DOI:
10.1039/D1AY01700D
(Paper)
Anal. Methods, 2022,
14, 58-66
Dinitrobenzene ether reactive turn-on fluorescence probes for the selective detection of H2S†
Received
6th October 2021
, Accepted 18th November 2021
First published on 18th November 2021
Abstract
Two novel fluorescent probes, namely, 3-(2,4-dinitrophenoxy)-2-(4-(diphenylamino)phenyl)-4H-chromen-4-one (P1) and 3-(2,4-dinitrophenoxy)-2-(pyren-1-yl)-4H-chromen-4-one (P2), were designed and synthesized here. The probes (P1 and P2) were found to be highly selective and sensitive toward hydrogen sulfide (H2S) in the presence of a wide range of anions. The new probes (P1 and P2) were fully characterized by analytical, NMR spectroscopy (1H and 13C), and ESI mass spectrometry. The sensing capability of chemodosimeters (P1 and P2) toward H2S was confirmed by fluorescence studies. The ‘turn-on’ fluorescence was used to calculate the detection limit of probes (LOD), which were found to be 2.4 and 1.2 μM for P1 and P2, respectively. Moreover, the probes were tested for their cytotoxicity against HeLa cells using the MTT assay and found to be non-cytotoxic in nature; hence, the probes P1 and P2 were successfully utilized to visualize H2S in the living cells.
Introduction
One of the important and effective species required for the regulation of intracellular metabolic processes and other signaling mechanisms in the biotic system is hydrogen sulfide (H2S).1,2 H2S generally belongs to RSS (reactive sulfur species) family, which also includes nitrosothiols, sulfonic acids, thiols, and sulfur dioxide derivatives.3–5 When compared to other signaling species such as CO (carbon monoxide) and NO (nitric oxide), the H2S species will directly target the active proteins by coordinating with the iron centers of heme proteins and also through the biosynthetic process such as cysteine sulfhydration.6,7 Not only in protein metabolisms, it also subsidizes its role in many health impacts such as diabetes, widening of blood vessels (vasodilation), apoptosis, Down's syndrome, angiogenesis (terrible growth of blood vessels), inflammation in mucous membranes, liver cirrhosis, Alzheimer's diseases, and neuromodulation (alteration in the nerve activity due to exposure toward H2S gas).8–12 The H2S molecules, on the other hand, protect the human body against the IRI disease (ischemia–reperfusion injury) caused due to the lack of oxygen in blood.13–15 In addition to the biological issues, the H2S species also make some crucial disturbances in ecological systems by inhibiting the plant functions such as growth and affects aquatic life.16 Because of the above consequences, some of the current researches were carried out to detect the H2S species in living biotic samples.17
Numerous analytical methods are available to detect the H2S species, which include electrochemical assays, metal-induced sulfide precipitation, colorimetric, voltammetry, and chromatographic techniques.18–20 However, the above methods lead to unparallel results because of their less sensitivity toward detection. These methods generally become less important in the present day and are not mostly preferred because of the fact that the analyses need a sample pre-treatment, are time consuming, less selective, less sensitive, complicated procedures, lead to the destruction of cells and tissues (cell lysis), and also need expensive equipment.21–23 Hence, to overcome these limitations, an effective tracking system must be introduced to detect H2S species in the natural and living systems. In the recent years, fluorimetric probes or fluorescent chemosensors have become renowned as effective molecules to detect the H2S species because they possess many boons such as selective interactions with analytes, capable of detecting analytes even in nanomolar concentration, on-time spatial imaging, high biocompatibility, and also it does not cause any damage to living cells and tissues during the detection of targeted analytes.24–29
In the span of years, researchers have reported a variety of fluorescent probes for H2S detection based on its strong reducibility, nucleophilicity, or thiolysis properties. For example, Kim et al.30 designed a reaction-based fluorescent probe AHS for H2S detection via the reduction of azide. The probe AHS showed excellent reactivity toward H2S and was capable of detecting H2S in biosamples. Ding et al.31 prepared new fluorescent probes for the selective and quantitative detection of H2S based on the nucleophilic addition reaction. A ratiometric probe DNPOCy was synthesized by Maity et al.32 to detect H2S in live cells. Upon the addition of H2S in the probe solution, the color change from greenish yellow to blue was readily observed by the “naked-eye”. A diethylaminocoumarin–hemicyanine-based ratiometric probe was synthesized and utilized by Yang et al.33 for determining H2S in biological species. Furthermore, a couple of elegant probes have been designed by splitting the dinitrophenyl ether group to restore their original fluorescence. Zhang et al.34 designed a colorimetric fluorescent molecule that possesses azo dye with copper(II) for the recognition of the H2S species with high selectivity. For instance, Peng et al. designed and synthesized a novel turn-on H2S fluorescent probe to detect H2S based on the thiolysis reaction on the dinitrophenyl ether bond in which the phenanthroimidazole derivative acts as a fluorophore.35 Mahapatra et al.36 synthesized the probe DPQI (2-{2-[8-(2,4-dinitro-phenoxy)-quinolin-2-yl]-vinyl}1,3,3-trimethyl-3H-indolium), in which the quinoline skeleton is used as a color reporting group and the DNB moiety can quench the fluorescence via the photoinduced electron transfer (PET) effect. A novel ESIPT fluorescence probe HBTSeSe was designed and synthesized by Guo et al.37 The design strategy was based on the nucleophilic attack of H2S on the Se–Se bond, followed by the thiolysis of ether to release the HBT (2-(2′-hydroxyphenyl)benzothiazole) fluorophore. The probe responded selectively and rapidly toward H2S with the increase in the fluorescence intensity by 47-fold. Feng et al.38 developed a new NBD ether-based probe DC-NBD for the rapid, selective, and sensitive detection of H2S. This probe shows significant turn-on fluorescence changes for H2S in the NIR region with a remarkably large Stokes' shift.
