DOI:
10.1039/D2AN00543C
(Paper)
Analyst, 2022,
147, 2828-2833
A novel AIE fluorescent probe for the monitoring of aluminum ions in living cells and zebrafish†
Received
29th March 2022
, Accepted 5th May 2022
First published on 9th May 2022
Abstract
A novel fluorescent probe BTD with aggregation induced emission (AIE) characteristics for the monitoring of Al3+ was developed. This fluorescent probe could be used to detect Al3+ in aqueous solution under mild conditions, along with high sensitivity and high selectivity. The detection limit of the probe BTD for Al3+ is as low as 3.25 nM, which is below the WHO recommendation concentration (7.41 μM) for drinking water. Furthermore, this probe was successfully applied to the sensing of Al3+ in living cells and zebrafish.
Introduction
Aluminum, the third most abundant element on the Earth's crust, is widely used in daily life, such as in utensils, food additives, building equipment, water purification, and pharmaceutical products.1–3 However, as a non-essential toxic element in the biological system, the accumulation of excessive Al3+ could induce various diseases, including Parkinson's disease, Alzheimer's disease, and amyotrophic lateral sclerosis.4–9 Moreover, the water-soluble Al3+ resulted by acid rain could cause serious damage to growing plants and the environment.10–12 Therefore, the development of an effective method to trace Al3+ is of great significance to human health and environment protection.
Though traditional analysis and detection techniques, such as chromatography, spectroscopy, and electrochemical analysis, are available to detect heavy transition metal ions, they are not convenient for the analysis of plentiful samples because of expensive equipment, high cost and time-consuming procedures.13–19 By contrast, significant progress has been achieved in the development and application of fluorescent probes for the selective binding and identification of different metals, exhibiting unique properties including operational simplicity, low cost, high sensitivity and high selectivity.20,21 Until now, some fluorescent probes for detecting Al3+ have been reported based on internal charge transfer (ICT),22–24 photo-induced electron transfer (PET),25–29 chelation-enhanced fluorescence (CHEF),30–32 and fluorescence resonance energy transfer (FRET) mechanisms.33 However, most of these probes need to be applied in the organic/water mixture for the detection of Al3+; the Al3+ ion easily dissolves in water medium. The poor water-solubility of these reported probes hinders their further application in environmental and biological systems. In recent years, fluorescent probes displaying aggregation-induced emission (AIE) characteristics have attracted great attention, and a lot of practical applications have been realized.34–40 Hence, it is valuable to develop new AIE probes with high selectivity and high sensitivity for the quantification and bioimaging of Al3+.
In this work, we rationally design and develop a novel fluorescent probe BTD with AIE characteristics, which exhibits fluorescence ‘turn-on’ for Al3+ in almost pure water (Scheme 1). This probe exhibits high sensitivity and high selectivity for the monitoring of Al3+ in water samples. Furthermore, the probe was successfully applied to detect Al3+ in BEL-7402 cells and zebrafish.
 |
| Scheme 1 Proposed mechanism for sensing Al3+. | |
Experimental
General information
The chemicals and solvents were purchased from Aladdin (Shanghai, China) and used without further purification. TLC analysis was performed using precoated silica plates. UV–vis absorption spectra were obtained on a UV-2450 spectrophotometer (Shimadzu Co., Japan). All the experimental pure water was obtained from a Millopore-Q water ultrapurification system. 1H NMR and 13C NMR spectra were obtained with tetramethyl silane (TMS) as the internal standard on a BRUKER AVANCE-500 spectrometer and the chemical shifts (δ) were expressed in ppm and coupling constants (J) were in Hertz. The IR spectrum was recorded on a NEXUS with KBr pellets and reported in cm−1. Mass analyses were performed using a Waters Q-Tof Premier mass spectrometer with an ESI ion source (Waters QTOF Premier, U.S.A.). Cells were imaged using a fluorescence confocal microscope (Zeiss LSM 880, Germany). Zebrafish experiments were conducted on a fluorescence confocal microscope system (Leica SP8, Germany).
