5%) efficiency roll-off does not sacrifice the attractive efficiency (ηmaxEQE = 3.6%). This finding makes bipolar Ir(III)-complex-grafted polymers a good platform to achieve high-performance NIR-PLEDs.
1. Introduction
Driven by the promising applications of near-infrared (NIR) organic/polymer light-emitting diodes (NIR-OLEDs/PLEDs) in night-vision and information-security displays,1 telecommunications2 and photo-dynamic therapies,3 concerted efforts have been devoted to the development of new and efficient NIR-emitters. In this context, owing to the harvesting of both singlet and triplet excitons towards a theoretical ηIQE (internal quantum efficiency) of 100%, NIR-emissive transition-metal (Pt(II), Ir(III) or Os(II), etc.) complex phosphors4 together with TADF (thermal activated delayed fluorescence) molecules5 capable of the facilitated reverse intersystem crossing (RISC) are highly attractive. Importantly, the octahedral Ir(III)-complexes with rather short triplet lifetimes and high efficiency which is competitive to those of other triplet-utilized counterparts, together with the superiority of a significantly alleviated efficiency roll-off, have particular appeal for application in NIR-OLEDs/PLEDs.
Until now, concrete C^N-cyclometalated Ir(III)-complexes possessing neutral [Ir(C^N)3]-homoleptic6 or [Ir(C^N)2(L^X)]-heteroleptic (L^X = O^O7 or N^O8) and cationic [Ir(C^N)2(N^N)]+ forms9 have been demonstrated for developing reliable NIR-OLEDs/PLEDs, and the wavelength–ηEQE (external quantum efficiency) relationship is summarized in Fig. 1 and Table S1 (ESI†). Nonetheless, as constrained by the so-called “energy gap law”,10 it remains a real challenge to develop new Ir(III)-complex-based NIR-emitters to achieve high efficiency. On the other hand, to suppress the detrimental triplet–triplet annihilation (TTA)11 of the Ir(III)-complex-based phosphors with narrow HOMO–LUMO band-gaps for the NIR emissions, it is necessary and also challenging to dope one specific Ir(III)-complex into an appropriate small-molecule host for the vacuum-deposited/solution-processed NIR-OLED (NIR-OLEDs-V/S) or polymeric matrix for the NIR-PLEDs (Table S1, ESI† and Fig. 1), respectively. In comparison, although cost-effective solution-processed NIR-PLEDs with Ir(III)-complex-doped polymers as the EMLs are more advantageous for the large-area scalability, the simple doping suffers from an inevitable phase-separation issue,12 thereby leading to inferior device efficiency and serious efficiency roll-off. Noticeably, despite the certain efficiency progress appreciable from the supplementation of one electron-transport layer (ETL) towards the facilitated carriers’ balance for some multi-layer NIR-PLEDs,12 the issues of device instability and undesirable efficiency-roll-off caused by the heterogeneity of the Ir(III)-complex-doping polymer systems are still difficult to address.
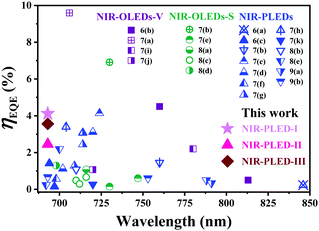 |
| Fig. 1 The λem-relative ηEQE comparison between the NIR-PLEDs-I–III in this work with those from Ir3+-complexes doping in small-molecular or polymer host with vacuum-deposition (NIR-OLEDs-V) or solution-processing (NIR-OLEDs-S/PLEDs). | |
To circumvent such problems, to some extent, we can rely on a conceptual approach to use Ir(III)-complex-grafted polymers in solution-processed multi-layer NIR-PLEDs. On one hand, benefiting from the covalent-bonding linkage, the NIR-emitting Ir(III)-complexes are molecularly dispersed into a hole-transporting polymer host with a uniform phase. Meanwhile, further through the doping or grafting of the electron-transport molecule, the resultant Ir(III)-complex-grafted polymers could exhibit a bipolar (electron/hole-transport ability) nature. In particular, through the smooth feeding ratio tuning of both the Ir(III)-complex and electron-transport molecule into the hole-transport polymer matrix, much room can be achieved to facilely reform the carrier's balance within the bipolar polymer towards an optimized optoelectronic feature. Noticeably, although bipolar Ir(III)-complex-grafted polymers capable of showing monochromatic13 or panchromatic14 emission in the visible-light range are achieved, no examples of their fabrication for NIR-PLEDs, to our knowledge, have been reported. Herein, taking the NIR-emitting [Ir(iqbt)2(vb-ppy)] with one vinyl group as the polymerizable complex monomer, as shown in Scheme 1, two series of Ir(III)-complex-grafted polymers Poly(NVK-co-[Ir(iqbt)2(vb-ppy)]) (100
:
1, 150
:
1 or 200
:
1) and Poly((vinyl-PBD)-co-NVK-co-[Ir(iqbt)2(vb-ppy)]) (15
:
150
:
1) are obtained from its copolymerization with NVK and/or monomer vinyl-PBD with the facilitated electron-transport, respectively. Moreover, using the Ir(III)-complex-grafted polymer doped or grafted with an electron-transport molecule as the EML, respectively, the first-examples of bipolar Ir(III)-complex-grafted NIR-PLEDs are also pursued.