Although great advances have been made, numerous limitations in practical use remain to be overcome. For instance, some probes employing the azido group as the sensing group usually show a long reaction time (≈20 min)39–43 or need to use surfactants to promote the reactions,44,45 which might be a disadvantage for the real-time tracking and bio-imaging of reactive and transient H2S in vivo. In a few instances, potentially competing species such as cysteine (Cys), homocysteine (Hcy), and glutathione (GSH) interfere with the detection process in nucleophilic reactions46–51 since they share similar chemical properties. Therefore, developing new fluorophores is crucial for gaining fluorescence probes with superior properties.
Keeping the above in perspective, in this work, distinct fluorophores triphenylamine aldehyde and 1-pyrenecarbaldehyde, were utilized to design two novel probes, namely, 3-(2,4-dinitrophenoxy)-2-(4-(diphenylamino)phenyl)-4H-chromen-4-one (P1) and 3-(2,4-dinitrophenoxy)-2-(pyren-1-yl)-4H-chromen-4-one (P2). These probes show selective targeting activity toward H2S species on the basis of aromatic nucleophilic substitution reaction, which in turn leads to excellent enhancement in their fluorescence property. Moreover, “in vitro” studies for the probes were employed to image the changes in the live cells by interacting them with the H2S species in order to apply the probes for clinical diagnosis.
Experimental section
Materials and methods
Analar grade (pure) chemicals and solvents were acquired from Sigma-Aldrich or Merck and used throughout the experiments without any extra purification. Doubly distilled fresh water was used all through the preparation processes. Technico micro heating table was used to measure the melting points. Vario EL III Elemental analyzer was used to get the analytical data. The emission spectra were recorded on a JASCO FP-8200 spectrophotometer using a 1 cm square pure silica quartz cuvette at room temperature in DMSO–H2O (1
:
9) medium at pH 7.4. The 1H-NMR (400 MHz) and 13C-NMR (100 MHz) spectra were recorded on a Bruker AMX spectrometer using DMSO4-d6 or CDCl3. The chemical shift (δ) values were measured in ppm units using the internal standard tetramethylsilane (TMS). The Q-TOF micro™ mass spectrometer was used to perform the electrospray ionization mass spectra (ESI mass spectra) of the synthesized compounds. Bioimaging was performed using a Fluorescence IX71 microscope (Japan, Olympus). The precursor compound triphenylamine aldehyde used for the synthesis of probe P1 has been synthesized according to the method reported in the previous work.52
General procedure for the synthesis of the probes
The following two-step procedures were used for the synthesis of probes P1 and P2.
Synthesis of 2-(4-(diphenylamino)phenyl)-3-hydroxy-4H-chromen-4-one (2).
o-Hydroxy acetophenone (1) (0.136 g, 1 mmol) was added to EtOH–H2O (20 mL, 5
:
1, v/v) solvents containing 10% NaOH. After stirring the above solution at room temperature for 30 min, an ethanol (5 mL) solution of 4-(diphenyl)aminobenzaldehyde (0.273 g, 1 mmol) was added slowly with constant stirring and kept at room temperature for 2 h. Then, the whole mixture was refluxed at 80 °C for 16 h, resulting in a red colored residue. The residue was filtered and washed with cold ethanol. The obtained red solid was again dissolved in 10 mL ethanol containing 1.5 mL of NaOH (10%) and then 1.5 mL of H2O2 (30%) solution was added dropwise to the reaction mixture and allowed to stir for 2 h at room temperature. The resulting reddish brown reaction mixture was poured on ice cold water for the formation of a solid compound. The formed solid was filtered, washed with water, and purified by column chromatography (petroleum ether, ethyl acetate, 9
:
1, v/v) to give a brown solid (compound 2).
Yield: 0.3235 g (82%), color: brown, mp: 218 °C. Rf: 0.48. Anal. calc. for C27H19NO3: C, 79.98; H, 4.72; N, 3.45%. Found: C, 80.38; H, 5.12; N, 3.75%. 1H NMR (400 MHz, DMSO-d6, ppm): 11.26 (s, 1H, OH), 8.23–8.19 (d, 2H, Ar H), 8.14–8.12 (d, 2H, Ar H), 7.68–7.66 (t, 1H, Ar H), 7.41–7.38 (t, 2H, Ar H), 7.14–7.11 (d, 2H, Ar H). 13C NMR (100 MHz, CDCl3, ppm): 180.35 (C
O), 133.80 (Ar C), 131.67 (Ar C), 131.28 (Ar C), 128.82 (Ar C), 127.93 (Ar C), 127.49 (Ar C), 127.41 (Ar C), 126.34 (Ar C), 126.06 (Ar C), 126.02 (Ar C), 125.31 (Ar C), 124.81 (Ar C), 124.74 (Ar C), 123.03 (Ar C), 118.52 (Ar C).