General procedure for UV-visible spectroscopy and fluorescence measurements
The stock solution of probe BTD (1.0 mM) was prepared in DMSO. Stock solutions (10.0 mM) of various metal ions including Na+, K+, Li+, Ag+, Ba2+, Ca2+, Co2+, Zn2+, Mg2+, Mn2+, Pb2+, Cd2+, Cu2+, Sn2+, Fe2+, Fe3+, Cr3+, Bi3+, Ni2+, Zr4+, Ge4+, Cys, Hcy, GSH, S2−, SO32−, Gly, Glu, Met, Asp, Thr, Lys, Try, and His were prepared in deionized water. These stock solutions were further diluted to the required concentration for measurement. Test solutions were prepared as follows: 20 μL of BTD solution (1.0 mM) and appropriate amounts of analyte solution were added into a test tube, and the solution was diluted to 2 mL with pure water. All the absorption and fluorescence spectra were obtained at room temperature after shaking well.
Synthesis of 4-(benzo[d]thiazol-2-yl)phenol (1)
To a solution of 150 mL anhydrous DMF were added 2-aminobenzenethiol (18.78 g, 150 mmol) and 4-hydroxybenzaldehyde (18.32 g, 150 mmol); then, sodium pyrosulfite (24.52 g, 129 mmol) was added to the resulting solution. The mixture was refluxed for 4 h with stirring until no starting materials were indicated by TLC. The reaction mixture was cooled to room temperature, and then poured into 1 L of cold water, and a white solid was precipitated. Then the solid was filtered and dried under vacuum to yield the target compound 1 (28.8 g, 84.5%). 1H NMR (500 MHz, DMSO-d6) δ 10.22 (s, 1H), 8.07 (d, J = 8.0 Hz, 1H), 7.98 (d, J = 8.2 Hz, 1H), 7.93 (d, J = 8.6 Hz, 2H), 7.49 (t, J = 7.6 Hz, 1H), 7.39 (t, J = 7.6 Hz, 1H), 6.94 (dd, J = 8.9, 2.5 Hz, 2H). 13C NMR (126 MHz, DMSO-d6) δ 167.9, 161.0, 154.2, 134.6, 129.5, 126.9, 125.4, 124.5, 122.8, 122.6, 116.6.
Synthesis of 5-(benzo[d]thiazol-2-yl)-2-hydroxybenzaldehyde (2)
To a solution of 4-(benzo[d]thiazol-2-yl) phenol (5.00 g, 22 mmol) in trifluoroacetic acid (120 mL) was added hexamethylenetetramine (3.70 g, 26.4 mmol); then the mixture was refluxed for 5 h. After the reaction mixture was cooled to room temperature, hydrochloric acid (100 mL, 6 mol L−1) was added, and the organic layer was separated out. The aqueous layer was extracted with CH2Cl2 (3 × 10 mL). Then the combined organic layers were washed with brine, dried with anhydrous Na2SO4 and concentrated under vacuum. The crude product was purified by column chromatography to yield a white solid (1.80 g, 32%). 1H NMR (500 MHz, CDCl3) δ 11.27 (s, 1H), 10.03 (s, 1H), 8.35 (d, J = 2.3 Hz, 1H), 8.22 (dd, J = 8.7, 2.3 Hz, 1H), 8.05 (d, J = 8.1 Hz, 1H), 7.91 (dd, J = 8.0, 1.3 Hz, 1H), 7.53–7.48 (m, 1H), 7.42–7.38 (m, 1H), 7.12 (d, J = 8.8 Hz, 1H). 13C NMR (126 MHz, CDCl3) δ 196.4, 166.0, 163.5, 154.0, 135.7, 134.8, 132.8, 126.6, 126.1, 125.3, 123.1, 121.7, 120.7, 118.6.
Synthesis of the probe BTD
To a solution of 10 mL ethanol were added 5-(benzo[d]thiazol-2-yl)-2-hydroxybenzaldehyde (300 mg, 1.18 mmol) and 2-hydrazinylpyridine (128 mg, 1.18 mmol). The resulting mixture was stirred overnight at room temperature. Then the residue was filtered and washed with cold ethanol three times. The solid was purified by column chromatography to yield the desired probe (291 mg, 71.5%). 1H NMR (500 MHz, DMSO-d6) δ 11.10 (d, J = 26.6 Hz, 2H), 8.44–8.32 (m, 2H), 8.15 (dd, J = 5.0, 1.8 Hz, 1H), 8.11 (d, J = 7.9 Hz, 1H), 8.03 (d, J = 8.0 Hz, 1H), 7.92 (dd, J = 8.5, 2.4 Hz, 1H), 7.72–7.66 (m, 1H), 7.56–7.49 (m, 1H), 7.47–7.39 (m, 1H), 7.13 (d, J = 8.3 Hz, 1H), 7.07 (d, J = 8.5 Hz, 1H), 6.80 (dd, J = 7.2, 4.9 Hz, 1H). 13C NMR (126 MHz, DMSO-d6) δ 167.6, 158.9, 156.8, 154.2, 148.5, 138.6, 137.1, 134.7, 129.1, 127.0, 125.8, 125.5, 125.0, 122.9, 122.7, 122.1, 117.4, 115.7, 106.8.
