DOI:
10.1039/D2AN00218C
(Paper)
Analyst, 2022,
147, 2819-2827
A portable blood lactate sensor with a non-immobilized enzyme for early sepsis diagnosis†
Received
4th February 2022
, Accepted 18th April 2022
First published on 26th May 2022
Abstract
Early determination of blood lactate levels may accelerate the detection of sepsis, one of the most time-sensitive illnesses. We developed and validated a portable blood lactate detection kit for clinical screening that can measure early bedside lactate levels in intensive care unit (ICU) patients suspected of having sepsis. A TiO2 sol–G nanocomposite was prepared and coated on a screen-printed carbon electrode (SPCE) integrated with non-immobilized lactate oxidase (LOx) to produce a novel lactate biosensor with high sensitivity and high storage stability for human blood lactate measurement. The detection kit was based on an electrochemical technique and showed a wide linear range of 1–20 mM (R2 = 0.9937) with a low detection limit of 0.2 mM for lactate detection. This allowed for differentiating patient groups who may have sepsis using a cut-off level of 4 mM. The device was successfully implemented for blood lactate determination in critical patients, showing an accuracy range from 75% to 112%. This device provided high-precision and rapid quantitative information validated using a blood gas analyzer. Our detection kit might help to reduce the morbidity and mortality rates in severe sepsis and septic shock patients in community hospitals.
Introduction
Blood lactate in circulation can be a useful biomarker to identify patients at increased risk of mortality from sepsis. It can be also an indicator of mortality and critical care admission.1,2 Serum lactate is produced from aerobic metabolism and is related to tissue hypoxia, aerobic glycolysis, and liver or renal failure that results in sepsis severity, sepsis shock, or mortality. Generally, the level of serum lactate in healthy people is less than 2 mM, but it can increase to 25 mM during intense exercise.3 It can also reach 5 or above in critically ill patients which is called hyperlactatemia or lactic acidosis.4,5 The lactate cut-off level was first suggested in 1964 when a lactate level of more than 4 mM was found to be associated with poor outcomes in patients with undifferentiated shock.4 An initial level of lactate monitored within an hour with a 2–4 mM serum lactate level is considered to be at low risk for mortality, with the risk increasing as the lactate level rises to greater than 4 mM.7,8 An increase in blood lactate levels is related to mortality among critically ill patients.2,5 Early recognition of elevated lactate levels may reduce the mortality rate in patients with severe sepsis.
Several analytical approaches including blood gas analyzers, portable point of c (POC) devices, and lactate assay kits have been used for blood lactate measurements.6–8 Although these methods are found in various referent laboratories or hospitals and are commercially available in worldwide markets, they involve transportation of blood samples to the laboratory which delays the reporting of results to clinicians.9 The point-of-care blood gas analyzer devices for blood lactate measurements in Thailand entirely are imported from abroad, which leads to high cost and time-consumption, require laboratory equipment and trained personnel and have limited accessibility for remote areas, especially for community hospitals. Some POC technologies are already available for lactate sensing such as the bench top blood gas analyzer. These devices are not portable and their availability is limited to specialist units, such as intensive care units.6 The advanced medical devices are also unavailable for patients in remote areas due to the high cost of medical equipment imported from overseas and the challenges of instrument transportation.
Many sensing platforms developed for blood lactate detection are based on amperometric biosensors combined with immobilized enzymes including lactate oxidase.10,11 These devices have advantages such as high specificity in detecting lactate; however, the use of immobilized enzyme systems for electrochemical biosensors suffers from the short-time storage stability of the biosensors.12–14 This may be ascribed to the denaturation of immobilized enzymes during storage, low stability characteristics, and mass transfer limitations of the enzymes. To overcome these limitations, non-immobilized enzyme systems could be an alternative for the development of a blood lactate detection kit. Even though these lactate biosensors systems need to split the step of enzyme addition into samples,15 it does not alter enzyme shapes and can function as a result of the constant enzyme activities.16 There may also not be diffusion problems for the substrate to access the enzyme.17
The development of electrochemical sensing platforms employs a working electrode as the most important part. To increase the sensitivity of electrochemical biosensors, it is necessary to use nanomaterials for working electrode modifications. Nanocomposite materials based on graphene (G) and titanium dioxide sol (TiO2 sol) have become promising nanomaterials for electrode surface modification. Since G possesses excellent electrical conductivity and a high specific surface area, it potentially enhances electrocatalytic activity of sensors and promotes the electron transfer of biomolecules on the modified electrode surfaces.18 In addition, a two-dimensional (2D) crystal structure of G makes it suitable as a support material for the anchoring of metal oxide (i.e. TiO2 sol), which offers versatile properties for sensor performance.19 It is well known that TiO2 sol is an effective interface for biomolecule immobilization due to its large specific surface area, strong adsorption ability, and good biocompatibility.20 Our group previously reported on the use of TiO2 sol–G nanocomposite modified electrodes along with immobilized LOx for a lactate sensor.21 It was found that the TiO2 sol–G nanocomposite played a role in enhanced surface area and improved electro-conductivity giving rise to a tremendous increase in the current response (∼47 times) for lactate compared to an unmodified electrode.21 Nonetheless, the stability of this sensor was still limited owing to the immobilized lactate oxidase enzyme on the electrode surface.