Synthesis of 3-(2,4-dinitrophenoxy)-2-(4-(diphenylamino)phenyl)-4H chromen-4-one (P1).
Compound 2 (0.109 g, 0.24 mmol) and 1-fluoro-2,4-dinitrobenzene (0.116 g, 0.6 mmol) were dissolved in chloroform (20 mL), and then 0.5 mL triethylamine was added to the reaction mixture in a dropwise manner and stirred at room temperature. The reaction process was constantly monitored by TLC. When the reaction is completed, the solvent present in the reaction mixture was evaporated using a rotary evaporator, leaving behind a red colored solid. Using silica gel column chromatography, the crude product formed was purified using petroleum ether and ethyl acetate (9
:
1 v/v) eluents.
Yield: 0.3612 g (78%), color: brown, mp: 262 °C. Rf: 0.63. Anal. calc. for C33H21N3O7: C, 69.35; H, 3.70; N, 7.35%. Found: C, 69.65; H, 4.06; N, 7.62%. 1H NMR (400 MHz, DMSO-d6, ppm): 8.88 (s, 1H, OH), 8.65–8.68 (d, 2H, Ar H), 8.32–8.34 (d, 2H, Ar H), 8.03–8.05 (d, 2H, Ar H), 7.92–7.95 (t, 2H, Ar H), 7.85–7.89 (t, 4H, Ar H), 7.56–7.61 (t, 4H, Ar H), 7.38–7.40 (d, 2H, Ar H), 6.91–6.93 (d, 4H, Ar H). 13C NMR (100 MHz, DMSO-d6, ppm): 167 (C
O), 157 (C–N), 146 (Ar C), 131 (Ar C), 126 (Ar C), 125 (Ar C), 127 (Ar C), 121 (Ar C), 119 (Ar C). MS (ESI, calcd, found, m/z): 571.5, 572.1 [M]+.
Synthesis of 3-hydroxy-2-(pyrene-1-yl)-4H-chromen-4-one (3).
The synthesis of compound 3 was carried out similar to that of the procedure used for compound 2 using o-hydroxyacetophenone and pyrene aldehyde.
Yield: 0.3235 g (80%), color: yellow, mp: 194 °C. Rf: 0.53. Anal. calc. for C25H14O3: C, 82.86; H, 3.89%. Found: C, 83.17; H, 4.07%. 1H NMR (400 MHz, DMSO-d6, ppm): 10.18 (s, 1H, OH), 8.87–8.83 (t, 2H, Ar H), 8.61–8.57 (t, 1H, Ar H), 8.57–8.59 (d, 2H, Ar H), 8.29–8.31 (d, 2H, Ar H), 8.08–8.10 (d, 4H, Ar H), 7.74–7.72 (d, 2H, Ar H), 7.63–7.64 (d, 2H, Ar H). 13C NMR (100 MHz, CDCl3, ppm): 173.29 (C
O), 133.81 (Ar C), 131.27 (Ar C), 129.58 (Ar C), 128.85 (Ar C), 127.95 (Ar C), 127.51 (Ar C), 127.41 (Ar C), 126.09 (Ar C), 126.03 (Ar C), 125.33 (Ar C), 125.31 (Ar C), 124.79 (Ar C), 123.05 (Ar C).
Synthesis of 3-(2,4-dinitrophenoxy)-2-(pyren-1-yl)-4H-chromen-4-one (P2).
The probe (P2) was synthesized similar to that of the procedure used for probe P1 using compound 3 and 1-fluoro-2,4-dinitrobenzene.
Yield: 0.3612 g (75%), color: red, mp: 218 °C. Rf: 0.72. Anal. calc. for C31H16N2O7: C, 70.46; H, 3.05; N, 5.30%. Found: C, 70.69; H, 3.23; N, 5.43%. 1H NMR (400 MHz, DMSO-d6, ppm): 8.24 (s. 1H, Ar H), 8.15–8.13 (d, 4H, Ar H), 7.88–7.85 (t, 4H, Ar H), 7.73–7.75 (d, 2H, Ar H), 7.56–7.58 (d, 2H, Ar H), 7.31–7.54 (t, 2H, Ar H), 7.29–7.32 (d, 2H, Ar H), 7.10–7.12 (d, 2H, Ar H). 13C NMR (100 MHz, DMSO-d6, ppm): 172 (C
O), 161 (C–N), 155 (Ar C), 151 (Ar C), 149 (Ar C), 140 (Ar C), 138 (Ar C), 135 (Ar C), 133 (Ar C), 131 (Ar C), 128 (Ar C), 127 (Ar C), 125 (Ar C), 123 (Ar C), 120. MS (ESI, calcd, found, m/z): 528.4, 529.5 [M + H]+.
Results and discussion
The target compounds P1 and P2 were synthesized by two-step reaction sequences as per Scheme 1. The formation of the compounds was confirmed by spectral (1H NMR, 13C NMR, and ESI-mass) and analytical methods (ESI, Fig. S1–S10†). The sensing ability of the probes was analyzed through fluorescence emission spectroscopic studies.