Cell imaging studies
The BEL-7402 cells were cultured in Dulbecco's modified Eagle's medium (DMEM, GIBCO) supplemented with 10% heat-inactivated fetal bovine serum (FBS, GIBCO), streptomycin (100 μg mL−1) and penicillin (100 μg mL−1). The cells were seeded into a 96 well culture plate and grown in DMEM medium under a humid atmosphere of 5% CO2 at 37 °C for 24 h. Subsequently, the cells were washed thrice with sterile phosphate buffered saline (PBS). For the detection of Al3+ in living cells, BEL-7402 cells were incubated with 2.0 μM probe BTD in DMEM at 37 °C for 30 min and subsequently incubated with Al2(SO4)3·18H2O (0.1, 0.2 and 0.4 mM) for 30 min, respectively, and then washed with phosphate-buffered saline (PBS) three times. Cells were imaged using a fluorescence confocal microscope (Zeiss LSM 880, Germany). (λex = 405 nm, λem = 421–475 nm for the blue channel; λex = 458 nm, λem = 500–550 nm for the green channel). Scale bar: 50 μm.
Zebrafish confocal fluorescence imaging
For the detection of Al3+in vivo, 3-day-old zebrafish was used for these experiments. All animal procedures were performed in accordance with the guidelines and approved by the Animal Ethics Committee (Hunan Normal University). Zebrafish was fed with E3 embryo media (15 mM NaCl, 0.5 mM KCl, 1 mM MgSO4, 1 mM CaCl2, 0.15 mM KH2PO4, 0.05 mM Na2HPO4, 0.7 mM NaHCO3, 10−5% methylene blue; pH 7.5) at 28 °C for 30 min, then incubated with Al3+ (10, 20 and 50 μM, 60 min) respectively, and finally incubated with the probe (5 μM) for 60 min. All the fishes were terminally anaesthetized using MS222, and images were obtained on a fluorescence confocal microscope system (Leica SP8, Germany). (λex = 405 nm, λem = 440–500 nm for the blue channel; λex = 488 nm, λem = 510–550 nm for the green channel). Scale bar: 0.5 mm.
Results and discussion
Aggregation-induced emission (AIE) of the probe BTD
The probe BTD was easily synthesized, as shown in Scheme 2, via a three-step process. The probe and intermediates were characterized by 1H NMR and 13C NMR (Fig. S13–S18†). Firstly, the AIE behavior of the probe in the presence of Al3+ was verified by obtaining the fluorescence spectra of the probe BTD with Al3+ in mixed solvents with varied acetone-to-H2O ratios. In pure acetone, almost no fluorescence was observed under excitation at 345 nm (Fig. 1). After the addition of water, the phenolic hydroxyl group, the N atoms on the pyridine and the C
N double bond have a unique affinity for Al3+ ions, resulting in the aggregation of the probe BTD, and the fluorescence intensities were gradually increased at 470 nm. The fluorescence intensity at 470 nm was enhanced with the increase in water content. And the content of 99% water leads to a ca. 49-fold increment in the fluorescence intensity. The changes in blue fluorescence could be obviously observed under UV light (Fig. 1, insert). These results suggested that the probe BTD was an AIE-active probe for the sensing of Al3+ ions.