In this study, we developed a lactate biosensor based on a TiO2 sol–G nanocomposite modified screen-printed electrode (SPCE) integrated with a non-immobilized enzyme system for use as a portable blood lactate detection kit. The electrochemical performance sensitivity and long-term stability of the sensing device were studied using amperometry. The proposed detection kit was employed for the measurement of patient blood lactate in ICU and validated with a blood gas analyzer to verify the practical efficacy for real clinical application.
Experimental
Ethics
All experiments were performed in accordance with the guidelines for human research protection according to the Declaration of Helsinki and Principles of Good Clinical Practice, and approved by the ethics committee at the Institutional Review Board of the Faculty of Medicine, Chulalongkorn University, Bangkok Thailand (IRB No. 498/63). Informed consent was obtained from human participants of this study.
Chemicals and materials
All the chemicals and materials are illustrated in the ESI, Text S1.†
Instruments
All the instruments are illustrated in the ESI, Text S2.†
Synthesis of the TiO2 sol–G nanocomposite
The TiO2 sol–G nanocomposite was prepared by hydrolysis and condensation reactions of titanium alkoxides using a modified method from our previous report.21 The prepared nanocomposite was characterized by transmission electron microscopy (TEM, Japan Electron Optics Laboratory, Japan).
Fabrication of a modified electrode and electrochemical analysis
The fabrication of the modified electrode and electrochemical analysis is illustrated in the ESI, Text S3 and S4.†
Sample collection
All patients admitted to all sections of intensive care units (ICUs) at King Chulalongkorn Memorial Hospital (KCMH) between November 2020 and February 2021 (without the duration of treatment) were initially screened for their blood lactate levels using a blood gas analyzer (Stat Profile Prime Plus®, NOVA, USA) (gold standard). All patients were adults aged 18 years and older. 2 mL of whole blood from critically ill patients was routinely collected into a lithium heparin tube (anti-coagulant) via arterial and central venous access devices by peripheral venipunctures. Because the samples were collected without the duration of treatment, some patients might recover from treatment, resulting in a lower lactate level than the cut-off.
Patient blood lactate measurements
After the optimization of the experimental conditions, all data were employed to develop a customized reader for the portable blood lactate detection kit following the configuration shown in Scheme 1. After the preparation of the modified electrode, aliquots of 50 μL from each patient's blood sample were added to individual microcentrifuge tubes added with 1 μL of lactate oxidase (50 U mL−1). The resulting mixture was incubated at room temperature for 5 min and examined for blood lactate by dropping the mixture onto the modified electrode inserted into the customized portable reader, and then counting down 170 s for the estimated lactate levels to be shown on the screen. All samples were analyzed with the portable blood lactate detection kit and validated with the previous blood gas analyzer results.
 |
| Scheme 1 Schematic of the proposed procedure for a portable blood lactate sensor with non-immobilized enzyme for the determination of lactate levels in human whole blood. | |
Statistical analysis
We presented our descriptive statistics as mean ± SD, relative standard deviation (% RSD) and accuracy. The strength of association between the two techniques was measured by the Pearson correlation coefficient (r). Analysis of the agreement was performed by using the precision-bias matrix as described by Bland Altman plot.22 Statistical analysis was carried out using a SPSS version 22.0 software.
Results and discussion
Optimization of TiO2 sol–G nanocomposite synthesis
Effect of TiO2 sol and G concentration and TEM characterization.