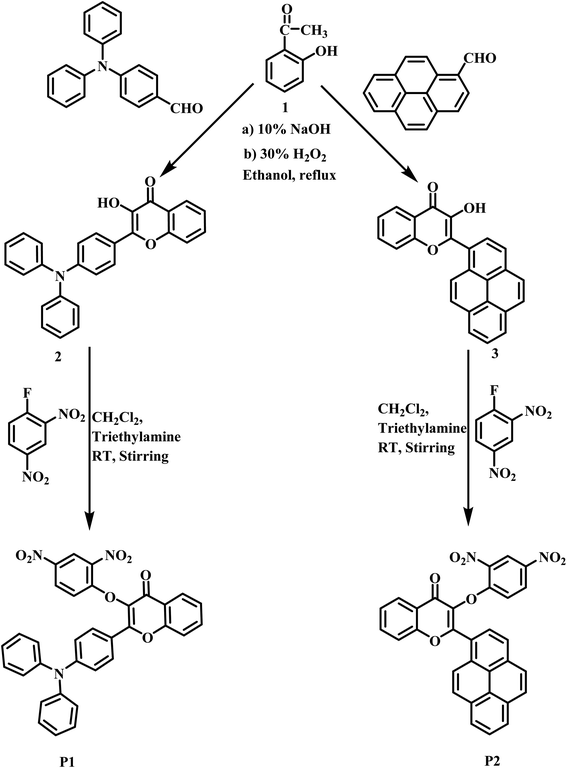 |
| Scheme 1 Synthesis of probes P1 and P2. | |
Fluorescence study
The sensing ability of the probes P1 and P2 was analyzed using fluorescence emission spectral studies in HEPES buffer medium (10 mM having 1% DMSO, pH = 7.4) in the presence of H2S (NaHS is the source of H2S) and common anions (Br−, F−, Cl−, I−, CO32−, HCO3−, NO32−, AcO−, SO42−, SCN− all with sodium salts and CN− with tetrabutylammonium salt) at room temperature and the results are depicted in Fig. 1a and b. Probe P1 exhibits a weak fluorescence emission at 540 nm and upon the addition of 20 μM solution of NaHS, there is a remarkable enhancement in the fluorescence intensity at 540 nm with a blue shift. The increment in the fluorescence intensity happens due to the aromatic nucleophilic substitution reaction taking place in probe P1 in the presence of H2S species, which leads to the cleavage of the alkoxy bond with the activation of the excited state intramolecular proton transfer (ESIPT) in the probe. However, the addition of other anions did not lead to any significant fluorescence intensity of probe P1. Similarly, probe P2 was examined under the same physiological conditions. Probe P2 exhibits weak fluorescence emission at 500 nm, which gets enhanced remarkably with the addition of 20 μM NaHS solution.
 |
| Fig. 1 Fluorescence responses of the probe P1 (a) and P2 (b) (5 μM) in DMSO/H2O (1 : 1 (v/v), HEPES = 50 mM, pH = 7.4) towards 20 μM of H2S (NaHS is source of H2S) and common anions (Br−, F−, Cl−, I−, CO32−, HCO3−, NO32−, AcO−, SO42−, SCN− all with sodium salts and CN− with tetrabutylammonium salt). | |
The sensitivity of probes P1 and P2 was examined by fluorescence titration experiments using the probes (P1 and P2) against various concentrations of NaHS (0–20 μM). As shown in Fig. 2a and b, weak fluorescence appeared for the probes at 540 nm (P1) and 500 nm (P2), which get increased gradually on the incremental addition of NaHS (0–20 μM) to the probes with red (P1) and blue (P2) shift. The increasing fluorescence intensity becomes saturated while reaching the probe concentration of 20 μM. The plot of fluorescence intensity vs. probe concentration shows a good linear agreement between them (Fig. 3a and b). From Fig. 3a and b, the detection limit of the probes (LOD) P1 and P2 was estimated using the formula 3σ/S (σ is the standard deviation of the probe S = slope from the linear plot) and was found to be 2.4 and 1.2 μM, respectively. The detection limits indicate that the probes P1 and P2 are highly sensitive and suitable for application in living systems since the physiologically relevant concentration of H2S is estimated to be in the range from nanomolar to millimolar levels.53
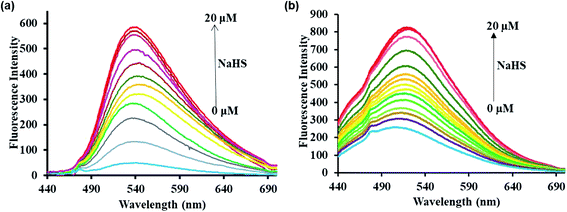 |
| Fig. 2 Fluorescence responses of the probes P1 (a) and P2 (b) (5 μM) in DMSO/H2O (1 : 9 (v/v), HEPES = 50 mM, pH = 7.4) against 0–20 μM of NaHS. | |
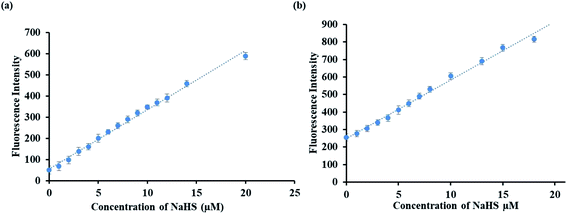 |
| Fig. 3 Detection limit plot of the probes P1 (a) and P2 (b) towards NaHS. | |
Interference study is an important experiment used to find the selectivity as well as performance of the synthesized probes P1 and P2. During the study, the probes P1 and P2 were added with other interfering anions and biothiols such as F−, Cl−, CN−, CO32−, N3−, SCN−, Br−, I−, SO32−, HSO3, Cys, GSH, and Hcy, in addition to H2S, and the fluorescence spectra were recorded (ESI, Fig. S11 and S12†). As illustrated in Fig. S11 and S12,† the fluorescence enhancement was not affected by any other anion or biothiol, which reveals the selective sensing behavior of probes P1 and P2 for H2S in the presence of other competitive species. The results show that the probes could be used as selective sensors for the determination of H2S over other interfering species.