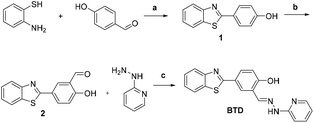 |
| Scheme 2 Synthesis of the probe BTD. Reagents and conditions: (a) DMF, Na2S2O5 reflux; 84.5%; (b) CF3COOH, HMTA, reflux; 32%; (c) EtOH, at room temperature; 71.5%. | |
 |
| Fig. 1 (a) Normalized fluorescence spectra of the probe BTD (10 μM) in the presence of Al3+ (100 μM) in different water contents from 0% to 100%. (b) The corresponding normalized fluorescence intensity changes at 470 nm. λex = 345 nm, silt: 2.5/2.5. The inset photos indicated the color changes of the probe BTD (10 μM) in the presence of Al3+ (100 μM) in different water contents (0–100%) under a UV lamp at 365 nm, respectively. | |
Spectroscopic response
Next, we examined the responses of the probe BTD (10 μM) in pure water (containing 1% DMSO) toward Al3+ at 37 °C. As shown in Fig. S1,† upon the addition of 100 μM Al3+, the initial absorption peak at 321 nm shifted to 327 nm. Meanwhile, the fluorescence spectrum of the probe BTD exhibited a weak fluorescence at 542 nm. After the probe was treated with 100 μM Al3+, a significant fluorescence increment was observed at 470 nm by more than 338 times when excited at 345 nm, accompanied by a clear color change from green to blue (inset images of Fig. S1†). Using quinoline sulfate in 0.1 M H2SO4 aqueous solution as the standard, the fluorescence quantum yield was calculated to be 0.157. These results demonstrated that the probe BTD was a visual and fluorescence turn-on probe for the detection of Al3+ in pure water.
Time-dependence and pH effect
The response time of the probe BTD with Al3+ in pure water (containing 1% DMSO) was also investigated. Upon addition of Al3+, the fluorescence at 470 nm increased rapidly and reached equilibrium after 25 minutes (Fig. S2†). Therefore, subsequent experiments for the monitoring of different analytes with the probe BTD were conducted for 25 min. Subsequently, the pH effect of the probe BTD for the monitoring of Al3+ was observed. As shown in Fig. S3,† the fluorescence of the probe BTD remained the same in the pH range of 2–10, indicating that the probe BTD was stable in a wide pH range. However, in the presence of Al3+, it showed significant fluorescence enhancements at pH 2–10. Thus, the probe BTD can be used for the detection of Al3+ over a wide pH range, including a physiologically relevant pH value, which is favorable for its biological applications in living cells and in vitro.
Titration experiments
To evaluate the sensing ability of the probe BTD for Al3+, fluorescence titration experiments were conducted. As shown in Fig. 2, the fluorescence at 470 nm enhanced gradually after the addition of Al3+ (0–10 μM). A good linear relationship was obtained in the Al3+ concentration range of 0–10 μM. And the detection limit of the probe BTD for Al3+ was calculated to be as low as 3.25 nM based on the signal-to-noise ratio (S/N = 3), which is as low as 7.41 μM Al3+ for bottled drinking water according to the World Health Organization (WHO) guidelines. Compared to some reported probes for Al3+ (Table S1†), this probe exhibited high sensitivity. These results indicated that the probe BTD is sensitive for the detection of Al3+ in environmental and biological systems.
 |
| Fig. 2 (a) Normalized fluorescence intensity spectra of the probe BTD (10 μM) in the presence of 0–10 μM Al3+ in pure water (containing 1% DMSO). (b) The linear changes in the normalized fluorescence intensity at 470 nm of the probe BTD and as a function of Al3+ concentration. λex = 345 nm, slit (nm): 2.5/2.5. | |
Selectivity and anti-interference
Subsequently, the selectivity of the probe BTD toward Al3+ was evaluated. Water-soluble metal species were tested, including Na+, K+, Li+, Ag+, Ba2+, Ca2+, Co2+, Zn2+, Mg2+, Mn2+, Pb2+, Cd2+, Cu2+, Sn2+, Fe2+, Fe3+, Cr3+, Bi3+, Ni2+, Zr4+ and Ge4+. As shown in Fig. 3, among these analytes, only Al3+ could induce a significant fluorescence enhancement, while no fluorescence was observed for the other competing analytes. Competition experiments were carried out in the presence of various metal ions and Al3+ under the same conditions (Fig. S4†). The fluorescence changes of the probe solution caused by the coexistence of other ions with Al3+ and the presence of Al3+ alone are similar, except for Co2+, Cu2+, Sn2+, Fe2+, Fe3+ and Ni2+, which could slightly reduce the fluorescence intensity at 470 nm. These results demonstrated that the probe BTD had good selectivity and anti-interference ability for the detection of Al3+ and has the potential for monitoring Al3+ in complex systems.