To understand the influence of TiO2 sol and G concentration on the current response for the measurement of lactate, a series of working electrodes was prepared by varying concentrations of TiO2 sol (2–40 mg mL−1, Fig. S1a†) with a fixed concentration of G at 1.5 mg mL−1, and varying concentrations of G (0–4.5 mg mL−1, Fig. S1b†) with a fixed concentration of TiO2 sol at 16 mg mL−1. These modified electrodes were examined by sensing 5 mM of lactate in PB (pH 7.4) using amperometry. The current response substantially increased on increasing the TiO2 sol concentration up to 16 mg mL−1, indicating the enhanced TiO2 sol nanoparticle accumulation and enlarged surface area on the electrode resulting in a corresponding increase in the current response to lactate. However, the current response decreased at a TiO2 sol concentration above 16 mg mL−1, which may have arisen due to the agglomeration of TiO2 sol on the electrode surface. The 16 mg mL−1 TiO2 sol was selected for all subsequent experiments. In a similar study, a measured optimum G concentration of 2.5 mg mL−1 was chosen for use in all subsequent experiments (Fig. S1b†). The decreased current response observed for the G concentration in excess of 2.5 mg mL−1 may have also been caused by the agglomeration of multiple layers of G on the electrode surface resulting in decreased electroconductivity for electrochemical detection of lactate.
The morphologies of TiO2 sol and the TiO2 sol–G nanocomposite were examined by TEM. The TEM of TiO2 sol is shown in Fig. 1a. The TiO2 sol particles showed a uniform spherical shape with an average size of 61.4 ± 9.8 nm and good dispersion without agglomeration. The as-prepared TiO2 sol was then incorporated with G using a sol–gel method. It can be readily observed that there is an intercalation between nanoparticles of TiO2 sol and GO sheets (Fig. 1b), consistent with the previous study,23 suggesting that TiO2 sol acts like a stabilizing agent that improves the dispersion of GO.
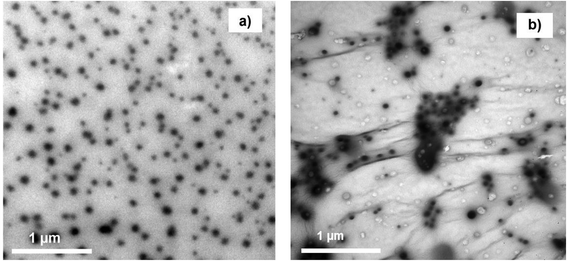 |
| Fig. 1 Transmission electron micrographs of TiO2 sol (a) and the TiO2 sol–G nanocomposite and (b) at 15 000× magnification. | |
Optimization of experimental parameters for lactate detection
Effect of enzyme loading volume and reaction time.
For lactate detection, the effects of enzyme loading volume and reaction time on the ensuing current response were investigated using amperometry based on the following reactions:
Lactate + O2 → pyruvate + H2O2 |
The current response of 5 mM lactate in 0.1 M PB (pH 7.4) measured on the TiO2 sol–G/SPCE with different volumes of 50 U mL−1 LOx with a reaction time of 5 min is shown in Fig. S2a.† The current response toward lactate peaked for an enzyme loading volume of 1 μL and tended to be constant for greater volumes of LOx, which may be attributed to all of the substrate being bound resulting in the slowing down of the reaction. The 1 μL of LOx volume was thus selected for all subsequent experiments. Based on a similar study, the evaluated optimum time of enzymatic reaction of 5 min was chosen for use in all follow-up experiments (Fig. S2b†). The decreased current response seen after 5 min may have resulted from some enzymes being noticeably unstable and losing a significant amount of activity over the period of reaction.
Analytical performance of the optimized TiO2 sol–G/SPCE
The TiO2 sol–G/SPCE biosensor exhibited a high sensitivity for lactate in a wide linear response range, obtained by a strict linear calibration plot from 0.001 to 0.02 M lactate in 0.1 M PB (pH 7.4) at an applied potential of +0.5 V (vs. Ag|AgCl) using amperometry (Fig. S3†). Detection limits of around 0.2 mM could be estimated using 3.3 × (SD/slope). A high sensitivity of 150.17 μA cm−2 mM−1 was observed. The analytical performance of the optimized TiO2 sol–G/SPCE was compared to the LOx modified TiO2 sol–G/SPCE at different lactate concentrations ranging from 0.00–0.003 M (Fig. S4†). The sensitivity of the immobilized LOx electrode (40.39 μA cm−2 mM−1) and the non-immobilized LOx electrode (26.52 μA cm−2 mM−1) differed insignificantly. The current response of the LOx/TiO2 sol–G/SPCE was found to be lower than that of TiO2 sol–G/SPCE. This result indicated that the non-immobilized approach could be used for lactate determination using the simpler electrode preparation without the enzyme immobilization method, reducing any immobilized enzyme stability concerns.
