DOI:
10.1039/D1AY01716K
(Paper)
Anal. Methods, 2022,
14, 52-57
Metal-organic gel as a fluorescence sensing platform to trace copper(II)†
Received
9th October 2021
, Accepted 22nd November 2021
First published on 23rd November 2021
Abstract
Metal-organic gel (MOG), as a novel type of metallic organic hybrid material, exhibits diverse properties. However, its application in fluorescence detection for specific metal ions has rarely been exploited. In this work, we have designed and synthesized a MOG based on Al-carboxylate coordination assemblies (denoted as MOG-Al). The resultant MOG-Al shows good specific fluorescence signal response to trace Cu2+. Under optimal conditions, the fluorescence quenching degrees (F0 − F) of the MOG-Al have a linear correlation with Cu2+ concentration ranging from 0.05 to 100 μM, and the limit of detection (LOD) is 45.00 nM. The proposed sensing platform was also applied for the detection of Cu2+ in real samples. Satisfactory recoveries (92–116%) for Cu2+ in rice, soybean milk powder and pork liver were obtained. These results indicate that MOG-Al is a promising material for the specific and sensitive sensing of Cu2+.
Introduction
Cuprum is one of the essential elements in the human body,1 and plays an important role in certain physiological and pathological activities.2,3 In recent years, Cu2+ has become a huge concern throughout the world because of its toxicity and enrichment through the food chain,4 threatening the ecosystem and human health. For instance, excessive intake of Cu2+ can cause negative effects on human health, leading to diarrhea, vomiting and stomach upset.5 Intracellular disruption of Cu2+ homeostasis may cause oxidative stress and severe disorders,6 which result in various diseases like cancer,7 Parkinson's disease,8 and heart and skin diseases.9 Thus, the detection of Cu2+ has drawn intensive attention.
Conventional techniques for Cu2+ detection generally include inductively coupled plasma mass spectrometry,10 flame atomic absorption spectrometry11 and inductively coupled plasma optical emission spectrometry.12 Although these approaches demonstrate satisfactory results, their additional extraction and pre-concentration steps hamper their application for on-site detection. Recently, fluorescence detection methods have attracted great attention due to their advantages of high sensitivity and rapid measurement.13 Some fluorescent materials, including SiO2-anchored CdS nanocrystals,14 gold nanoclusters,15 quantum dots (QDs)16 and chitin grafted polyaniline,17 have been applied to detect Cu2+. Liu and co-workers18 used COFs as fluorescent probes for detecting Cu2+ in the range of 0.31 to 0.4 μM in a tetrahydrofuran medium. The development of COFs for the detection of Cu2+ in an organic environment limits their further application due to the poor solubility of metal ions in such a medium. A MOF was incubated with Cu2+ in aqueous solution for 180 min, and the fluorescence response showed a good linear correlation with different concentrations of Cu2+.19 Electrically responsive chitin grafted polyaniline17 has been reported for the detection of Cu2+ in the range of 1–103 ppm. These previous methods demonstrate success to a certain extent; however, there are still many problems to overcome, such as narrow detection range, poor solubility and long detection time. Therefore, a new material with better performance is still highly desired for Cu2+ detection.
Metal-organic gel (MOG) is constructed by noncovalent interactions (ionic attraction, π–π stacking, hydrogen bonding and electronic interactions) between metal ions and suitable organic ligands.20 MOGs, as a novel type of multifunctional metallic organic hybrid material, are attracting tremendous attention due to their unique properties such as desirable high surface area,21 stimuli-responsive properties,22 inherent open metal sites23 and rapid mass transfer.24 MOGs have been successfully applied in sorption,21 catalysis25 and porogen templating.26 Besides, the organic ligands endow MOGs with appealing fluorescence properties, which lead to their potential applications in fluorescence detection.27–29 Zr-MOG30 has demonstrated a rapid response to CrO42− in the range from 50.0 μM to 0.5 mM. However, MOGs as fluorescence probes are still in the initial stage, with more MOGs needed to meet the requirements of different analytes.