 |
| Fig. 3 (a) Normalized fluorescence spectra responses of the probe BTD (10 μM) to various metal ions in pure water (containing 1% DMSO). (b) The corresponding normalized fluorescence intensity changes at 470 nm. Various metal ions (100 μM) including: 1. None; 2. Na+; 3. K+; 4. Li+; 5. Ag+; 6. Ba2+; 7. Ca2+; 8. Co2+; 9. Zn2+; 10. Mg2+; 11. Mn2+; 12. Pb2+; 13. Cd2+; 14. Cu2+; 15. Sn2+; 16. Fe2+; 17. Fe3+; 18. Cr3+; 19. Bi3+; 20. Ni2+; 21. Zr4+; 22. Ge4+; 23. Al3+. λex = 345 nm, slit (nm): 2.5/2.5. | |
Reversibility
Reversibility and regeneration are also essential factors for the development of devices for sensing analytes in practical applications.41–53 We first evaluated the fluorescence response of the in situ product BTD-Al3+ toward other analytes, such as Cys, Hcy, GSH, S2−, SO32−, Gly, Glu, Met, Asp, Thr, Lys, Try, and His. As shown in Fig. S5,† fluorescence at 470 nm was significantly quenched only after Lys was added to the solution of the product BTD-Al3+. However, negligible fluorescence changes were observed for other analytes under the same conditions. Subsequently, the fluorescence intensity of the solution of the product BTD-Al3+ was rapidly quenched for 2 min upon addition of 500 μM Lys (Fig. S6†). As shown in Fig. S7,† the alternate addition of a constant amount of Al3+ (50 μM) and Lys (500 μM) to the aqueous solution of probe BTD would result in switchable changes in the fluorescence intensity at 470 nm. And this reversible fluorescence interconversion could be repeated more than 4 times by the modulation of the Al3+/Lys addition. These results indicated that the probe BTD could be applied to the reversible detection of Al3+ in environment and biological systems. In addition, the probe–Al3+ complex could be used to detect lysine (Fig. S8†).
Sensing mechanism
The sensing mechanism of the probe BTD toward Al3+ was carefully investigated. The Job's plot titration experiments of the probe BTD with Al3+ were first carried out to determine the binding ratio between the probe BTD and Al3+. As shown in Fig. S9,† the maximum fluorescence intensity was obtained when the molar ratio of the probe BTD with Al3+ was 0.5, indicating a 1
:
1 metal–ligand chelation ratio between the probe BTD and Al3+. Mass spectrometry analysis was further conducted, as shown in Fig. S10.† The peak at m/z 565.15 was attributed to [BTD − Al3+ + 2SO42− + H+]− [calcd, m/z: 564.97]. Moreover, the FT-IR spectra of free probe BTD and the complex of probe BTD and Al3+ were obtained, respectively (Fig. S11†). These results further confirmed the binding mode of the probe BTD with Al3+. According to the analyses of Job's plot, ESI-MS and FT-IR spectra, the proposed mechanism for the sensing of Al3+ using the probe BTD was reasonable (Scheme 1).
Detection of Al3+ in real water samples
To verify whether the probe BTD could detect Al3+ ions in real water samples or not, this probe was applied to quantify Al3+ ions in Xiangjiang water and tap water. As shown in Table 1, after the addition of 3 μM and 6 μM Al3+ ions to different water samples respectively, good results were obtained and the recovery of Al3+ ranged from 97.3% to 102.2%. Thus, the probe BTD was suitable to detect Al3+ ions in the water samples.
Table 1 Quantification of Al3+ with the probe BTD in real water samples. RSD (%, n = 3)
Samples |
Al3+ (μM) |
Spiked (μM) |
Detected (μM) |
Recovery (%) |
RSD (%) |
Xiang jiang River |
0.00 |
3.00 |
2.92 |
97.3 |
0.67 |
6.00 |
5.94 |
99.0 |
0.52 |
Tap water |
0.00 |
3.00 |
2.94 |
98.0 |
0.85 |
6.00 |
6.13 |
102.2 |
1.23 |
Imaging Al3+ in living cells
Encouraged by the aforementioned results, the probe BTD was further applied to the imaging of Al3+ in living cells using a laser scanning confocal microscope. According to the results from MTT assays in BEL-7402 cells, the probe BTD has low cytotoxicity towards living cells (Fig. S12†). As shown in Fig. 4, when BEL-7402 cells were incubated with the probe BTD (2.0 μM) for 30 min, a faint green fluorescence was observed (Fig. 4, A1–A3), while, after the addition of Al3+ (0.1–0.4 mM), blue fluorescence was observed (Fig. 4, B1–D3). These results indicated that the probe BTD was successfully applied to the imaging of Al3+ in living cells.