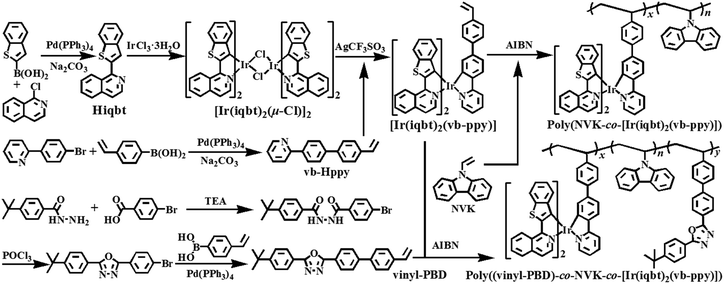 |
| Scheme 1 Synthetic scheme of the ligands Hiqbt, vb-Hppy, and vinyl-PBD, the complex monomer [Ir(iqbt)2(vb-ppy)] and their two series of Ir3+-complex-grafted polymers Poly(NVK-co-[Ir(iqbt)2(vb-ppy)]) and Poly((vinyl-PBD)-co-NVK-co-[Ir(iqbt)2(vb-ppy)]). | |
2. Experimental section
The information on starting materials and general characterization methods is provided in the ESI.† The HC^N1 main ligand Hiqbt was synthesized by the Suzuki coupling of 2-Cl-isoquinoline with benzo[b]thien-2-yl boronic acid as reported in our recent literature.8e As to the μ-chloro-bridged dimeric intermediate [Ir(iqbt)2(μ-Cl)]2, it was prepared according to the typical Nonoyama procedure.15 For the vinyl-functionalized HC^N2 ancillary ligand vb-Hppy, it was synthesized from the improved Suzuki coupling reaction of 4-vinylphenyl-boronic acid with 2-(4-bromophenyl)-pyridine as in the literature.16 The vinyl-modified electron-transport monomer vinyl-PBD was obtained through the dehydration cyclization17 and the subsequent Suzuki coupling reaction.18
Synthesis of the vinyl-functionalized complex monomer [Ir(iqbt)2(vb-ppy)]
To a solution of the μ-chloro-bridged dimeric intermediate [Ir(iqbt)2(μ-Cl)]2 (270 mg, 0.18 mmol) in mixed solvents of CH2Cl2 (8 mL) and MeOH (4 mL), the synthesized vb-Hppy (139 mg, 0.54 mmol) and AgCF3SO3 (138 mg, 0.54 mmol) were added, and the mixture was heated at 45 °C under a dry N2 atmosphere for 24 h. After cooling to room temperature, the white solid was removed and the residue was purified by column chromatography on silica gel using CH2Cl2/acetonitrile (v/v = 2
:
1) as the eluent. Yield: 35 mg (20%). Calc. for C53H34IrN3S2: C, 65.68; H, 3.54; N, 4.34%. Found: C, 65.63; H, 3.58; N, 4.30%. FT-IR (KBr, cm−1): 3051 (w), 2953 (m), 2918 (m), 2851 (m), 2359 (w), 1618 (w), 1601 (w), 1582 (w), 1558 (w), 1541 (w), 1501 (w), 1468 (w), 1452 (w), 1435 (m), 1412 (s), 1375 (w), 1360 (w), 1335 (m), 1306 (w), 1288 (w), 1273 (w), 1231 (m), 1157 (w), 1148 (w), 1124 (w), 1067 (w), 1040 (w), 1020 (w), 988 (w), 962 (w), 910 (m), 862 (w), 845 (w), 806 (m), 779 (w), 760 (m), 727 (vs), 706 (w), 687 (s), 662 (m), 633 (w), 598 (w), 565 (w), 528 (w), 500 (w). 1H NMR (400 MHz, CDCl3): δ (ppm) 9.27 (d, 1H, –Py), 8.80 (d, 1H, –Py), 8.51 (d, 1H, –Py), 8.06 (d, 1H, –Py), 8.02 (d, 1H, –Py), 7.95 (m, 7H, –Ph), 7.84 (t, 2H, –Ph), 7.69 (t, 2H, –Ph), 7.64 (d, 1H, –Py), 7.61 (d, 2H, –Ph), 7.58 (d, 1H, –Py), 7.53 (t, 2H, –Ph), 7.49 (d, 1H, –Ph), 7.39 (d, 1H, –Ph), 7.23 (d, 1H, –Ph), 7.17 (m, 2H, –Ph), 7.11 (t, 1H, –Ph), 6.88 (t, 2H, –Ph), 6.82 (t, 1H, –Ph), 6.66 (t, 1H, –CH
), 5.65 (d, 1H,
CH2), 5.15 (d,1H,
CH2). ESI-MS (in CH2Cl2) m/z: 970.21 (100%), [M + H]+.
Synthesis of the Ir3+-complex-grafted polymers Poly(NVK-co-[Ir(iqbt)2(vb-ppy)]) (100
:
1, 150
:
1 or 200
:
1)
A mixture of NVK and the complex monomer [Ir(iqbt)2(vb-ppy)] at a stipulated feed molar ratio (100
:
1, 150
:
1 or 200
:
1) in the presence of AIBN (azobis(isobutyronitrile); 1.5 mol% of NVK) was dissolved in toluene (30 mL), and the resultant homogeneous solution was purged with N2 for 10 min and sealed under a reduced N2 atmosphere. The reaction mixture was heated to 80 °C with continuous stirring for 48 h. The viscous mixture was diluted with toluene (15 mL) and precipitated with n-hexane (50 mL) three times. The resulting solid products were collected by filtration and dried at 45 °C under vacuum to constant weight, respectively. For Poly(NVK-co-Ir(iqbt)2(vb-ppy)) (150
:
1): yield: 92%. FT-IR (KBr, cm−1): 3059 (w), 2968 (w), 2934 (w), 2359 (w), 1597 (w), 1483 (m), 1450 (s), 1325 (m), 1223 (m), 1157 (w), 1124 (w), 1028 (w), 1003 (w), 926 (w), 829 (w), 745 (vs), 721 (s), 617 (w), 567 (w), 528 (w). 1H NMR (400 MHz, CDCl3): δ (ppm) 9.24 (m, 3H, –Ph), 8.10–6.04 (br, 1100H + 26H), 5.52–2.75 (br, 138H), 2.38 (b, 1H), 1.65 (b, 2H), 1.30–0.88 (b, 276H). XPS result: 0.80 mol% versus NVK. The characterization of the other Ir3+-complex-grafted polymers Poly(NVK-co-[Ir(iqbt)2(vb-ppy)]) (100
:
1 or 200
:
1) is provided in the ESI.†
Synthesis of the bipolar Ir3+-polymer Poly((vinyl-PBD)-co-NVK-co-[Ir(iqbt)2(vb-ppy)]) (15
:
150
:
1)
The bipolar Ir3+-polymer Poly((vinyl-PBD)-co-NVK-co-[Ir(iqbt)2(vb-ppy)]) (15
:
150
:
1) was synthesized in the same way as the Poly(NVK-co-[Ir(iqbt)2(vb-ppy)]) (150
:
1) except that the mixture of the organic monomer vinyl-PBD, NVK and the complex monomer [Ir(iqbt)2(vb-ppy)] at a stipulated feed molar ratio of 15
:
150
:
1 (1.5 mol% of AIBN relative to NVK) instead of the mixture of NVK and the complex monomer [Ir(iqbt)2(vb-ppy)] at a feeding ratio of 150
:
1 (1.5 mol% of AIBN relative to NVK) was adopted. For Poly((vinyl-PBD)-co-NVK-co-[Ir(iqbt)2(vb-ppy)]) (15
:
150
:
1): yield: 91%. FT-IR (KBr, cm−1): 3051 (w), 2963 (w), 2932 (w), 2354 (w), 1598 (w), 1483 (m), 1452 (s), 1333 (m), 1225 (m), 1157 (w), 1124 (w), 1027 (w), 1003 (w), 924 (w), 829 (w), 742 (vs), 723 (s), 616 (w), 568 (w), 529 (w). 1H NMR (400 MHz, CDCl3): δ (ppm) 8.13–5.93 (br, 1135H), 4.92–2.39 (b, 135H), 1.59 (s, 135H), 1.28–0.91 (b, 270H). XPS result: 0.78 mol% versus NVK.