Herein, we designed and synthesized MOG-Al based on 1,2,4,5-tetrakis-(4-carboxyphenyl)-benzene (H4TCPB), 2,5-pyrazinedicarboxylic acid dihydrate (2,5-pzdc) and Al3+. The obtained MOG-Al exhibits strong fluorescence emission and excellent solubility in aqueous medium. Moreover, the fluorescence quenching degrees of MOG-Al are related to the concentration of Cu2+. Furthermore, MOG-Al shows good performance in real samples with satisfactory recovery. This opens a new field for MOGs in the fluorescence sensing of metal ions.
Results and discussion
Synthesis and characterization
In order to obtain a MOG with rapid mass transfer, H4TCPB was chosen as one of the ligands, which endows the resulting gel with a porous structure due to possessing a large three-dimensional spatial arrangement. Another ligand, 2,5-pzdc, with good water-solubility and nitrogen atoms in the pyrazine ring, was also utilized. In addition, Al3+ was chosen as the metal ion due to its gelation property. As shown in Scheme 1, MOG-Al was synthesized via solvothermal processes.
 |
| Scheme 1 Synthesis scheme of MOG-Al. | |
To characterize the gel properties, the obtained MOG-Al was first assessed by simply turning the sample upside down. As shown in Fig. S1,† MOG-Al displays a distinct gelatinous state. The morphology of MOG-Al exhibits a rough wave-like morphology and long cracks arrange randomly on the surface.
The functional groups of MOG-Al were analyzed by the FT-IR technique. As shown in Fig. 1b, H4TCPB and 2,5-pzdc show strong peak at 1693.45 cm−1 and 1695.38 cm−1, respectively, which are attributed to the C
O stretching vibration of carboxyl. For MOG-Al, the peaks at 1693.45 and 1695.38 cm−1 disappear, and a new peak at 1384.85 cm−1 associated with –COO– is observed, which proves the coordination interaction between Al3+ and the carboxyl group. In addition, the vibration band at 1616.30 cm−1 is clearly observed, which results from the stretching vibration of C
N in the pyrazine ring of 2,5-pzdc (Fig. 1b).
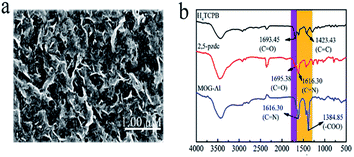 |
| Fig. 1 (a) SEM image of MOG-Al; (b) FT-IR spectra of H4TCPB, 2,5-pzdc, and MOG-Al. | |
Fluorescence response of MOG-Al to Cu2+
The fluorescence spectrum of MOG-Al shows a symmetric and strong fluorescence emission peak at 390 nm under a stimulation of 270 nm (Fig. 2a) in water. To select the appropriate detection conditions, the composition of buffer, pH and concentration of MOG-Al were systematically investigated.
 |
| Fig. 2 (a) Fluorescence excitation and emission spectra of MOG-Al (the concentration is fixed at 5.0 mg L−1). Cu2+-caused fluorescence quenching degrees in different buffers (b), pH (c) and concentration of MOG-Al (d). | |
Firstly, the fluorescence responses of MOG-Al to Cu2+ were investigated in the following buffers (pH 7.0): HEPES buffer, 3-(N-morpholino) propanesulfonic acid (MOPs) buffer, phosphate buffer (PB) and tris hydrochloride (Tri-HCl). Under the same concentration of Cu2+, MOG-Al exhibits the best fluorescence quenching response in the HEPES buffer (Fig. 2b), which may be attributed to the strong interaction between the gel and the metal ion in such a buffer system.31 Thus, HEPES was chosen for further experiments. Next, the effect of pH on the fluorescence quenching degree was evaluated. It can be observed that the fluorescence quenching degree gradually increases in the pH range of 5.0–8.0 and quickly declines from 8.0 to 9.0 (Fig. 2c). Thus pH 8.0 was employed for the subsequent experiments. The effects of MOG-Al concentration are presented in Fig. 2d; the fluorescence quenching degree is gradually enhanced within the MOG-Al concentration range of 1.0–7.0 mg L−1; thus 7.0 mg L−1 was used in further experiments. In summary, HEPES buffer (pH 8.0) and 7.0 mg L−1 MOG-Al were adopted for the subsequent experiments.