 |
| Fig. 4 Confocal fluorescence images of exogenous Al3+ in BEL-7402 cells. (A1–D3) The cells were incubated with the probe BTD (2.0 μM) for 30 min and then incubated with 0.1 mM, 0.2 mM and 0.4 mM Al3+ for 30 min, respectively. (λex = 405 nm, λem = 421–475 nm for the blue channel; λex = 458 nm, λem = 500–550 nm for the green channel.) Scale bar: 50 μm. | |
Imaging of Al3+ in zebrafish
Finally, the probe BTD was applied to image Al3+ in zebrafish. As shown in Fig. 5, when the zebrafish were treated with the probe BTD (5 μM) for 60 min, a green fluorescence was observed. When the zebrafish was preincubated with Al3+ (10, 20, and 50 μM, respectively) and then incubated with the probe BTD, a green fluorescence disappeared, and the blue fluorescence was increased. These results demonstrated that the probe BTD is an effective candidate for the monitoring of Al3+ fluctuation in zebrafish.
 |
| Fig. 5 Confocal fluorescence imaging of exogenous Al3+ in zebrafish. Top row: zebrafish were incubated with the probe BTD (5 μM) for 60 min. Others: zebrafish were preincubated with 10, 20 and 50 μM Al3+ for 60 min and then incubated with the probe BTD (5 μM) for 60 min, respectively. (λex = 405 nm, λem = 440–500 nm for the blue channel; λex = 488 nm, λem = 510–550 nm for the green channel). Scale bar: 0.5 mm. | |
Conclusions
In summary, a novel fluorescent probe BTD for the monitoring of Al3+ has been developed based on the AIE mechanism. The probe BTD is easily prepared through a simple three-step process, which could detect Al3+ in water samples under mild conditions. And this probe exhibited a fast response, high selectivity, and good sensitivity toward Al3+. Furthermore, the probe BTD was successfully applied to the sensing of Al3+ in living cells and zebrafish, which would be helpful for the understanding of the pathological functions of Al3+ in biological systems.
Author contributions
Yabing Gan: methodology, investigation, formal analysis, and writing – original draft. Guoxing Yin: investigation and validation. Zhaomin Xu: investigation and validation. Jianhua Wang: conceptualization, methodology, and writing – review & editing. Peng Yin: supervision, conceptualization, funding acquisition, project administration, and writing – review & editing.
Conflicts of interest
There are no conflicts to declare.
Acknowledgements
This work was supported by the funding from the National Natural Science Foundation of China (Grant No. 21877035).
Notes and references
- E. Delhaize and P. R. Ryan, Plant Physiol., 1995, 107, 315–321 CrossRef CAS PubMed.
- B. Valeur and I. Leray, Coord. Chem. Rev., 2000, 205, 3–40 CrossRef CAS.
- H. Okubo, Proc. Natl. Acad. Sci. U. S. A., 1988, 85, 3888–3892 CrossRef PubMed.
- S. W. King, J. Savory, M. R. Wills and H. J. Gitelman, Crit. Rev. Clin. Lab. Sci., 1981, 14, 1–20 CrossRef CAS PubMed.
- I. S. Parkinson, M. K. Ward and D. N. Kerr, J. Clin. Pathol., 1981, 34, 1285 CrossRef CAS PubMed.
- T. P. Flaten, Brain Res. Bull., 2001, 55, 187–196 CrossRef CAS PubMed.
- V. K. Gupta, A. K. Jain and G. Maheshwari, Talanta, 2007, 72, 1469–1473 CrossRef CAS PubMed.
- G. D. Fasman, Coord. Chem. Rev., 1996, 149, 125–165 CrossRef CAS.
- L. K. Kumawat, N. Mergu, M. Asif and V. K. Gupta, Sens. Actuators, B, 2016, 231, 847–859 CrossRef.