For practical utility in whole blood lactate detection, an additional standard method was used to study the performance of the biosensor. The TiO2 sol–G/SPCE biosensor was tested in the spiked lactate concentrations in human whole blood ranging from 0.001–0.020 mM at an applied potential of +0.5 V (vs. Ag|AgCl). A calibration plot for blood lactate was constructed using amperometric current responses measured at a steady state current in 170 s. As shown in Fig. 2a, the current response increased as a function of the lactate concentration, and the proposed biosensor showed a linear trend over a range of 0.001–0.020 M with a correlation coefficient of 0.9948 and a sensitivity of 191.07 μA cm−2 mM−1 (Fig. 2b). The detection limit of the biosensor was estimated to be 0.5 mM at a signal-to-noise ratio of 3. Compared with the calibration plot of the lactate detection in PB (Fig. S3†), the results show that the amperometric response and the slope of blood lactate detection are significantly greater than lactate detection in PB, which may be attributed to interfering compounds in whole blood that had a great effect on the biosensor signal and changed the analytical sensitivity. Therefore, the additional standard method needs to be used to study the sensitivity of the biosensor for sensing lactate in real samples and the obtained calibration plot was applied to further experiments in lactate detection in whole blood.
 |
| Fig. 2 Amperometric responses of the TiO2 sol–G nanocomposite/SPCEs (n = 5) for various spiked lactate concentrations (0.001–0.020 M) in whole blood measured at +0.5 V (a). Calibration plot for blood lactate detection constructed from the signal measurements presented in a (b). | |
Reproducibility and stability
TiO2 sol–G nanocomposite and TiO2 sol–G nanocomposite/SPCE were produced under optimized conditions. The production reproducibility of the TiO2 sol–G nanocomposite was investigated by measuring the amperometric current response of 5 mM lactate in 0.1 M PB (pH 7.4) for five different production batches. The relative standard deviation (RSD) was estimated to be 3.1% (Fig. 3a), indicating an acceptable reproducibility in the nanocomposite production. The reproducibility in the TiO2 sol–G/SPCE biosensor construction was evaluated by preparing five biosensors and determining the current response of 5 mM lactate in 0.1 M PB (pH 7.4) for each biosensor. Compared with the immobilized LOx electrode, the reproducibility of TiO2 sol–G/SPCE yielded an RSD of 2.6% (Fig. 3b), while the immobilized LOx electrode yielded an RSD of 5.1% (Fig. S5†). The data support the fact that the non-immobilized enzyme system minimized electrode-to-electrode variability, which may be ascribed to decreased unfavorable orientation and (partial) unfolding of enzymes, improving their activity in non-immobilization.24
 |
| Fig. 3 Reproducibility of five different nanocomposite production batches (a) and TiO2 sol–G nanocomposite modified electrodes (b). Long-term stability of the TiO2 sol–G nanocomposite (c) and the nanocomposite modified electrodes (d) recorded in 0.1 M PB (pH 7.4) containing 5 mM lactate at +0.5 V; each data point for a, c, and d has been obtained by measurements with 5 individual electrodes; error bars indicate the standard deviations. | |
The long-term storage stability of the TiO2 sol–G nanocomposite and the TiO2 sol–G modified electrode were investigated by evaluating their current responses to a 5 mM lactate solution in 0.1 M PB (pH 7.4). In this case, a percent relative response, which refers to the signal of the initial day retained after being stored, was used to monitor the remaining signal response of the nanocomposite and the modified electrode after the storage time to evaluate the shelf-life of the biosensor.25,26 The nanocomposite was stored in a brown glass bottle and the modified electrode was kept in a desiccator at room temperature when not in use. Under the storage conditions, the nanocomposite exhibited good stability. Insignificant changes were observed in the current responses after storing the nanocomposite for over 5 months (Fig. 3c). The storage stability of the TiO2 sol–G/SPCEs still retained more than 95% of their original responses to lactate after 59 days (Fig. 3d), which is more stable than the LOx modified TiO2 sol–G/SPCEs with a decreased relative response of 76% on day 20 (Fig. S6†). This is because the surface immobilization disrupts the enzyme structure leading to a loss of the catalytic efficiency and stability of the enzyme. This result confirms that the non-immobilized enzyme system can extend the shelf-life of the biosensor, allowing for high stability that makes it applicable for practical use.