The time-dependent fluorescence study was carried out to analyze the sensing ability of the probes P1 and P2 toward H2S. The emission intensities of probes P1 and P2 increase gradually with increasing time and become saturated within 10 and 6 min, respectively (ESI Fig. S13 and S14†). When compared to P2, the delay in the detection ability of probe P1 may be due to the steric effect among the 2,4-dinitrophenyl ether (DNP) moiety and the fluorophoric unit, which leads to a slight hindrance in ether thiolysis by nucleophilic substitution reaction but this will not affect the sensitivity of the probe toward H2S detection.
Mechanism of sensing of H2S by P1 and P2
The mechanism of the sensing of probes P1 and P2 with H2S was evidenced by 1H NMR titration and mass spectral studies. 1H NMR titration experiments were carried out to confirm the binding between probes P1 and P2 with H2S. The spectra of probes P1 and P2 alone show peaks in the aromatic region at 6.0–8.8 ppm. However, the spectra of the probes after the addition of NaHS show new –OH (phenolic) proton peaks at 12.26 and 10.08 ppm besides the aromatic peaks (Fig. 4a and b). The results reveal that the addition of NaHS to the probes (P1 and P2) cleave the alkoxy bond by the aromatic nucleophilic substitution reaction, which leads to the release of 2,4-dinitrobenzene (electron withdrawing group) and the free fluorophore.
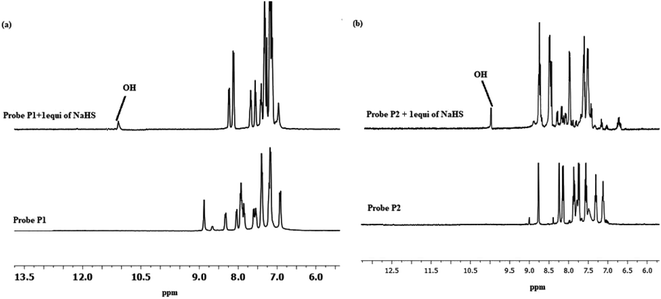 |
| Fig. 4
1H NMR spectra of (a) probe P1 alone (bottom) and probe P1 with 1 equivalent of NaHS (top) (b) probe P2 alone (bottom) and probe P2 with 1 equivalent of NaHS (top). | |
Moreover, the mass spectra results provide further evidence for the above mechanism. The mass spectra of the probes after the addition of NaHS were recorded and the spectra show a molecular ion peak at m/z 406.1 and 363.4 (ESI, Fig. S15 and S16†), which are equal to the molecular masses of compounds 2 and 3. The formation of compounds 2 and 3 confirms the nucleophilic substitution reaction in the alkoxy bond of the probes.
pH study
To verify the sensing abilities of probes P1 and P2 toward H2S in various pH medium, the fluorescence emission intensities were recorded with and without the addition of H2S in different pH range (2–14). The result shows that the probes P1 and P2 were stable in the pH range of 2 to 14. However, the addition of NaHS leads to an increase in the emission intensity of the probes P1 and P2 in the pH range of 6–11 (ESI, Fig. S17 and S18†). The results reveal that probes P1 and P2 could detect H2S in a wide range of pH.
Life time study
The fluorescence lifetime decay experiment was done and the lifetime of the probes P1 and P2 were found to be 1.32 and 1.24 ns (at λem = 440 nm), respectively. On adding NaHS to the probes P1 and P2, there is an increase in the lifetime from 1.32 to 1.83 and 1.24 to 1.67, respectively (Fig. 5a and b). The average life gets enhanced due to the cleavage of the alkoxy bond, the removal of the electron-withdrawing group, and the activation of the ESIPT mechanism.
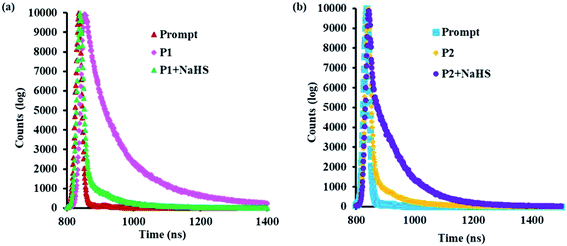 |
| Fig. 5 Fluorescence lifetime decay profiles of (a) P1 and P1 + NaHS (b) P2 and P2 + NaHS. | |
Based on the above spectroscopic results, a possible mechanism for the sensing of H2S by probes P1 and P2 was proposed in Scheme 2.