Fluorescence sensing of Cu2+
Under the optimal detection conditions, the fluorescence quenching degrees of MOG-Al caused by Cu2+ at different concentrations were investigated. As shown in Fig. 3a, the fluorescence emission of MOG-Al is gradually quenched with the increase in Cu2+ concentration, and plots of F0 − F vs. lg
CCu2+ display a good linear relationship within the range of 0.05–100 μM (Fig. 3b). The relationship between fluorescence quenching degree and lg
CCu2+ can be expressed as
F0 − F = 1958.37517 lg CCu2+ + 14408.11623 (R2 = 0.9996) |
where F0 and F are the fluorescence intensities of MOG-Al at 390 nm without and with Cu2+, respectively. The obtained LOD is 0.45 μM according to 3σ/k (where σ and k are the standard deviation of 10 blank measurements and the slope of the standard calibration curve, respectively).
 |
| Fig. 3 (a) Fluorescence spectra of MOG-Al incubation with different concentrations of Cu2+ (0–100 μM). (b) Calibration plot of F0 − F vs. lg CCu2+. | |
In comparison with previously reported approaches (Table 1), the proposed fluorescence sensing platform is advantageous in terms of detection range and LOD. The proposed approach has a broad detection range. In addition, the excellent stability and solubility in aqueous solution make MOG-Al suitable for the detection of Cu2+ in more complex systems.
Table 1 The comparison of the present approach with previous reports
Methods |
Materials |
Detection range (μM) |
LOD (nM) |
Ref. |
Fluorescence |
Ag-nanoclusters |
0.01–30 |
10 |
32
|
Fluorescein |
10–70 |
22.2 |
33
|
Carbon dots |
0.1–10 |
36 |
34
|
Si nanowires |
0.05–20 |
10 |
35
|
Dual-function chemosensors |
2–12 |
570 |
36
|
Carbon dot |
5–100 |
2400 |
37
|
BODIPY-based probe |
0.5–2.5 |
5500 |
38
|
APBHN |
1–18 |
148 |
39
|
CQDs |
10–50 |
1000 |
40
|
Cdots |
0.3–1.6 |
90 |
41
|
UCNPs |
0.1–2.0 |
57.8 |
42
|
MOG-Al |
0.05–100 |
45 |
This work |
Stability and selectivity of MOG-Al
To investigate its stability, MOG-Al (7.0 mg) was dispersed in HEPES (1.0 mL, pH 8.0) and the fluorescence intensity was measured at fixed intervals. As shown in Fig. S2,† MOG-Al shows insignificant changes within 8 h, which indicates satisfactory stability. To evaluate the selectivity of MOG-Al to the target ion (Cu2+), the responses of MOG-Al towards other ions (Al3+, Cr3+, In3+, Fe3+, Ba2+, Cd2+, Co2+, Ni2+, Pb2+, Sr2+, Zn2+, K+, Ag+ and Li+) were studied (Fig. 4a). The results show that only Cu2+ can significantly quench the fluorescence of MOG-Al, indicating the high selectivity of MOG-Al to Cu2+.
 |
| Fig. 4 (a) The fluorescence responses of MOG-Al to Cu2+ and interference ions. (b) The fluorescence quenching degree of MOG-Al to Cu2+ in binary systems. | |
The effect of coexisting metal ions on the fluorescence intensity of MOG-Al was further investigated in a binary system (Fig. 4b). It is very encouraging that MOG-Al shows high anti-interference capacity with other metal ions. Although MOG-Al shows a negative fluorescence response to Sr2+ in a single metal system, it does not interfere with the fluorescence response of MOG-Al to Cu2+ in the binary system. This may be attributed to the stronger combination of MOG-Al to Cu2+ than Sr2+.
Fluorescence sensing of Cu2+ in real samples
The high selectivity and excellent stability make MOG-Al a promising fluorescent probe for trace Cu2+ in complex samples. The fluorescence sensing of Cu2+ in rice, soybean milk powder and pork liver was investigated. As shown in Table 2, the recoveries are 93–100%, 98–113% and 98–116% for rice, soybean milk powder and pork liver, respectively. These results show the accuracy of MOG-Al for trace Cu2+ in real samples.