A comparative study of different electrodes for blood lactate biosensors (Table 1) showed that the proposed system achieves comprehensive electrochemical properties with a good amperometric response and linearity in a wide range with limits of detection comparable to those founds in previous studies based on biosensors for blood lactate detection. Our biosensor also has longer storage stability than other biosensors. Its linearity exceeded 3 electrodes,27–29 and its limit of detection was comparable to one sensor30 and better than 2 sensors.28,29 Furthermore, the sensitivity obtained from this sensor (1.0–20.0 mM) was sufficient for distinguishing groups of critical patients that may progress to septic shock according to the lactate cut-off level of 4 mM.
Table 1 Comparison of different modified electrodes for electrochemical detection of blood lactate
Working electrode |
Enzyme |
Linear range |
Limit of detection |
Response time |
Stability |
Ref. |
LOx: lactate oxidase; ITO: indium tin oxide electrode; An-co-FAn: aniline-co-fluoroaniline; GCE: glassy carbon electrode; NADH: nicotinamide adenine dinucleotide; LDH: lactate dehydrogenase; CeO2: cerium oxide; MWCNT: multi-walled carbon nanotube; PCS; poly(carbamoyl) sulfonate; SPG: screen-printed graphite; PAA: polyacrylic acid; Si4N3: silicon nitride; ND: nanostructured; CPE: carbon paste electrode; NR: not report. |
Mucin/albumin hydrogel/Pt |
Immobilized LOx |
0.2–1.0 mM |
0.8 μM |
300 s |
30 days |
27
|
Poly(An-co-FAn)/ITO |
Immobilized LOx |
0.1–5.5 mM |
0.1 mM |
5 s |
<26 days |
30
|
Polycarbonate/Pt |
Immobilized LOx |
0.7–1.5 mM |
0.7 mM |
90 s |
27 days |
28
|
NADH/NanoCeO2/GCE |
Immobilized LDH |
0.2–2.0 mM |
50 μM |
4 s |
12 days |
14
|
MWCNT/NanoPt/GCE |
Immobilized LOx |
0.2–2.0 mM |
0.01 mM |
5 s |
28 days |
31
|
PCS hydrogel/SPG/Nafion/Pt |
Immobilized LOx |
0.0–0.5/0–1.0 mM |
0.5 mM |
42 s |
42 days |
29
|
MWCNT/GCE |
Immobilized LDH |
0.2–2.0 mM |
0.3 μM |
NR |
NR |
32
|
PAA/Si4N3 (ND) surface |
Immobilized LDH |
0.0–50.0 μM |
0.2 μM |
60 s |
NR |
33
|
NanoZnO–MWCNTs/GCE |
Immobilized LOx |
0.01–10 μM/10–200 μM |
4.0 mM |
NR |
NR |
34
|
Niobium oxide/CPE |
Immobilized LOx |
0.1–5.0 mM |
NR |
NR |
45 |
35
|
TiO2 sol–G/3D porous Ni foam |
Immobilized LOx |
50 μM–10 mM |
19 μM |
180 s |
8 days |
25
|
TiO2 sol–G/SPCE |
Non-immobilized LOx |
1.0–20.0 mM |
0.2 mM |
170 s |
>59 days |
Presented work |
Development of a portable kit for blood lactate sensing
Results from the studies listed in Table 1 provided a foundation for the development of a handheld blood lactate detection kit for clinical screening in ICU patients. As shown in Fig. 4, the proposed kit was initially developed by optimization of the experimental parameters, such as detection potential and a steady state analysis time, for lactate measurements using a Sensit Smart portable potentiostat. The best conditions for the preparation of TiO2 sol–G/SPCE are 16 mg mL−1 TiO2 sol and 2.5 mg mL−1 G. The optimal conditions for lactate detection are 1.0 μL of 50 U mL−1 Lox and 5 min of reaction time, including detection potential at +0.5 V (vs. Ag|AgCl) and a steady state current time analysis in 170 s for amperometric measurements. However, our results were obtained by calculating the current signal processing and displaying the result via a computer since it is too complex to perform in a practical implementation. Next, all optimized results were employed to fabricate a customized device by the Wuxi Admas Technology company in which the function of the device was based on amperometry and the calibration plot of spike blood lactate. This device could be used for specific blood lactate monitoring and could display the precise levels of lactate on the screen instead of through current signals. The detection kit was used with the ICU patients for blood lactate determination.
 |
| Fig. 4 Steps for developing a portable blood lactate electrochemical device. | |
Clinical trials and statistical analysis
General characteristics of ICU sepsis patients including age, sex and arterial lactate level were documented. Fifty patients were enrolled in the study. As shown in Table S1,† 22 patients (44%) were female and 28 patients (56%) were male. The average age was 60.2 ± 20.8 years (mean ± SD).