Device designs of the doping-type NIR-PLED-I based on the Ir3+-complex monomer and the grafting-type NIR-PLEDs-II–III based on the Ir3+-polymers
Using a mixture of the complex monomer [Ir(iqbt)2(vb-ppy)] (5 wt%) and the co-host PVK
:
PBD (65
:
30, wt%; PVK = poly(N-vinyl-carbazole), PBD = (2-(4-tert-butylphenyl)-5-(4-biphenylyl)-1,3,4-oxadiazole)) as the EML, the doping-type NIR-PLED-I was fabricated with the configuration of ITO/PEDOT:PSS (40 nm)/PVK:PBD:[Ir(iqbt)2(vb-ppy)] (120 nm)/TmPyPB (15 nm)/LiF (1 nm)/Al (100 nm) for comparison. As to the grafting-type NIR-PLEDs-II–III, they were fabricated with the configurations of ITO/PEDOT:PSS (40 nm)/Poly(NVK-co-[Ir(iqbt)2(vb-ppy)]) (150
:
1):PBD (30 wt%) (120 nm)/TmPyPB (15 nm)/LiF (1 nm)/Al (100 nm) and ITO/PEDOT:PSS (40 nm)/Poly((vinyl-PBD)-co-NVK-co-[Ir(iqbt)2(vinyl-ppy)]) (15
:
150
:
1) (120 nm)/TmPyPB (15 nm)/LiF (1 nm)/Al (100 nm), respectively. Their difference lies in the usage of PVK:PBD:[Ir(iqbt)2(vb-ppy)] for the NIR-PLED-I, Poly(NVK-co-[Ir(iqbt)2(vb-ppy)]) (150
:
1):PBD for the NIR-PLED-II or Poly((vinyl-PBD)-co-NVK-co-[Ir(iqbt)2(vb-ppy)]) (15
:
150
:
1) for the NIR-PLED-III, respectively. TmPyPB (1,3,5-tri[(3-pyridyl)-phen-3-yl]benzene) was used to further promote the electron-transport ability in the NIR-PLEDs-I–III. Details of the series of NIR-PLED fabrication and their testing are presented in the ESI.†
3. Results and discussion
Synthesis, characterization and photo-physical properties of the Ir3+-complex monomer [Ir(iqbt)2(vb-ppy)]
Through the improved Suzuki coupling reaction8e of 2-Cl-isoquinoline (instead of 2-Br-isoquinoline7g) with benzo[b]thien-2-yl boronic acid, the synthesized HC^N1 main ligand Hiqbt was cyclometalated with IrCl3·nH2O to give the μ-chloro-bridged dimeric intermediate [Ir(iqbt)2(μ-Cl)]2 as specified in the literature.15 Also as shown in Scheme 1, further based on the cyclometalation of the intermediate [Ir(iqbt)2(μ-Cl)]2 with another vinyl-functionalized HC^N2 ancillary vb-Hppy by AgCF3SO3, the chloride-free, vinyl-functionalized [Ir(C^N1)2(C^N2)] complex monomer [Ir(iqbt)2(vb-ppy)] was obtained.
The vinyl-functionalized complex monomer [Ir(iqbt)2(vb-ppy)] was well characterized via EA, FT-IR, 1H NMR and ESI-MS, despite the failure to produce its single-crystals. Evidently, in the 1H NMR spectrum (Fig. S1, ESI†) of the complex monomer [Ir(iqbt)2(vb-ppy)], the stipulated molar ratio of 2
:
1 between the C^N1(iqbt)− and the C^N2(vb-ppy)− proton resonances confirms its desirable [Ir(C^N1)2(C^N2)] component. Meanwhile, attributed to the incorporation of the asymmetric vinyl-functionalized HC^N2 ancillary vb-Hppy, the point group of its complex monomer [Ir(iqbt)2(vb-ppy)] is C1, from which the two sets of doublet peaks at δ = 8.80 and 8.51 ppm can be safely assigned to the two protons on the C atoms adjacent to N atoms in the pyridyl rings of the two (iqbt)−-C^N1 ligands, respectively. Moreover, upon the Ir(III)-coordination, besides the double signal (δ = 9.27 ppm) of the proton on the C atom adjacent to N atoms in the pyridyl ring of the (vb-ppy)−-C^N2 ligand being significantly down-field shifted compared to that (δ = 8.70 ppm) for the free vb-Hppy, the slightly high-field shifts (δ = 6.66, 5.65 and 5.15 ppm) of the vinyl-terminal proton resonances for the complex monomer [Ir(iqbt)2(vb-ppy)] relative to those (δ = 6.78, 5.82 and 5.30 ppm) of the free vb-Hppy further verify the successful vinyl-modification. Furthermore, the ESI-MS result of the complex monomer [Ir(iqbt)2(vb-ppy)] exhibits the strongest mass peak at m/z 970.21 assigned to the major species [M + H]+, indicating that its [Ir(C^N1)2(C^N2)]-characteristic unit can remain stable in solution.
The photo-physical properties of the complex monomer [Ir(iqbt)2(vb-ppy)] were examined in degassed solution at RT or 77 K, and the results are summarized in Table S2 (ESI†) and Fig. 2. As shown in Fig. 2, in contrast to the limited (λab < 400 nm; Fig. S2, ESI†) absorptions of the two kinds of C^N ligands, the complex monomer [Ir(iqbt)2(vb-ppy)] exhibits significantly broadened UV-visible-NIR absorption: intense absorption bands below 420 nm from the intraligand π–π* transitions, moderate absorption bands (λab = 456, 487 (sh), 518 and 557 (sh) nm) assigned to the 1,3LLCT/1,3MLCT-admixed (LLCT = ligand-to-ligand charge transfer; MLCT = metal-to-ligand charge transfer) transitions, and weak bands extending over 600 nm probably from the S0 → T1 excitation. Upon photo-excitation at λex = 463 nm, the complex monomer [Ir(iqbt)2(vb-ppy)] displays a strong NIR emission (58% of the λem ≥ 700 nm proportion) peaking at 693 nm with a shoulder at 754 nm (Fig. 2). In contrast to the non-emissive character (λem = 415 nm for the HC^N1 ligand Hiqbt and λem = 403 nm for the HC^N2 ligand vb-Hppy; Fig. S2, ESI†) of the two C^N ligands in the NIR range, the NIR emission of the complex monomer [Ir(iqbt)2(vb-ppy)] should originate from the Ir3+-induced T1 state. Moreover, the time-decayed mono-exponential lifetime of 0.25 μs (Fig. S3, ESI†) was obtained at λem = 693 nm for the complex monomer [Ir(iqbt)2(vb-ppy)] species, confirming the intrinsic NIR-phosphorescent nature. Noticeably, the NIR-emissive lifetime (τ = 0.25 μs) is remarkably shorter than those of the heteroleptic Ir3+-complexes [Ir(iqbt)2(O^O)]7d,g or [Ir(iqbt)2(N^O)],8e which should be originated from the stronger π-backbonding effect19 due to the asymmetric C^N2-(vb-ppy) ancillary π-donor in the complex monomer [Ir(iqbt)2(vb-ppy)] with a restricted vibronic motion to the NIR-emitting excited-state. Accordingly, owing to the large radiative rate constant (kr = 7.6 × 105 s−1), its NIR-emissive efficiency of ΦPL = 0.19 is realized. Furthermore, as illustrated for the emission (85% of the λem ≥ 700 nm proportion; Fig. 2) with a well-resolved vibronic structure at 77 K, the 0–0 transition at 704 nm and the 0–1 transition at 764 nm with small bathochromic shifts compared to the RT one (Fig. 2) give a Huang–Rhys factor (SM) of 0.98, suggesting that the complex monomer [Ir(iqbt)2(vb-ppy)] has a weak geometry distortion20 of the T1 state relative to the ground state. As a result, the thermal gravimetric (TG; Fig. S4, ESI†) analysis reveals that the complex monomer [Ir(iqbt)2(vb-ppy)] exhibits a desirably good thermal stability with a comparable decomposition temperature (Td, with 5 wt% weight loss) of 384 °C to those of typical [Ir(C^N)3]-homoleptic6 complexes.