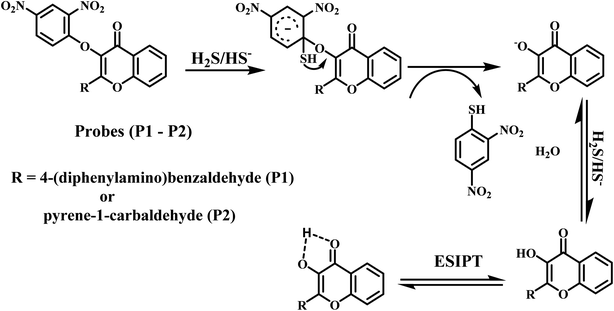 |
| Scheme 2 Schematic representation of H2S sensing by the probes P1 and P2. | |
Applications in cell imaging
Due to the high sensitivity and selective turn on fluorescence changes, P1 and P2 were expected to be good probes for bioimaging applications. For live cell imaging, biocompatibility is always the first consideration. Hence, the probes were tested for their cytotoxicity against HeLa cells using the MTT assay. For this assay, primarily, the cells were incubated with different concentrations (0, 10, 20, 30, 40, and 50 μM) of the probes P1 and P2 for 48 h at 37 °C. The results reveal that cell viability is not even affected at concentrations of the probes P1 and P2 in the culture medium as high as 50 μM (ESI, Fig. S19†). Therefore, the cell line imaging observations were carried out, and the fluorescent pictures of the HeLa cells were captured using a fluorescence microscope. The results (Fig. 6) show that no fluorescence occurs when the cells were preliminarily treated for 1 h with the probes (5 μM) P1 and P2 due to the weak fluorescence ability of the probes. Furthermore, with the treatment of the cells with 20 μM solution of NaHS, there is an occurrence of green fluorescence in the cells. These observations were captured using a fluorescence microscope and the results clearly show that the probes P1 and P2 are cell permeable and have high potential ability toward the monitoring of H2S in live cells.
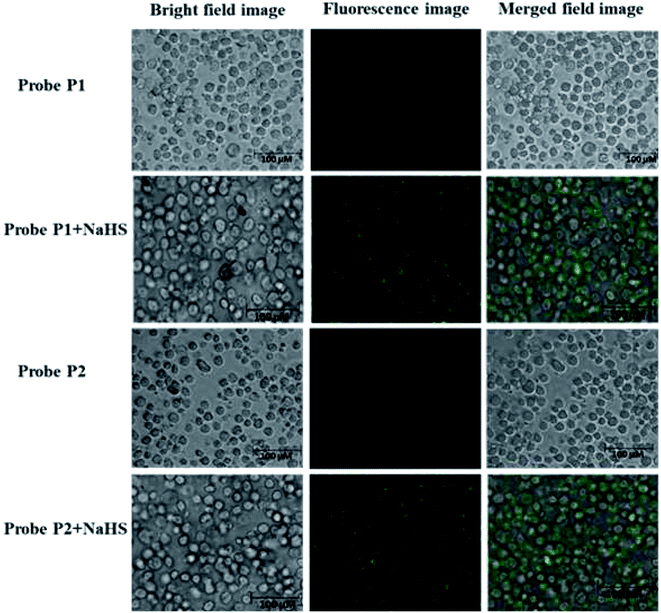 |
| Fig. 6 Cell imaging studies of probes P1 (5 μM) and P2 (5 μM) along with NaHS (20 μM). | |
The present probes P1 and P2 were compared with already reported chemosensors (ESI, Table S1†). The comparison results reveal that the present probes P1 and P2 show comparable detection limit.17,18,35,36,54 On the other hand, the probes P1 and P2 exhibit superior performance than the reported probes while comparing the response time.17,18,54–57 Hence, it is emphasized that the present probes P1 and P2 could play a better analytical sensor role for the detection of H2S with low detection limit, less response time, and no interference with other ions.
Conclusions
From this work, we summarize that we have effectively designed and synthesized two novel ‘turn-on’ luminescent probes P1 and P2 for the rapid detection of H2S. The probes P1 and P2 not only show rapid response but also possess excellent sensitivity and superior selectivity toward hydrogen sulfide detection when compared to other common biothiols and anions in physiological conditions. In addition to this, the sensing mechanism for H2S detection on the basis of ether thiolysis reaction was reinforced by 1H NMR and ESI-MS studies. Subsequently, the developed probes P1 and P2 were successfully applied to live cell imaging studies.
Author contributions
Rajasekaran Dhivya: formal analysis. Investigation, writing – original draft; Venkatachalam Kavitha: validation, visualization; Asaithambi Gomathi: visualization; Ponmudi Keerthana: investigation; Narayanan Santhalakshmi: investigation; Periasamy Viswanathamurthi: conceptualization, funding acquisition, methodology, validation, supervision, project administration, writing – review & editing; Jebiti Haribabu: investigation.
Conflicts of interest
There are no conflicts of interest to declare.
Acknowledgements
The authors express their sincere thanks to Council of Scientific and Industrial Research (CSIR), New Delhi, India [grant no. 01 (2907)/17/EMR-II] and University Grants Commission (UGC), India [SAP grant no. 540/20/DRS-I/2016(SAP-I)] for financial assistance.