To verify the practical utility of the proposed blood lactate detection kit, the device was tested with whole blood samples (n = 50) obtained from patients without sepsis and ICU patients with sepsis (Fig. S7†). Sample analysis was carried out using the method described in the Experimental section. It was then analyzed on three independently prepared electrodes using the portable blood lactate detection kit. Table S2† shows the lactate measurement results from 5 different whole blood samples of healthy people with lactate levels in the range from 2.1 to 5.7 mM, corresponding well with a normal blood lactate level of 2.5–4.0 mM.11,36 Raised lactate levels commonly arise in a wide variety of conditions (e.g., intense exercises) depending on the activities throughout the day.37 In cases of sepsis, levels greater than 4 mM are considered as severe sepsis.16 Table S3† shows the lactate measurement results from 50 different whole blood samples. The results are in agreement with the measurements obtained by a blood gas analyzer used in the referent hospital laboratory.
Pearson coefficients were calculated for testing the correlation between the lactate concentrations measured using the blood gas analyzer (gold standard) and the proposed electrochemical biosensor as shown in Fig. 5a. There was a very strong, highly significant correlation between the two techniques (Pearson r = 0.978 (p < 0.001).
 |
| Fig. 5 Correlation analysis in clinical studies: comparison of lactate levels in whole blood (n = 50) by a blood gas analyzer (mmol L−1) and electrochemical assay (mmol L−1) with the Pearson r coefficient of 0.978 (p < 0.001) (a). Difference between blood gas analyzer and electrochemical assay (means SD, 0.27 ± 1.32 mmol L−1) (b). | |
Bland and Altman manner (1986) statistics exhibited an average bias for the electrochemical biosensor of 0.27, which is lower than the blood gas analyzer, with 95% probability limits of agreement ranging from −2.32 to 2.85. As shown in Fig. 5b, the solid line represents the bias and dashed lines represent the upper and lower 95% limit of agreement. The average correlation of each pair of blood gas analyzer and electrochemical biosensor values was plotted against their differences (mean ± SD, 0.27 ± 1.32 mmol L−1). No significant difference was found between two the techniques, electrochemistry and blood gas analyzer.
The study results have revealed that the electrochemical biosensor device provided accurate determination, rapid results, portability, and cost effectiveness. It may be a useful and simple strategy to identify patients at risk of severe sepsis by testing whole blood lactate at ICU admission. Further studies are needed to compare the use of the two techniques in a larger population.
Conclusions
TiO2 sol–G nanocomposite/SPCE was successfully synthesized and fabricated on a working electrode of a portable blood lactate kit for clinical testing. A wide linear response was observed for spiked lactate concentrations in whole blood that ranged over 1.0–20.0 mM, enabling the device to identify patients with sepsis using a value indicative of compromised sepsis of 4 mM lactate. This device was sensitive enough for lactate measurements at higher and lower than normal concentrations with significantly unchanged sensitivity over 59 days. For clinical trials, the lactate measurements using the portable blood lactate kit correlated well with results from a blood gas analyzer in ICU patients with sepsis and normal or slightly raised lactate levels. This device might reduce importing costs of portable blood lactate instruments in developing countries (e.g., Thailand). Further studies are required to corroborate these results with a larger population, especially patients with severe sepsis or septic shock, and to assess the effects of early detection of blood lactate levels on the prognosis, treatment, and mortality rate reduction of ICU patients.
Author contributions
All authors have accepted the responsibility for the entire content of this manuscript and approved its submission. Conceptualization: NS, NR, PR, SB, PK. Methodology: SB, PK, TL. Formal analysis and investigation: NS, NR, SB, PK. Writing: PK, SB. Original draft preparation: NS, NR, PR, SB, PK, TL. Writing – review and editing: PK, SB, NR, PR, NS. Funding acquisition: NS, NR. Supervision: NS, NR.
Conflicts of interest
There are no conflicts to declare.
Acknowledgements
This work was supported by the Grant for Invention Chulalongkorn University (Ratchadaphiseksomphot Endowment Fund). Siraprapa Boobphahom would like to thank Postdoctoral Fellowship, Ratchadapisek Somphot Fund, Chulalongkorn University. This research is supported by the Thailand Science Research and Innovation Fund (CU_FRB65_food (29)219_62_04), Chulalongkorn University.