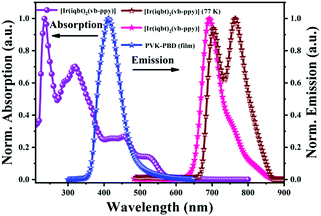 |
| Fig. 2 Normalized UV-visible-NIR absorption and emission spectra for [Ir(iqbt)2(vb-ppy)] (λex = 463 nm) in degassed CH2Cl2 solution and PVK-PBD (65 : 30, weight ratio; λex = 273 nm) in film at RT or 77 K. | |
Electronic structure calculations of the complex monomer [Ir(iqbt)2(vb-ppy)]
To explore the absorption nature of the complex monomer [Ir(iqbt)2(vb-ppy)], DFT/TD-DFT (time-dependent density functional theory) calculations based on its optimized S0 geometry were performed, and the results are summarized in Table S3 (ESI†) and Fig. 3. As shown in Fig. 3, in contrast to the almost entire contribution (92.76%) from one C^N1-(iqbt)− main ligand to the LUMO, the HOMO is mainly (51.01% and 23.06%) localized at the two C^N1-(iqbt)− main ligands and accompanied by the substantial (23.73%) contribution from the Ir(III)-centre and less (2.20%) contribution from the C^N2-(vb-ppy)− ancillary ligand. However, different from the dominant (84.71%) contribution from one C^N1-(iqbt)− main ligand and the more substantial (10.22%) contribution from the C^N2-(vb-ppy)− ancillary ligand to the LUMO+1, the LUMO+2 is predominantly (86.93%) located at the C^N2-(vb-ppy)− ancillary ligand. Meanwhile, besides the prevalent (74.44%) contribution from the two C^N1-(iqbt)− main ligands to the HOMO−2 like the HOMO−1 (97.79%), some substantial contributions from the Ir(III)-centre (14.59%) and the C^N2-(vb-ppy)− ancillary ligand (10.97%) are observed. Further checking from Table S3 (ESI†), the calculated S0 → Sn (n = 1–4) transition absorption wavelengths of the complex monomer [Ir(iqbt)2(vb-ppy)] are predicted at 558, 512, 489 and 445 nm, respectively. For the S0 → S1 transition absorption at 558 nm, the population analysis of the HOMO → LUMO (97.64%) transition verifies the partial (22.7%) 1MLCT, the substantial (21.6%) 1ILCT (intraligand charge transfer) and the dominant (51.4%) 1LLCT feature from the π orbitals of one C^N1-(iqbt)− main ligand to the π* orbitals of the other one. The calculated absorption peak at 512 nm, 489 nm or 445 nm mainly results from the corresponding HOMO → LUMO+1 (95.32%), HOMO → LUMO+2 (97.26%) or HOMO−1 → LUMO (76.73%) transition, respectively, also exhibiting the 1LLCT/1MLCT-admixed character. Hence, all the calculated absorptions featuring 1LLCT/1MLCT-admixed transitions are in good agreement with the experimental data (λab = 557, 518, 487 and 456 nm) of the complex monomer [Ir(iqbt)2(vb-ppy)] in solution. Interestingly, due to the large contribution combined from the HOMO → LUMO (59.94%) and the HOMO−1 → LUMO (30.32%) transitions to the T1 state, the experimental S0 → T1 absorption (over 600 nm) transition can be reasonably assigned to the 3ILCT/3MLCT/3LLCT-admixed transitions.
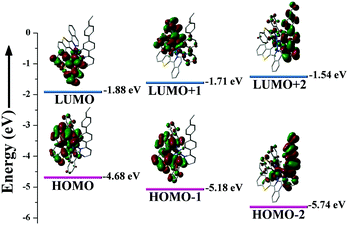 |
| Fig. 3 The HOMO and LUMO patterns for the complex monomer [Ir(iqbt)2(vb-ppy)] based on its optimized S0 geometry. | |
In order to definitely elucidate the NIR-emissive behaviour of the complex monomer [Ir(iqbt)2(vb-ppy)], natural transition orbital (NTO; Table S4 (ESI†) and Fig. 4) calculations were further performed on its optimized T1 geometry, where based on the entire (100%) hole → particle transition, the 3ILCT dominated (73.8%) and the less prevalent (13.9%) 3MLCT transitions are responsible for its NIR-emitting phosphorescence.
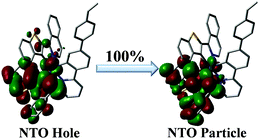 |
| Fig. 4 The NTO pattern for the T1 → S0 emission of the complex monomer [Ir(iqbt)2(vb-ppy)] based on its optimized T1 geometry. | |
Synthesis, characterization and photo-physical properties of the two series of Ir3+-complex-grafted polymers
Considering the excellent physical properties (high thermal stability, good mechanical intensity and excellent spin-coated film-formability, etc.) of the semi-conducting PVK as a popular polymer host,21 the grafting-type Ir3+-polymers Poly(NVK-co-[Ir(iqbt)2(vb-ppy)]) with different feeding ratios (100
:
1, 150
:
1 or 200
:
1) were synthesized from the AIBN-initiated copolymerization (Scheme 1) of NVK and the complex monomer [Ir(iqbt)2(vb-ppy)]. As a matter of fact, not only does the PVK host function as a hole-transport matrix, it with the significantly higher T1 level also acts as an effective energy donor to transfer energy via the Förster mechanism22 to the low energy-state Ir3+-complex-acceptor. Moreover, to further overcome the electron-transport deficiency of the Ir3+-polymers Poly(NVK-co-[Ir(iqbt)2(vb-ppy)]), another grafting-type Ir3+-polymer Poly((vinyl-PBD)-co-NVK-co-[Ir(iqbt)2(vb-ppy)]) (also Scheme 1) was designed, where through the AIBN-assisted ternary copolymerization of NVK, the complex monomer [Ir(iqbt)2(vb-ppy)] and the electron-transport monomer vinyl-PBD, the bipolar (electron/hole-transport) Ir3+-polymer Poly((vinyl-PBD)-co-NVK-co-[Ir(iqbt)2(vb-ppy)]) (15
:
150
:
1) was obtained.