Table 2 Detection of Cu2+ in rice, soybean milk powder and pork liver
Samples |
Added (μM) |
Found (μM) |
Recovery (%) |
RSD (%) |
Rice |
0.07 |
0.07 |
97.43 |
2.76 |
0.10 |
0.10 |
99.60 |
4.39 |
1.00 |
0.97 |
97.40 |
4.13 |
10.00 |
9.27 |
92.70 |
4.25 |
Soybean milk powder |
0.07 |
0.07 |
98.14 |
0.64 |
0.10 |
0.11 |
113.00 |
3.02 |
1.00 |
0.99 |
99.02 |
2.14 |
10.00 |
10.30 |
103.00 |
6.52 |
Pork liver |
0.07 |
0.07 |
98.14 |
4.00 |
0.10 |
0.12 |
115.00 |
2.62 |
1.00 |
1.16 |
116.00 |
0.96 |
10.00 |
10.30 |
103.00 |
2.18 |
Sensing mechanism
To conduct a thorough investigation of the sensing mechanism, the fluorescence responses of H4TCPB and 2,5-pzdc to different concentrations of Cu2+ (1 μM, 10 μM and 100 μM) were tested (Fig. S3 and S4†). The fluorescence intensity of H4TCPB remains constant at low concentrations (1 and 10 μM) and then decreases when the concentration of Cu2+ increases to 100 μM. The fluorescence intensity of 2,5-pzdc decreases slowly from 95.45% to 76.66% with the increase of Cu2+ concentration, which demonstrates the interaction between 2,5-pzdc and Cu2+. Compared with that of MOG-Al, the fluorescence responses of the two monomers caused by Cu2+ are insignificant. We speculate that the sensing mechanism can be attributed to the simultaneous coordination of Cu2+ to the free carboxylate groups and the pyrazine ring within MOG-Al, which causes electron transfer from the ligand to Cu2+. The full scan XPS spectrum of MOG-Al (Fig. S5†) shows four peaks at 284.80 eV, 400.42 eV, 531.99 eV and 74.80 eV, which confirms the presence of C, N, O, and Al within MOG-Al, respectively. However, the signal of Cu2+ is too weak to observe. The high resolution XPS spectra of MOG-Al and MOG-Al loaded with Cu2+ were also measured. The O 1s spectra of MOG-Al (Fig. S6a†) shows two characteristic peaks at 531.96 eV and 532.01 eV, which are attributed to Al–O and C
O, respectively.43,44 When MOG-Al is incubated with Cu2+, the peak at 532.01 eV shifts to 530.33 eV, and the resulting lower energy level verifies the coordination interaction between Cu2+ and the carboxylate groups. In addition, the N 1s peak of the pyrazine ring shifts from 400.51 eV to 400.62 eV (Fig. S6c and d†), which further verifies that binding occurs between the nitrogen atoms of the pyrazine ring and Cu2+.45 The Al 2p high resolution XPS spectrum is divided into two peaks at 75.65 eV and 74.85 eV, which are attributed to Al–OH and Al–O, respectively (Fig. S6e†). As shown in Fig. S6f,† the binding energy of Al–OH and Al–O is lowered after the addition of Cu2+, which indicates the increase of the electron cloud density of Al. All results demonstrate that both the carboxylic group and nitrogen atom of the pyrazine ring dominate the fluorescence response of MOG-Al toward Cu2+.
Experimental
Materials
H4TCPB was purchased from Beijing HWRK Chem Co., Ltd (Beijing, China). 2,5-pzdc was purchased from Sigma-Aldrich, Inc. (New York, USA). Aluminium nitrate nonahydrate was purchased from the Tianjin Jiangtian Chemical Technology Co. Ltd (Tianjin, China). N-2-Hydroxyethylpiperazine-N′-2-ethanesulfonic acid (HEPES) was purchased from the Beijing Biotopped Science & Technology Co. Ltd (Beijing, China). All food samples were obtained from a local supermarket (Tianjin, China).