References
- M. D. Howell,
et al., Occult hypoperfusion and mortality in patients with suspected infection, Intensive Care Med., 2007, 33(11), 1892–1899 CrossRef PubMed.
- N. I. Shapiro,
et al., Serum lactate as a predictor of mortality in emergency department patients with infection, Ann. Emerg. Med., 2005, 45(5), 524–528 CrossRef PubMed.
- M. Mascini,
et al., Lactate and pyruvate electrochemical biosensors for whole blood in extracorporeal experiments with an endocrine artificial pancreas, Clin. Chem., 1987, 33(4), 591–593 CrossRef CAS.
- G. Broder and M. H. Weil, Excess lactate: an index of reversibility of shock in human patients, Science, 1964, 143(3613), 1457–1459 CrossRef CAS PubMed.
- D. A. Del Portal,
et al., Emergency department lactate is associated with mortality in older adults admitted with and without infections, Acad. Emerg. Med., 2010, 17(3), 260–268 CrossRef PubMed.
- F. Ismail,
et al., The accuracy and timeliness of a Point Of Care lactate measurement in patients with Sepsis, Scand. J. Trauma Resusc. Emerg. Med., 2015, 23(1), 1–7 CrossRef PubMed.
- P. Murugesan,
et al., Kinin B1 receptor inhibition with BI113823 reduces inflammatory response, mitigates organ injury, and improves survival among rats with severe sepsis, J. Infect. Dis., 2016, 213(4), 532–540 CrossRef CAS PubMed.
- N. D. Indrasari, J. P. Wonohutomo and N. Sukartini, Comparison of point-of-care and central laboratory analyzers for blood gas and lactate measurements, J. Clin. Lab. Anal., 2019, 33(5), e22885 CrossRef PubMed.
- N.-E. Drenck, Point of care testing in Critical Care Medicine: the clinician's view, Clin. Chim. Acta, 2001, 307(1–2), 3–7 CrossRef CAS.
- P. J. Lamas-Ardisana,
et al., Disposable amperometric biosensor based on lactate oxidase immobilised on platinum nanoparticle-decorated carbon nanofiber and poly(diallyldimethylammonium chloride) films, Biosens. Bioelectron., 2014, 56, 345–351 CrossRef CAS PubMed.
- K. Dagar and C. Pundir, An improved amperometric L-lactate biosensor based on covalent immobilization of microbial lactate oxidase onto carboxylated multiwalled carbon nanotubes/copper nanoparticles/polyaniline modified pencil graphite electrode, Enzyme Microb. Technol., 2017, 96, 177–186 CrossRef CAS PubMed.
- G. Aydın,
et al., Amperometric enzyme electrode for L (+)-lactate determination using immobilized L (+)-lactate oxidase in poly(vinylferrocenium) film, Sens. Actuators, B, 2002, 87(1), 8–12 CrossRef.
- A. Parra,
et al., Design and characterization of a lactate biosensor based on immobilized lactate oxidase onto gold surfaces, Anal. Chim. Acta, 2006, 555(2), 308–315 CrossRef CAS.
- N. Nesakumar,
et al., Fabrication of lactate biosensor based on lactate dehydrogenase immobilized on cerium oxide nanoparticles, J. Colloid Interface Sci., 2013, 410, 158–164 CrossRef CAS PubMed.
- H. Patel and A. Kharat, Comparative study of free enzyme & immobilized enzyme on BSA digestion, Int. J. Pharma Sci. Res., 2012, 3(12), 5015–5023 Search PubMed.
- H. H. Nguyen and M. Kim, An overview of techniques in enzyme immobilization, Appl. Sci. Converg. Technol., 2017, 26(6), 157–163 CrossRef.
- M. Hartmann and X. Kostrov, Immobilization of enzymes on porous silicas – benefits and challenges, Chem. Soc. Rev., 2013, 42(15), 6277–6289 RSC.
- Y. Shao,
et al., Graphene based electrochemical sensors and biosensors: a review, Electroynalysis, 2010, 22(10), 1027–1036 CrossRef CAS.
- P. V. Kamat, Graphene-based nanoarchitectures. Anchoring semiconductor and metal nanoparticles on a two-dimensional carbon support, J. Phys. Chem. Lett., 2010, 1(2), 520–527 CrossRef CAS.