To verify the AIBN-assisted radical copolymerization,23 both series of grafting-type Ir3+-polymers were characterized by FT-IR, 1H NMR and GPC (gel permeation chromatography) methods. On one hand, in the 1H NMR spectrum (Fig. S1, ESI†) of the representative Poly(NVK-co-[Ir(iqbt)2(vb-ppy)]) (150
:
1) or Poly((vinyl-PBD)-co-NVK-co-[Ir(iqbt)2(vb-ppy)]) (15
:
150
:
1), the presence of the broadened proton resonances of the polymerized [Ir(iqbt)2(vb-ppy)], NVK and/or vinyl-PBD, together with the disappearance of their original vinyl-characteristic proton resonances, indicate that the complex monomer [Ir(iqbt)2(vb-ppy)] and/or the vinyl-PBD are actually covalent-bonded into the corresponding PVK backbone. On the other hand, GPC results (Table S5, ESI†) show that all the PDIs (PDI = Mw/Mn) with different feed molar ratios for the two kinds of the grafting-type Ir3+-polymers are in a relatively narrow range (<1.30) due to the AIBN-initiated radical copolymerization.23 Moreover, with regard to the actual Ir3+-complex-grafting content, the XPS (X-ray photoelectron spectroscopy) quantitative analyses reveal that every Ir3+-complex-grafting content is found to be slightly higher than the corresponding initial feeding ratio, which probably arises from the loss of oligomeric PVK during the isolation of one specific Ir3+-polymer.24 Furthermore, the PXRD (powder X-ray diffraction) pattern (Fig. S5, ESI†) of either Poly(NVK-co-[Ir(iqbt)2(vb-ppy)]) or Poly((vinyl-PBD)-co-NVK-co-[Ir(iqbt)2(vb-ppy)]) just exhibits the PVK-based amorphous peaks, suggesting the low-concentration homogeneous dispersion of the monomers [Ir(iqbt)2(vb-ppy)] and/or vinyl-PBD into the PVK backbone. TG and DSC (differential scanning calorimetry; Fig. S4, ESI†) results of these grafting-type Ir3+-polymers show that an improved (Td; > 400 °C) thermal stability over that (384 °C) of the complex monomer [Ir(iqbt)2(vb-ppy)] and a desirable Tg (glass transition temperature) above 160 °C are observed.
The photo-physical properties of the two series of grafting-type Ir3+-polymers Poly(NVK-co-[Ir(iqbt)2(vb-ppy)]) (100
:
1, 150
:
1 or 200
:
1) and Poly((vinyl-PBD)-co-NVK-co-[Ir(iqbt)2(vb-ppy)]) (15
:
150
:
1) were investigated in solid-state or solution at RT, and the data are summarized in Table S2 (ESI†) and Fig. 5 and Fig. S6 (ESI†). As shown in Fig. S6 (ESI†), both the DR (diffuse reflection) and the solution absorption spectra of all the grafting-type Ir3+-polymers show significantly broader absorption bands than that of the PVK, in which, besides the strong absorptions below 400 nm attributed to the π–π* transitions from the organic portions of PVK and the ligands, the absorptions across the whole visible range should be assigned to the 1,3LC/1,3MLCT and S0 → T1 admixed transitions of the grafted complex monomer [Ir(iqbt)2(vb-ppy)]. Noticeably, owing to the significant spectral overlap (also Fig. 2) between the absorption of the complex monomer [Ir(iqbt)2(vb-ppy)] and the emission of PVK, effective Förster energy transfer22 should occur. Convincingly, upon photo-excitation, the resulting emissions (Fig. 4) of all the grafting-type Ir3+-polymers do not show the simple addition spectra, but they are highly related to the stipulated feeding ratio. For the Poly(NVK-co-[Ir(iqbt)2(vb-ppy)]) (100
:
1 or 150
:
1), photo-excitation gives rise to the almost entire NIR emission (λem = 696 nm), resembling that (Fig. 2) of the complex monomer [Ir(iqbt)2(vb-ppy)] in solution. The absence of the PVK-based blue-light is due to the effective Förster energy transfer22 from the PVK to the Ir3+-complex-acceptor, giving rise to the satisfactory ΦPL of 0.13 (100
:
1) or 0.16 (150
:
1). On further increasing the feeding ratio up to 200
:
1, the dual-emitting (ΦPL = 0.21) behaviour associated with the PVK-centered emission at 420 nm and the Ir3+-complex-based NIR emission (λem = 690 nm) is observed, and the 28 ns of the PVK-centered lifetime together with the Ir3+-complex-decayed lifetime of 1.29 μs further confirm the dual-emitting character (Fig. S7, ESI†). Accordingly, based on the equation25ΦET = 1 − (τDA/τD) (τDA or τD is the donor's amplitude-weighted lifetime with and without acceptor, respectively; τD = 44 ns (λem = 430 nm) for the pure PVK as in the literature26) for Poly(NVK-co-[Ir(iqbt)2(vb-ppy)]) (200
:
1), the Förster energy transfer ΦET of 36% is qualitatively estimated. For comparison, accompanied by the almost constant and mono-exponential Ir3+-complex-decayed lifetime (1.24 μs) for Poly(NVK-co-[Ir(iqbt)2(vb-ppy)]) (150
:
1) and the significantly reduced lifetime of 0.97 μs for Poly(NVK-co-[Ir(iqbt)2(vb-ppy)]) (100
:
1), the facilitated separation of the complex monomers [Ir(iqbt)2(vb-ppy)] within the PVK backbone should occur at the lower Ir3+-complex-grafting level (150
:
1 or 200
:
1), from which the undesirable aggregation-caused quenching (ACQ)27 effect from the high grafting content (100
:
1) is effectively suppressed. Interestingly, with an appropriate amount of the electron-transport vinyl-PBD further grafted for the bipolar Poly((vinyl-PBD)-co-NVK-co-[Ir(iqbt)2(vb-ppy)]) (15
:
150
:
1), besides the similar Ir3+-complex-based NIR emission (λem = 693 nm) to that of Poly(NVK-co-[Ir(iqbt)2(vb-ppy)]) (150
:
1), its typical and comparable NIR-emitting phosphorescence (τ = 1.25 μs and ΦPL = 0.17) is also observed.