References
- L. Zhou, D. Lu, Q. Wang, S. Liu, Q. Lin and H. Sun, Biosens. Bioelectron., 2017, 91, 699–705 CrossRef CAS PubMed.
- K. Zhang, J. Zhang, Z. Xi, L. Li, X. Gu, Q. Zhang and L. Yi, Chem. Sci., 2017, 8, 2776–2781 RSC.
- M. K. Copinot, R. Guo, D. A. Tocher, A. B. M. Buanz, S. Gaisford, S. L. Price and D. Bucar, Cryst. Growth Des., 2017, 17, 827–833 CrossRef.
- P. Kumar, V. Kumar and R. Gupta, RSC Adv., 2017, 7, 7734–7741 RSC.
- S. Kumar, R. Kishan, P. Kumar, S. Pachisia and R. Gupta, Inorg. Chem., 2018, 57, 1693–1697 CrossRef CAS.
- V. Kumar, P. Kumar, S. Panday and R. Gupta, Dalton Trans., 2018, 47, 9536–9545 RSC.
- C. H. Min, S. Na, J. E. Shin, J. K. Kim, T. G. Jo and C. Kim, New J. Chem., 2017, 41, 3991–3999 RSC.
- L. K. Wareham, Biochem. Soc. Trans., 2018, 46, 1107–1118 CrossRef CAS PubMed.
- M. Gao, F. Yu, C. Lv, J. Choo and L. Chen, Chem. Soc. Rev., 2017, 46, 2237–2271 RSC.
- X. Zhu, H. Wu, X. Guo and H. Wang, Dyes Pigm., 2019, 165, 400–407 CrossRef CAS.
- N. Zhou, C. Yin, J. Chao, Y. Zhang and F. Huo, Sens. Actuators, B, 2019, 287, 131–137 CrossRef CAS.
- Y. Chen, X. Shang, C. Li, Z. Xue, H. Chen, H. Wu and T. Wang, Sci. Rep., 2018, 8, 16159–16168 CrossRef PubMed.
- A. Ji, Y. Fan, W. Ren, S. Zhang and H. Ai, ACS Sens., 2018, 3, 992–997 CrossRef CAS PubMed.
- J. Zhang, X. Ji, J. Zhou, Z. Chen, X. Dong and W. Zhao, Sens. Actuators, B, 2018, 257, 1076–1082 CrossRef CAS.
- Y. Ji, L. J. Xia, L. Chen, X. F. Guo, H. Wang and H. J. Zhang, Talanta, 2018, 181, 104–111 CrossRef CAS PubMed.
- M. Karger, H. R. Darabi, A. Sharifi and A. Mostashari, Analyst, 2020, 145, 2319–2330 RSC.
- D. Fu, W. Zhi, L. Lv, Y. Luo, X. Xiong, X. Kang, W. Hou, J. Yan, H. Zhao and L. Zheng, Spectrochim. Acta, Part A, 2020, 224, 117391–117400 CrossRef CAS PubMed.
- K. Liu, C. Liu, H. Shang, M. Ren and W. Lin, Sens. Actuators, B, 2018, 256, 342–350 CrossRef CAS.
- X. Shang, X. Yue, Y. Chen, C. Li, H. Chen and T. Wang, Inorg. Chem. Commun., 2019, 99, 1–10 CrossRef CAS.
- N. Paul, R. Sarkar, A. Barui and S. Sarkar, J. Chem. Sci., 2020, 132, 21–28 CrossRef CAS.
- W. Chen, P. Xie, X. Shan, H. Zhao, Y. Wu, H. Zhou and X. Jin, J. Mol. Struct., 2020, 1207, 127822–127827 CrossRef CAS.
- B. Gu, W. Su, L. Huang, C. Wu, X. Duan and Y. Li, Sens. Actuators, B, 2018, 255, 2347–2355 CrossRef CAS.
- L. Zhang, Y. Shi, Z. Sheng, Y. Zhang, X. Kai, M. Li and X. Yu, ACS Sens., 2019, 4, 3147–3155 CrossRef CAS PubMed.
- Y. An, P. Wang and Z. Yue, Spectrochim. Acta, Part A, 2019, 216, 319–327 CrossRef CAS PubMed.
- T. Cao, Z. Teng, D. Gong, J. Qian, W. Liu, K. Iqbal, W. Qin and H. Guo, Talanta, 2019, 198, 185–192 CrossRef CAS PubMed.
- S. H. Hwang, D. Yun, H. Lee, M. Kim, M. H. Kim, K. T. Kim and C. Kim, Dyes Pigm., 2019, 165, 264–272 CrossRef CAS.
- S. Kundu and P. Sahoo, New J. Chem., 2019, 43, 12369–12374 RSC.
- K. B. Li, W. Qu, Q. Shen, S. Zhang, W. Shi, L. Dong and D. M. Han, Dyes Pigm., 2020, 173, 107918–107924 CrossRef CAS.
- S. Chen, H. Li and P. Hou, Sens. Actuators, B, 2018, 256, 1086–1092 CrossRef CAS.