- U. Diebold, Structure and properties of TiO2 surfaces: a brief review, Appl. Phys. A, 2003, 76(5), 681–687 CrossRef CAS.
- S. Boobphahom,
et al., TiO2 sol/graphene modified 3D porous Ni foam: A novel platform for enzymatic electrochemical biosensor, J. Electroanal. Chem., 2019, 833, 133–142 CrossRef CAS.
- K. Dewitte,
et al., Application of the Bland–Altman plot for interpretation of method-comparison studies: a critical investigation of its practice, Clin. Chem., 2002, 48(5), 799–801 CrossRef CAS.
- N. Promphet, P. Rattanawaleedirojn and N. Rodthongkum, Electroless NiP-TiO2 sol-RGO: A smart coating for enhanced corrosion resistance and conductivity of steel, Surf. Coat. Technol., 2017, 325, 604–610 CrossRef CAS.
- J. E. Butler, Solid supports in enzyme-linked immunosorbent assay and other solid-phase immunoassays, Methods, 2000, 22(1), 4–23 CrossRef CAS PubMed.
- A. C. Celik,
et al., Amperometric lactate biosensor based on carbon paste electrode modified with benzo[c]cinnoline and multiwalled carbon nanotubes, Electroanalysis, 2015, 27(12), 2820–2828 CrossRef CAS.
- T. Shimomura,
et al., Amperometric l-lactate biosensor based on screen-printed carbon electrode containing cobalt phthalocyanine, coated with lactate oxidase-mesoporous silica conjugate layer, Anal. Chim. Acta, 2012, 714, 114–120 CrossRef CAS PubMed.
- M. R. Romero,
et al., Amperometric biosensor for direct blood lactate detection, Anal. Chem., 2010, 82(13), 5568–5572 CrossRef CAS PubMed.
- J. Chen,
et al., A novel composite of molecularly imprinted polymer-coated PdNPs for electrochemical sensing norepinephrine, Biosens. Bioelectron., 2015, 65, 366–374 CrossRef CAS PubMed.
- N. Patel,
et al., Fabrication and characterization of disposable type lactate oxidase sensors for dairy products and clinical analysis, Sens. Actuators, B, 2000, 67(1–2), 134–141 CrossRef CAS.
- S. Suman,
et al., Development of a lactate biosensor based on conducting copolymer bound lactate oxidase, Sens. Actuators, B, 2005, 107(2), 768–772 CrossRef CAS.
- J. Huang,
et al., Development of an amperometric l-lactate biosensor based on l-lactate oxidase immobilized through silica sol–gel film on multi-walled carbon nanotubes/platinum nanoparticle modified glassy carbon electrode, Mater. Sci. Eng., C, 2008, 28(7), 1070–1075 CrossRef CAS.
- J. Huang,
et al., A highly-sensitive L-lactate biosensor based on sol-gel film combined with multi-walled carbon nanotubes (MWCNTs) modified electrode, Mater. Sci. Eng., C, 2007, 27(1), 29–34 CrossRef CAS.
- A. Lupu,
et al., Development of a potentiometric biosensor based on nanostructured surface for lactate determination, Sens. Actuators, B, 2007, 127(2), 606–612 CrossRef CAS.
- B. Haghighi and S. Bozorgzadeh, Fabrication of a highly sensitive electrochemiluminescence lactate biosensor using ZnO nanoparticles decorated multiwalled carbon nanotubes, Talanta, 2011, 85(4), 2189–2193 CrossRef CAS PubMed.
- A. C. Pereira,
et al., Development of a carbon paste electrode for lactate detection based on Meldola's blue adsorbed on silica gel modified with niobium oxide and lactate oxidase, Electroanalysis, 2011, 23(6), 1470–1477 CrossRef CAS.
- F. J. Gondim,
et al., Determination of the anaerobic threshold and maximal lactate steady state speed in equines using the lactate minimum speed protocol, Comp. Biochem. Physiol., Part A: Mol. Integr. Physiol., 2007, 146(3), 375–380 CrossRef PubMed.
- N. Hirst,
et al., An amperometric lactate biosensor using H2O2 reduction via a Prussian Blue impregnated poly (ethyleneimine) surface on screen printed carbon electrodes to detect anastomotic leak and sepsis, Sens. Actuators, B, 2013, 186, 674–680 CrossRef CAS.
|
This journal is © The Royal Society of Chemistry 2022 |
Click here to see how this site uses Cookies. View our privacy policy here.