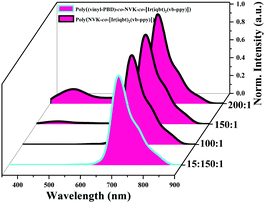 |
| Fig. 5 Normalized emission spectra of the Ir3+-polymers Poly(NVK-co-[Ir(iqbt)2(vb-ppy)]) (100 : 1, 150 : 1 or 200 : 1) and Poly((vinyl-PBD)-co-NVK-co-[Ir(iqbt)2(vb-ppy)]) (15 : 150 : 1) in solid-state at RT. | |
Device performance of NIR-PLEDs-I–III based on the complex monomer [Ir(iqbt)2(vb-ppy)] and its Ir3+-polymers
Due to the suitability of PVK-PBD (65
:
30; wt%) with good hole/electron transport as the co-host,28 it is of interest to use the efficient NIR-emitting complex monomer [Ir(iqbt)2(vb-ppy)] as the dopant (5 wt%) for the prototype NIR-PLED-I with the configuration shown in Fig. 6(a). Attributed to the fact that the experimental (Fig. S8, ESI†) HOMO (−5.17 eV) and LUMO (−3.03 eV) levels of the complex monomer [Ir(iqbt)2(vb-ppy)] aligned well within the band gap (−6.2 to −5.5 eV of HOMO and −2.6 to −2.0 eV of LUMO) of PVK-PBD, the injected electrons and holes through the PVK-PBD matrix are first trapped, and then direct charge trapping29 should occur within the NIR-emitting Ir(III)-complexes. As expected, as shown in Fig. 6(d), the electroluminescence spectra of the NIR-PLED-I are voltage-independent while just Ir(III)-complex-related NIR (λem = 696 and 756 (sh) nm; ca. 70% of the λem ≥ 700 nm proportion) emissions well resembled that (also Fig. 2) of the complex monomer [Ir(iqbt)2(vb-ppy)] in solution. The absence of the PVK-PBD residual light indicates that the effective Förster energy transfer22 also takes place within the doping EML upon electrical driving. For the NIR-PLED-I, upon the turn-on voltage (Von, defined as the voltage of the output irradiance (R) = 5.0 W sr−1 cm−2) of 9.0 V, as shown in Fig. 6(e), both the R and the current density (J) monotonically increase with the increase of the applied bias voltage (V), exhibiting an Rmax of 3772.1 W sr−1 cm−2 with a Jmax of 452.8 mA cm−2 at 21.0 V. Meanwhile, the NIR-PLED-I exhibits the R-regulated waving for the ηEQE (Fig. 6(f)), where the ηmaxEQE of 4.1% with the R = 65.5 W sr−1 cm−2 at 12.0 V and about 30% efficiency-roll-off in the higher radiance range of R = 65.5–3772.1 W sr−1 cm−2 are observed. It is worthy of note that due to the contribution from more excitons confined within the broadened recombination zone supplemented with the facilitated electron-transport TmPyPB,30 the overall device performance of the NIR-PLED-I is at the top-level (also Fig. 1) and comparable to the best one7c among the previously reported NIR-PLEDs.
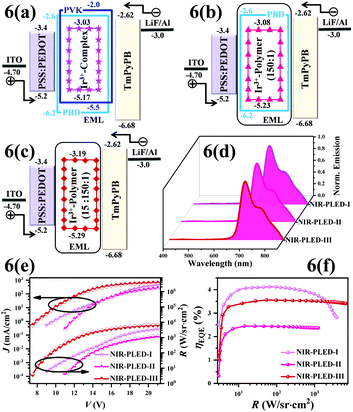 |
| Fig. 6 Device structures and energy level diagrams for the doping-type NIR-PLED-I (a) and the grafting-type NIR-PLEDs-II–III (b and c); normalized EL spectra (d); R–J–V (e) and ηEQE–R curves (f). | |
Considering the almost identical Ir3+-complex-grafted content between Poly(NVK-co-[Ir(iqbt)2(vb-ppy)]) (150
:
1) and Poly((vinyl-PBD)-co-NVK-co-[Ir(iqbt)2(vb-ppy)]) (15
:
150
:
1) comparable to that of the doping system (PVK
:
PBD
:
[Ir(iqbt)2(vb-ppy)]; 65
:
30
:
5, wt%) for the NIR-PLED-I, the bipolar Ir3+-polymers of Poly(NVK-co-[Ir(iqbt)2(vb-ppy)]) (150
:
1) and Poly((vinyl-PBD)-co-NVK-co-[Ir(iqbt)2(vb-ppy)]) (15
:
150
:
1) further doped with PBD and grafted with vinyl-PBD were used as the EML for the grafting NIR-PLEDs-II–III (Fig. 6(b and c)), respectively. Through the further grafting of the vinyl-PBD for the Poly((vinyl-PBD)-co-NVK-co-[Ir(iqbt)2(vb-ppy)]) (15
:
150
:
1), the electron-transport promotion is reflected from its experimentally (Fig. S9, ESI†) stabilized LUMO level (−3.19 eV) in comparison to that (−3.08 eV) of the Poly(NVK-co-[Ir(iqbt)2(vb-ppy)]) (150
:
1). Excitingly, for both the NIR-PLED-II with the doping of PBD into Poly(NVK-co-[Ir(iqbt)2(vb-ppy)]) (150
:
1) and the NIR-PLED-III based on Poly((vinyl-PBD)-co-NVK-co-[Ir(iqbt)2(vb-ppy)]) (15
:
150
:
1), Ir(III)-complex-exclusive NIR-emissive spectra similar to those of the NIR-PLED-I or their photo-luminescence results (also Fig. 5) in solid-states are observed. As compared with the NIR-PLED-I, due to the deeper LUMO gap between PBD and Poly(NVK-co-[Ir(iqbt)2(vb-ppy)]) (150
:
1), the Von of the NIR-PLED-II is up to 11.0 V. Moreover, the decreased ηmaxEQE of 2.5% and the Rmax of 1239.2 W sr−1 cm−2 show a good trade off with the significantly alleviated (ca. 3%) efficiency roll-off within the 12.0–21.0 range, which should be attributed to the lower carrier-trapping probability with the better carrier-balance within Poly(NVK-co-[Ir(iqbt)2(vb-ppy)]) (150
:
1). By contrast, using Poly((vinyl-PBD)-co-NVK-co-[Ir(iqbt)2(vb-ppy)]) (15
:
150
:
1) as the bipolar EML for the NIR-PLED-III, Förster energy transfer22 and carrier-trapping29 mechanisms also concurrently proceed within the TmPyPB-assisted recombination zone.30 Interestingly, for the NIR-PLED-III, besides the low Von at 7.5 V and the ηmaxEQE up to 3.6% at 9.0 V, the high RMax of 6559.3 W sr−1 cm−2 at 21.0 V is at the cost of the highest Jmax of 647.5 mA cm−2. Nonetheless, the superior device performance of the NIR-PLED-III is represented by the ηmaxEQE of 3.6% (9.0 V) and the weak (ca. 4%) efficiency roll-off with a preserved ηEQE of 3.4% at 21.0 V, which means that the high-efficiency of the NIR-PLED-I and the negligible efficiency roll-off of the NIR-PLED-II are well realized for the NIR-PLED-III. Importantly, this result makes bipolar Ir(III)-complex-grafted polymers a conceptual strategy to achieve high-performance NIR-PLEDs.