- G. Kim, E. Jang, A. M. Page, T. Ding, K. A. Carlson and H. Cao, RSC Adv., 2016, 6, 95920–95924 RSC.
- J. Ding, Y. Ge and B. Zhu, Anal. Sci., 2013, 29, 1171–1175 CrossRef CAS PubMed.
- D. Maity, A. Raj, P. K. Samanta, D. Karthigeyan, T. K. Kundu, S. K. Pati and T. Govindaraju, RSC Adv., 2014, 4, 11147 RSC.
- Y. Yang, L. He, K. Xu and W. Lin, New J. Chem., 2019, 43, 10926–10931 RSC.
- D. Zhang and W. Jin, Spectrochim. Acta, Part A, 2012, 90, 35–39 CrossRef CAS PubMed.
- S. Peng, T. Zhong, T. Guo, D. Shu, D. Meng, H. Liu and D. Guo, New J. Chem., 2018, 42, 5185–5192 RSC.
- U. N. Guria, K. Maiti, S. S. Ali, S. K. Samanta, D. Mandal, R. Sarkar, P. Datta, A. K. Ghosh and A. Mahapatra, New J. Chem., 2018, 42, 5367–5375 RSC.
- H. Guan, A. Zhang, P. Li, L. Xia and F. Guo, ACS Omega, 2019, 4, 9113–9119 CrossRef CAS PubMed.
- S. Gong, E. Zhou, J. Hong and G. Feng, Anal. Chem., 2019, 91, 13136–13142 CrossRef CAS PubMed.
- B. Gu, N. Mi, Y. Zhang, P. Yin, H. Li and S. Yao, Anal. Chim. Acta, 2015, 879, 85–90 CrossRef CAS PubMed.
- T. S. Bailey and M. D. Pluth, J. Am. Chem. Soc., 2013, 135, 16697–16704 CrossRef CAS PubMed.
- X. L. Liu, X. J. Du, C. G. Dai and Q. H. Song, J. Org. Chem., 2014, 79, 9481–9489 CrossRef CAS PubMed.
- A. R. Lippert, E. J. New and C. J. Chang, J. Am. Chem. Soc., 2011, 133, 10078–10080 CrossRef CAS PubMed.
- Z. Wu, D. Liang and X. Tang, Anal. Chem., 2016, 88, 9213–9218 CrossRef CAS PubMed.
- J. Zhang and W. Guo, Chem. Commun., 2014, 50, 4214–4217 RSC.
- X. Cao, W. Lin, K. Zheng and L. He, Chem. Commun., 2012, 48, 10529–10531 RSC.
- Q. Hu, C. Yu, X. Xia, F. Zeng and S. Wu, Biosens. Bioelectron., 2016, 81, 341–348 CrossRef CAS PubMed.
- D. Lee, G. Kim, J. Yin and J. Yoon, Chem. Commun., 2015, 51, 6518–6520 RSC.
- W. Chen, H. Luo, X. Liu, J. W. Foley and X. Song, Anal. Chem., 2016, 88, 3638–3646 CrossRef CAS PubMed.
- X. Chen, Y. Zhou, X. Peng and J. Yoon, Chem. Soc. Rev., 2010, 39, 2120–2135 RSC.
- C. Liu, B. Peng, S. Li, C. M. Park, A. R. Whorton and M. Xian, Org. Lett., 2012, 14, 2184–2187 CrossRef CAS PubMed.
- C. Liu, J. Pan, S. Li, Y. Zhao, L. Y. Wu, C. E. Berkman, A. R. Whorton and M. Xian, Angew. Chem., Int. Ed., 2011, 50, 10327–10329 CrossRef CAS PubMed.
- A. Ozdemir and S. Erdemir, J. Photochem. Photobiol., A, 2020, 390, 112328–112345 CrossRef CAS.
- J. Furne, A. Saeed and M. D. Levitt, Am. J. Physiol.: Regul., Integr. Comp. Physiol., 2008, 295, 1479–1485 CrossRef PubMed.
- X. Lin, Y. Chen, S. Wang, K. Liu and F. Kong, Anal. Bioanal. Chem., 2019, 411, 7127–7136 CrossRef CAS PubMed.
- J. Cui, T. Zhang, Y. Sun, D. Li, J. Liu and B. Zhao, Sens. Actuators, B, 2016, 232, 705–711 CrossRef CAS.
- S. Chen, C. Ma, D. Wang, X. Han, L. Zhang and J. Wang, Anal. Methods, 2015, 7, 7646–7652 RSC.
- C. Tang, Q. Zheng, S. Zong, Z. Wang and Y. Cui, Sens. Actuators, B, 2014, 202, 99–104 CrossRef CAS.
Footnote |
† Electronic supplementary information (ESI) available: Experimental procedures for cell imaging studies and MTT assay, 1H NMR and 13C NMR spectra of compounds 2, 3, P1, and P2, ESI-mass spectra of P1, P2, probe P1 + NaHS, and probe P2 + NaHS, interference studies, time-dependent fluorescence spectra, pH studies, cytotoxicity assay of probes P1 and P2, comparison table of the present probes with the reported probes. See DOI: 10.1039/d1ay01700d |
|
This journal is © The Royal Society of Chemistry 2022 |
Click here to see how this site uses Cookies. View our privacy policy here.