Characterization
Scanning electron microscopy (SEM) images, Fourier transform infrared (FT-IR) spectra, X-ray photoelectron spectroscopy (XPS) and fluorescence spectra were obtained with a LEO-1530VP (Zeiss, Germany), a Vector 22 FT-IR spectrophotometer in the range of 2500 to 500 cm−1, an Escalab 250xi photoelectron spectrometer (Thermo, USA) and an F-7000 fluorescence spectrometer (Hitachi, Japan), respectively. The investigated food samples were treated with the microwave digestion system MARS6 (Cem, USA).
Preparation of MOG-Al
H4TCPB (16.76 mg), 2,5-pzdc (12.25 mg), and aluminium nitrate nonahydrate (75.60 mg) were dissolved in 5.0 mL N,N-dimethylformamide (DMF), and nitric acid (0.10 M, 0.50 mL) was then added. The mixture was transferred into a high-pressure reactor vessel. Subsequently, the mixture was first heated at 100 °C for 24 h, and then cooled to 30 °C within 40 h. The product was collected by centrifugation and washed with DMF three times.
Fluorescence sensing of Cu2+
In a typical experiment, 1.00 mg MOG-Al was dissolved in 1.00 mL HEPES. Subsequently, 0.90 mL of the MOG-Al solution and 0.10 mL of different concentrations of Cu2+ solutions (0–100
μM) were transferred to cuvettes for further analysis. The fluorescence intensity of the system at 390 nm was recorded under excitation at 270 nm. For fluorescence interference experiments, interfering ions (Al3+, Cr3+, In3+, Fe3+, Ba2+, Cd2+, Co2+, Ni2+, Pb2+, Sr2+, Zn2+, K+, Ag+ and Li+) at the same concentration (0.10 μM) were added into the system containing MOG-Al (0.90 mL) and Cu2+ (0.10 mL). The fluorescence intensity of the system at 390 nm was recorded. All fluorescence measurements were performed in triplicate for statistical purposes.
Rice, soybean milk powder and pork liver were chosen as real samples to verify the proposed approach. The sample (0.20 g) was first digested in HNO3 (5.00 mL, 65%) and H2O2 (2.00 mL, 30%) by the microwave digestion system. The obtained solution was diluted to 50.00 mL with deionized water. Then, the diluted sample solution was purified through a 0.22 μm membrane. Finally, the matrix solution was obtained through an acidic digestion process and its fluorescence response was tested under the optimized experimental conditions.
Conclusions
In summary, a novel MOG was prepared based on H4TCPB, 2,5-pzdc and Al3+ via a solvothermal-ultrasonic method. The resultant MOG-Al demonstrated strong fluorescence emission and high stability, and was successfully applied for Cu2+ fluorescence detection in HEPES. Moreover, the practicability of the present MOG-Al was demonstrated by detecting Cu2+ in rice, soybean milk powder and pork liver, and satisfactory recoveries were obtained (92.7–116.0%). Compared with previous reports, MOG-Al has a wider detection range (0.05–100 μM) and lower LOD (45.0 nM), making it an ideal candidate for the detection of Cu2+ in complex samples.
Author contributions
Hao Qi: investigation, writing – original draft. Tianli Zhang, Chuang Jing: software, supervision, data curation, investigation. Zhen Zhang, Yujie Chen: conceptualization, methodology. Yali Chen: software, writing. Qiliang Deng, Shuo Wang: validation, data curation, writing – review, supervision.
Conflicts of interest
The authors declare that they have no known competing financial interests or personal relationships that could have appeared to influence the work reported in this paper.
Acknowledgements
This work was supported by the Ministry of Science and Technology of China [Project No. 2017YFC1600402] and the National Natural Science Foundation of China [Project No. 21375094].
Notes and references
- P. Wang, L. Sun, J. Wu, X. Yang, P. Lin and M. Wang, J. Hazard. Mater., 2021, 407, 124388 CrossRef CAS PubMed.
- Z. Wang, X. Xiao, T. Zou, Y. Yang, X. Xing, R. Zhao, Z. Wang and Y. Wang, Nanomater, 2019, 9, 32 CrossRef PubMed.
- X. Q. Yi, Y. F. He, Y. S. Cao, W. X. Shen and Y. Y. Lv, ACS Sens., 2019, 4, 856–864 CrossRef CAS PubMed.