4. Conclusions
In summary, through the copolymerization of NVK, the vinyl-functionalized NIR-emitting monomer [Ir(iqbt)2(vb-ppy)] and/or the electron-transporting monomer vinyl-PBD, two series of Ir(III)-complex-grafted polymers Poly(NVK-co-[Ir(iqbt)2(vb-ppy)]) (100
:
1, 150
:
1 or 200
:
1) and Poly((vinyl-PBD)-co-NVK-co-[Ir(iqbt)2(vb-ppy)]) (15
:
150
:
1) are obtained, respectively. Moreover, using the doping system of PVK:PBD:[Ir(iqbt)2(vb-ppy)] or Poly(NVK-co-[Ir(iqbt)2(vb-ppy)]) (150
:
1) doped with PBD and the grafting system of the bipolar Poly((vinyl-PBD)-co-NVK-co-[Ir(iqbt)2(vb-ppy)]) (15
:
150
:
1) as the EML, reliable NIR-PLEDs-I–III are realized, respectively. Excitingly, for the NIR-PLED-III based on the bipolar Poly((vinyl-PBD)-co-NVK-co-[Ir(iqbt)2(vb-ppy)]) (15
:
150
:
1), the superior device performance (the ηmaxEQE of 3.6% and the negligible (<5%) efficiency roll-off) makes bipolar Ir(III)-complex-grafted polymers a new platform to achieve high-performance NIR-PLEDs.
Conflicts of interest
There are no conflicts to declare.
Acknowledgements
This work was funded by the National Natural Science Foundation (21373160, 21173165), the State Key Laboratory of Structure Chemistry (20190026), the Guangdong Basic and Applied Basic Research Foundation (2019A1515110527), the Wisteria Scientific Research Cooperation Special Project of Northwest University, the Hong Kong Research Grants Council (PolyU153058/19P), the Hong Kong Polytechnic University (1-ZE1C, YW4T) and the Endowed Professorship in Energy from Ms Clarea Au (847S) in China.
Notes and references
-
(a) L. T. Dou, Y. S. Liu, Z. R. Hong, G. Li and Y. Yang, Chem. Rev., 2015, 115, 12633–12665 CrossRef CAS;
(b) A. Rogalski and K. Chrzanowski, Opto-Electron. Rev., 2002, 10, 111–136 CAS.
- J. Clark and G. Lanzani, Nat. Photonics, 2010, 4, 438–446 CrossRef CAS.
- F. Ding, Y. Fan, Y. Sun and F. Zhang, Adv. Healthcare Mater., 2019, 8, 1900260 CrossRef.
-
(a) A. Zampetti, A. Minotto and F. Cacialli, Adv. Funct. Mater., 2019, 29, 1807623 CrossRef;
(b) M. Ibahim-Ouali and F. Dumur, Molecules, 2019, 24, 1412 CrossRef;
(c) C.-L. Ho, H. Li and W.-Y. Wong, J. Organomet. Chem., 2014, 751, 261–285 CrossRef CAS;
(d) H. F. Xiang, J. H. Cheng, X. F. Ma, X. G. Zhou and J. J. Chruma, Chem. Soc. Rev., 2013, 42, 6128–6185 RSC.
- J. H. Kim, J. H. Yun and J. Y. Lee, Adv. Opt. Mater., 2018, 6, 1800255 CrossRef.
-
(a) Y. X. Zhang, Q. Li, M. H. Cai, J. Xue and J. Qiao, J. Mater. Chem. C, 2020, 8, 8484–8492 RSC;
(b) J. Xue, L. J. Xin, J. Y. Hou, L. Duan, R. J. Wang, Y. Wei and J. Qiao, Chem. Mater., 2017, 29, 4775–4782 CrossRef CAS;
(c) I. Shigeru, Y. Shigeyuki, M. Takeshi, N. Hiroyuki, F. Hideki, K. Shiro and S. Yoshiaki, Inorg. Chem. Commun., 2013, 38, 14–19 CrossRef.
-
(a) Z. Chen, H. Y. Zhang, D. W. Wen, W. H. Wu, Q. G. Zeng, S. M. Chen and W.-Y. Wong, Chem. Sci., 2020, 11, 2342–2349 RSC;
(b) C. F. You, D. H. Liu, M. B. Zhu, J. T. Yu, B. Zhang, Y. Liu, Y. F. Wang and W. G. Zhu, J. Mater. Chem. C, 2020, 8, 7079–7088 RSC;
(c) C. F. You, D. H. Liu, F. Y. Meng, Y. F. Wang, J. T. Yu, S. Wang, S. J. Su and W. G. Zhu, J. Mater. Chem. C, 2019, 7, 10961–10971 RSC;
(d) J. Zhou, G. R. Fu, Y. N. He, L. N. Ma, W. T. Li, W. X. Feng and X. Q. Lü, J. Lumin., 2019, 209, 427–434 CrossRef CAS;
(e) H. U. Kim, S. Sohn, W. Choi, M. Kim, S. U. Ryu, T. Park, S. Jung and K. S. Bejoymohandas, J. Mater. Chem. C, 2018, 6, 10640–10658 RSC;
(f) Y. Liu, Z. R. Han, F. Y. Meng, P. Wang, L. Yang, Y. F. Wang, Y. Pei and S. J. Su, Chem. Phys. Lett., 2018, 699, 99–106 CrossRef CAS;
(g) S. Kesarkar, W. Mróz, M. Penconi, M. Pasini, S. Destri, M. Cazzaniga, D. Ceresoli, P. R. Mussini, C. Baldoli, U. Giovanella and A. Bossi, Angew. Chem., Int. Ed., 2016, 55, 2714–2718 CrossRef CAS;
(h) X. S. Cao, J. S. Miao, M. R. Zhu, C. Zhong, C. L. Yang, H. B. Wu, J. G. Qin and Y. Cao, Chem. Mater., 2015, 27, 96–104 CrossRef CAS;
(i) R. Tao, J. Qiao, G. L. Zhang, L. Duan, C. Chen, L. D. Wang and Y. Qiu, J. Mater. Chem. C, 2013, 1, 6446–6454 RSC;
(j) J. Qiao, L. Duan, L. T. Tang, L. He, L. D. Wang and Y. Qiu, J. Mater. Chem., 2009, 19, 6573–6580 RSC;
(k) E. L. Williams, J. Li and G. E. Jabbour, Appl. Phys. Lett., 2006, 89, 083506 CrossRef.