- Neeraj, A. Kumar, S. K. Asthana, Shweta and K. K. Upadhyay, J. Photochem. Photobiol., A, 2016, 329, 69–76 CrossRef CAS.
- W. A. Banks and A. J. Kastin, Neurosci. Biobehav. Rev., 1989, 13, 47–53 CrossRef CAS.
- V. Rondeau, H. Jacqmin-Gadda, D. Commenges, C. Helmer and J.-F. Dartigues, Am. J. Epidemiol., 2009, 169, 489–496 CrossRef PubMed.
- M. Frankowski, A. Zioła-Frankowska and J. Siepak, Talanta, 2010, 80, 2120–2126 CrossRef CAS PubMed.
- R. N. Goyal, V. K. Gupta and S. Chatterjee, Biosens. Bioelectron., 2009, 24, 3562–3568 CrossRef CAS PubMed.
- C.-H. Guo, G.-S. W. Hsu, C.-J. Chuang and P.-C. Chen, Environ. Toxicol. Pharmacol., 2009, 27, 176–181 CrossRef CAS PubMed.
- H. Wang, Z. Yu, Z. Wang, H. Hao, Y. Chen and P. Wan, Electroanalysis, 2011, 23, 1095–1099 CrossRef CAS.
- B. J. Sanghavi and A. K. Srivastava, Analyst, 2013, 138, 1395–1404 RSC.
- B. J. Sanghavi, S. Sitaula, M. H. Griep, S. P. Karna, M. F. Ali and N. S. Swami, Anal. Chem., 2013, 85, 8158–8165 CrossRef CAS PubMed.
- N. S. Gadhari, B. J. Sanghavi and A. K. Srivastava, Anal. Chim. Acta, 2011, 703, 31–40 CrossRef CAS PubMed.
- B. M. Luby, D. M. Charron, C. M. MacLaughlin and G. Zheng, Adv. Drug Delivery Rev., 2017, 113, 97–121 CrossRef CAS PubMed.
- J. A. Kaczmarski, J. A. Mitchell, M. A. Spence, V. Vongsouthi and C. J. Jackson, Curr. Opin. Struct. Biol., 2019, 57, 31–38 CrossRef CAS PubMed.
- Y.-J. Liu, F.-F. Tian, X.-Y. Fan, F.-L. Jiang and Y. Liu, Sens. Actuators, B, 2017, 240, 916–925 CrossRef CAS.
- P. Torawane, K. Tayade, S. Bothra, S. K. Sahoo, N. Singh, A. Borse and A. Kuwar, Sens. Actuators, B, 2016, 222, 562–566 CrossRef CAS.
- Z. Li, C. Liu, J. Wang, S. Wang, L. Xiao and X. Jing, Spectrochim. Acta, Part A, 2019, 212, 349–355 CrossRef CAS PubMed.
- G. T. Selvan, M. Kumaresan, R. Sivaraj, I. V. M. V. Enoch and P. M. Selvakumar, Sens. Actuators, B, 2016, 229, 181–189 CrossRef CAS.
- M. Mukherjee, B. Sen, S. Pal, A. Maji, D. Budhadev and P. Chattopadhyay, Spectrochim. Acta, Part A, 2016, 157, 11–16 CrossRef CAS PubMed.
- M. A. Kozlov, D. Y. Uvarov, S. A. Gorbatov, N. G. Kolotirkina, A. D. Averin, V. V. Kachala, K. A. Lyssenko, I. V. Zavarzin and Y. A. Volkova, Eur. J. Org. Chem., 2019, 4196–4206 CrossRef CAS.
- J.-H. Wang, D.-R. Gao, X.-Y. Wang, Y.-M. Lu, W.-X. Shen and Y.-Y. Lv, Sens. Actuators, B, 2019, 294, 14–23 CrossRef CAS.
- G. Zhu, Y. Huang, C. Wang, L. Lu, T. Sun, M. Wang, Y. Tang, D. Shan, S. Wen and J. Zhu, Spectrochim. Acta, Part A, 2019, 210, 105–110 CrossRef CAS PubMed.
- S. Mukherjee, P. Mal and H. Stoeckli-Evans, J. Lumin., 2016, 172, 124–130 CrossRef CAS.
- L. Bai, F. Tao, L. Li, A. Deng, C. Yan, G. Li and L. Wang, Spectrochim. Acta, Part A, 2019, 214, 436–444 CrossRef CAS PubMed.