- Y. Xu, Y. Hou, Y. Wang, Y. Wang, T. Li, C. Song, N. Wei and Q. Wang, Ecotoxicol. Environ. Saf., 2019, 168, 356–362 CrossRef CAS PubMed.
- J. Chen, H. Chen, T. Wang, J. Li, J. Wang and X. Lu, Anal. Chem., 2019, 91, 4331–4336 CrossRef CAS PubMed.
- Q. Fan, J. Li, Y. Zhu, Z. Yang, T. Shen, Y. Guo, L. Wang, T. Mei, J. Wang and X. Wang, ACS Appl. Mater. Interfaces, 2020, 12, 4797–4803 CrossRef CAS.
- D. C. Brady, M. S. Crowe, M. L. Turski, G. A. Hobbs, X. Yao, A. Chaikuad, S. Knapp, K. Xiao, S. L. Campbell, D. J. Thiele and C. M. Counter, Nature, 2014, 509, 492–496 CrossRef CAS PubMed.
- X. He, K. Jia, Y. Bai, Z. Chen, Y. Liu, Y. Huang and X. Liu, J. Hazard. Mater., 2021, 405, 124263 CrossRef CAS PubMed.
- R. Cary, S. Unser, I. Monroe, J. Holbrook and L. Sagle, Analyst, 2020, 145, 4950–4956 RSC.
- M. Monier, A. A. H. Bukhari and N. H. Elsayed, Int. J. Biol. Macromol., 2020, 155, 795–804 CrossRef CAS PubMed.
- S. Qiu, Y. Wei, T. Tu, J. Xiang, D. Zhang, Q. Chen, L. Luo and Z. Lin, Food Chem., 2020, 317, 126434 CrossRef CAS PubMed.
- I. M. Moreno, D. González-Weller, V. Gutierrez, M. Marino, A. M. Cameán, A. G. González and A. Hardisson, Microchem. J., 2008, 88, 56–61 CrossRef CAS.
- F. S. Awad, K. M. AbouZied, A. M. Bakry, W. M. Abou El-Maaty, A. M. El-Wakil and M. S. El-Shall, Anal. Chim. Acta, 2020, 1140, 111–121 CrossRef CAS.
- T. Xie, X. Zhong, Z. Liu and C. Xie, Microchim. Acta, 2020, 187, 323 CrossRef CAS PubMed.
- Y. Niu, T. Ding, J. Liu, G. Zhang, L. Tong, X. Cheng, Y. Yang, Z. Chen and B. Tang, Talanta, 2021, 223, 121745 CrossRef CAS PubMed.
- Y. F. Xie, Y. J. Jiang, H. Y. Zou, J. Wang and C. Z. Huang, Talanta, 2020, 220, 121430 CrossRef CAS PubMed.
- V. K. Singh, C. S. Kushwaha and S. K. Shukla, Int. J. Biol. Macromol., 2020, 147, 250–257 CrossRef CAS.
- Z. Li, Y. Zhang, H. Xia, Y. Mu and X. Liu, Chem.
Commun., 2016, 52, 6613–6616 RSC.
- C. Cheng, R. Zhang, J. Wang, Y. Zhang, C. Wen, Y. Tan and M. Yang, Analyst, 2020, 145, 797–804 RSC.
- H. Mehdi, H. Pang, W. Gong, M. K. Dhinakaran, A. Wajahat, X. Kuang and G. Ning, Org. Biomol. Chem., 2016, 14, 5956–5964 RSC.
- L. Liu, J. Zhang, H. Fang, L. Chen and C.-Y. Su, Chem.–Asian J., 2016, 11, 2278–2283 CrossRef CAS.
- P. Liao, H. Fang, J. Zhang, Y. Hu, L. Chen and C.-Y. Su, Eur. J. Inorg. Chem., 2017, 2017, 2580–2584 CrossRef CAS.
- A. Mahmood, W. Xia, N. Mahmood, Q. Wang and R. Zou, Sci. Rep., 2015, 5, 10556 CrossRef PubMed.