-
(a) H. U. Kim, H. J. Jang, W. Choi, S. Park, T. Park, J. Y. Lee and K. S. Bejoymohandas, J. Mater. Chem. C, 2020, 8, 4789–4800 RSC;
(b) Y. N. He, G. R. Fu, W. T. Li, B. W. Wang, T. Z. Miao, M. F. Tan, W. X. Feng and X. Q. Lü, J. Lumin., 2020, 218, 116847 CrossRef CAS;
(c) J. T. Yu, C. Xu, F. Y. Meng, H. Tan, M. Q. Liu and W. G. Zhu, Dyes Pigm., 2019, 166, 307–313 CrossRef CAS;
(d) Z. R. Hao, M. Li, Y. J. Liu, Y. F. Wang, G. H. Xie and Y. Liu, Dyes Pigm., 2018, 149, 315–322 CrossRef CAS;
(e) G. R. Fu, H. Zheng, Y. N. He, W. T. Li, X. Q. Lü and H. S. He, J. Mater. Chem. C, 2018, 6, 10589–10596 RSC.
-
(a) L. J. Xin, J. Xue, G. T. Lei and J. Qiao, RSC Adv., 2015, 5, 42354–42361 RSC;
(b) R. Tao, J. Qiao, G. L. Zhang, L. Duan, L. D. Wang and Y. Qiu, J. Phys. Chem. C, 2012, 116, 11658–11664 CrossRef CAS.
- J. V. Caspar and T. J. Meyer, J. Phys. Chem., 1983, 87, 952–957 CrossRef CAS.
- Y. M. Zhang, Y. F. Wang, J. Song, J. L. Qu, B. H. Li, W. G. Zhu and W.-Y. Wong, Adv. Opt. Mater., 2018, 6, 1800466 CrossRef.
- F. Xu, H. U. Kim, J. Kim, B. J. Jung, A. C. Grimsdale and D. Hwang, Prog. Polym. Sci., 2015, 47, 92–121 CrossRef CAS.
-
(a) Q. Zhao, S.-J. Liu and W. Huang, Macromol. Rapid Commun., 2010, 31, 794–807 CrossRef CAS;
(b) V. Marin, E. Holder, R. Hoogenboom and U. S. Schubert, Chem. Soc. Rev., 2007, 36, 618–635 RSC.
-
(a) C.-L. Ho and W.-Y. Wong, Top. Curr. Chem., 2016, 374, 1–23 CrossRef CAS;
(b) L. Ying, C.-L. Hong, H. B. Wu, Y. Cao and W.-Y. Wong, Adv. Mater., 2014, 26, 2459–2473 CrossRef CAS.
- M. Nonoyama, Bull. Chem. Soc. Jpn., 1979, 52, 3749–3750 CrossRef CAS.
- Z. Zhang, Y. L. He, L. Liu, X. Q. Lü, X. J. Zhu, W.-K. Wong, M. Pan and C. Y. Su, Chem. Commun., 2016, 52, 3713–3716 RSC.
- J. K. Augustine, V. Vairperrumal, S. Narasimhan, P. Alagarsamy and A. Radhakrishnan, Tetrahedron, 2009, 65, 9989–9996 CrossRef CAS.
- L. Liu, M. Y. Pang, H. T. Chen, G. R. Fu, B. N. Li, X. Q. Lü and L. Wang, J. Mater. Chem. C, 2017, 5, 9021–9027 RSC.
- P.-N. Lai, C. H. Brysacz, M. K. Alam, N. A. Ayoub, T. G. Gray, J. Bai and T. S. Teets, J. Am. Chem. Soc., 2018, 140, 10198–10207 CrossRef CAS.
- P.-N. Lai and T. S. Teets, Chem. – Eur. J., 2019, 25, 6026–6037 CrossRef CAS.
- C. Tang, X. D. Liu, F. Liu, X. L. Wang, H. Xu and W. Huang, Macromol. Chem. Phys., 2013, 241, 314–342 CrossRef.
- D. R. Martir and E. Zysman-Colman, Coord. Chem. Rev., 2018, 364, 86–117 CrossRef.
- G. R. Fu, Y. N. He, W. T. Li, T. Z. Miao, X. Q. Lü, H. S. He, L. Liu and W.-Y. Wong, Chem. Sci., 2020, 11, 2640–2646 RSC.
- G. R. Fu, L. Liu, W. T. Li, Y. N. He, T. Z. Miao, X. Q. Lü and H. S. He, Adv. Opt. Mater., 2019, 7, 1900776 CrossRef CAS.
- A. Kirch, M. Gmelch and S. Reineke, J. Phys. Chem. Lett., 2019, 10, 310–315 CrossRef CAS.
- Z.-H. Wang, H. Lee and H.-N. Cui, J. Appl. Phys., 2012, 111, 023512 CrossRef.
- M. Mauro and C. Cebrian, Isr. J. Chem., 2018, 58, 901–914 CrossRef CAS.
-
(a) Z. R. Hao, H. G. Jiang, Y. Liu, Y. M. Zhang, J. T. Yu, Y. F. Wang, H. Tan, S. J. Su and W. G. Zhu, Tetrahedron, 2016, 72, 8542–8549 CrossRef CAS;
(b) V. Jankus, K. Abdullah, G. C. Griffiths, C. Gareth, H. Al-Attar, Y. H. Zheng, M. R. Bryce and A. P. Monkman, Org. Electron., 2015, 20, 97–102 CrossRef CAS;
(c) H. Al-Attar, G. C. Griffiths, T. N. Tom, M. Tavasli, M. A. Fox, M. R. Bryce and A. P. Monkman, Adv. Funct. Mater., 2011, 21, 2376–2382 CrossRef CAS.
-
(a) T. Y. Li, J. Wu, Z. G. Wu, Y. X. Zheng, J. L. Zuo and Y. Pan, Coord. Chem. Rev., 2018, 374, 55–92 CrossRef CAS;
(b) X. L. Yang, G. J. Zhou and W.-Y. Wong, Chem. Soc. Rev., 2015, 44, 8484–8575 RSC.
-
(a) T. Earmme and S. A. Henehke, J. Mater. Chem. C, 2012, 22, 4660–4668 RSC;
(b) T. L. Te, S. Y. Shao, J. S. Chem, L. X. Wang and D. G. Ma, ACS Appl. Mater. Interfaces, 2011, 3, 410–416 CrossRef.
Footnotes |
† Electronic supplementary information (ESI) available: Starting materials and characterization; NMR, UV, and PL spectra. See DOI: 10.1039/d0tc04377j |
‡ These authors contributed equally and should be considered co-first authors. |
|
This journal is © The Royal Society of Chemistry 2021 |
Click here to see how this site uses Cookies. View our privacy policy here.