- Y. Li, Q. Niu, T. Wei and T. Li, Anal. Chim. Acta, 2019, 1049, 196–212 CrossRef CAS PubMed.
- M. Arduini, F. Felluga, F. Mancin, P. Rossi, P. Tecilla, U. Tonellato and N. Valentinuzzi, Cheminform, 2003, 34, 1606–1607 CrossRef.
- Y. Hong, J. W. Y. Lam and B. Z. Tang, Chem. Soc. Rev., 2011, 40, 5361–5388 RSC.
- Y. Liu, Y. Tang, N. N. Barashkov, I. S. Irgibaeva, J. W. Y. Lam, R. Hu, D. Birimzhanova, Y. Yu and B. Z. Tang, J. Am. Chem. Soc., 2010, 132, 13951–13953 CrossRef CAS PubMed.
- X. Chen, X. Y. Shen, E. Guan, Y. Liu, A. Qin, J. Z. Sun and B. Z. Tang, Chem. Commun., 2013, 49, 1503–1505 RSC.
- J. Wu, F. Du, P. Zhang, I. A. Khan, J. Chen and Y. Liang, J. Inorg. Biochem., 2005, 99, 1145–1154 CrossRef CAS PubMed.
- Z. Qiao, Y. Wu, B. Tang, R. Perestrelo and R. Bhalla, Tetrahedron Lett., 2019, 60, 150918 CrossRef CAS.
- J. Luo, Z. Xie, J. W. Lam, L. Cheng, H. Chen, C. Qiu, H. S. Kwok, X. Zhan, Y. Liu and D. Zhu, Chem. Commun., 2001, 18, 1740–1741 RSC.
- Y. Hong, J. W. Y. Lam and B. Z. Tang, Chem. Commun., 2009, 40, 4332–4353 RSC.
- Y.-J. Tang, S. He, X.-F. Guo and H. Wang, Analyst, 2021, 146, 7740–7747 RSC.
- Y. Ma, W. Gao, L. Zhu, Y. Zhao and W. Lin, Analyst, 2020, 145, 1865–1870 RSC.
- Y. Zhang, Y. Zuo, T. Yang, Z. Gou, X. Wang and W. Lin, Analyst, 2019, 144, 5075–5080 RSC.
- J. Xiong, Z. Li, J. Tan, S. Ji, J. Sun, X. Li and Y. Huo, Analyst, 2018, 143, 4870–4886 RSC.
- M. Ren, L. Wang, X. Lv, Y. Sun, H. Chen, K. Zhang, Q. Wu, Y. Bai and W. Guo, Analyst, 2019, 144, 7457–7462 RSC.
- Q. Wu, L. Feng, J. B. Chao, Y. Wang and S. Shuang, Analyst, 2021, 146, 4348–4356 RSC.
- Z. Liu, X. Zhou, Y. Miao, Y. Hu, N. Kwon, X. Wu and J. Yoon, Angew. Chem., Int. Ed., 2017, 56, 5812–5816 CrossRef CAS PubMed.
- H. Ren, F. Huo and C. Yin, New J. Chem., 2021, 45, 9096–9101 RSC.
- W. Zhang, T. Liu, F. Huo, P. Ning, X. Meng and C. Yin, Anal. Chem., 2017, 89, 8079–8083 CrossRef CAS PubMed.
- T.-B. Ren, S.-Y. Wen, L. Wang, P. Lu, B. Xiong, L. Yuan and X.-B. Zhang, Anal. Chem., 2020, 92, 4681–4688 CrossRef CAS PubMed.
- Y. Ma, W. Gao, L. Zhu, Y. Zhao and W. Lin, Chem. Commun., 2019, 55, 11263–11266 RSC.
- X. Liu, X. Gong, J. Yuan, X. Fan, X. Zhang, T. Ren, S. Yang, R. Yang, L. Yuan and X.-B. Zhang, Anal. Chem., 2021, 93, 5420–5429 CrossRef CAS PubMed.
- Y. Ma, Y. Tang, Y. Zhao and W. Lin, Anal. Chem., 2019, 91, 10723–10730 CrossRef CAS PubMed.
|
This journal is © The Royal Society of Chemistry 2022 |
Click here to see how this site uses Cookies. View our privacy policy here.