- M. X. Guo, L. Yang, Z. W. Jiang, Z. W. Peng and Y. F. Li, Spectrochim. Acta, Part A, 2017, 187, 43–48 CrossRef CAS.
- M. Araújo, S. Díaz-Oltra and B. Escuder, Chem.–Eur. J., 2016, 22, 8676–8684 CrossRef PubMed.
- Q. Wei and S. L. James, Chem. Commun., 2005, 12, 1555–1556 RSC.
- S. Bhowmik, B. N. Ghosh, V. Marjomäki and K. Rissanen, J. Am. Chem. Soc., 2014, 136, 5543–5546 CrossRef CAS.
- Z. S. Qin, W. W. Dong, J. Zhao, Y. P. Wu, Q. Zhang and D. S. Li, Inorg. Chem. Front., 2018, 5, 120–126 RSC.
- W. Miao, L. Zhang, X. Wang, H. Cao, Q. Jin and M. Liu, Chem.–Eur. J., 2013, 19, 3029–3036 CrossRef CAS.
- W. s. Liu, Y. Yang, Q. k. Zhong, Z. p. Xu, J. z. Zhang, B. b. Yao, X. Lian and H. l. Niu, Mater. Chem. Front., 2021, 5, 1932–1941 RSC.
- M. Sokołowska and W. Bal, J. Inorg. Biochem., 2005, 99, 1653–1660 CrossRef PubMed.
- J. Liu, X. Ren, X. Meng, Z. Fang and F. Tang, Nanoscale, 2013, 5, 10022–10028 RSC.
- B. Muthuraj, R. Deshmukh, V. Trivedi and P. K. Iyer, ACS Appl. Mater. Interfaces, 2014, 6, 6562–6569 CrossRef CAS.
- L. Lu, C. Feng, J. Xu, F. Wang, H. Yu, Z. Xu and W. Zhang, Biosens. Bioelectron., 2017, 92, 101–108 CrossRef CAS.
- S. Mu, J. C. Chang and S. T. Lee, Nano Lett., 2008, 8, 104–109 CrossRef.
- Y. Hu, A. Chen, Z. Kong and D. Sun, Molecules, 2019, 24, 4238 CrossRef PubMed.
- X. Ma, S. Lin, Y. Dang, Y. Dai, X. Zhang and F. Xia, Anal. Bioanal. Chem., 2019, 411, 6645–6653 CrossRef CAS PubMed.
- S. Wu, X. Ma, Y. Wang, J. Zhou, X. Li and X. Wang, Spectrochim. Acta, Part A, 2021, 249, 119330 CrossRef CAS.
- C. Wang, L. Lu, W. Ye, O. Zheng, B. Qiu, Z. Lin, L. Guo and G. Chen, Analyst, 2014, 139, 656–659 RSC.
- A. Zhu, Q. Qu, X. Shao, B. Kong and Y. Tian, Angew. Chem., Int. Ed., 2012, 51, 7185–7189 CrossRef CAS.
- A. Salinas-Castillo, M. Ariza-Avidad, C. Pritz, M. Camprubí-Robles, B. Fernández, M. J. Ruedas-Rama, A. Megia-Fernández, A. Lapresta-Fernández, F. Santoyo-Gonzalez, A. Schrott-Fischer and L. F. Capitan-Vallvey, Chem. Commun., 2013, 49, 1103–1105 RSC.
- F. Wang, C. Zhang, Q. Xue, H. Li and Y. Xian, Biosens. Bioelectron., 2017, 95, 21–26 CrossRef CAS PubMed.
- K. Lalitha, V. Sridharan, C. U. Maheswari, P. K. Vemula and S. Nagarajan, Chem. Commun., 2017, 53, 1538–1541 RSC.
- L. Lin, M. Rong, S. Lu, X. Song, Y. Zhong, J. Yan, Y. Wang and X. Chen, Nanoscale, 2015, 7, 1872–1878 RSC.
- F. Shi, S. Cui, H. Liu and S. Pu, Dyes Pigm., 2020, 173, 107914 CrossRef CAS.
Footnote |
† Electronic supplementary information (ESI) available. See DOI: 10.1039/d1ay01716k |
|
This journal is © The Royal Society of Chemistry 2022 |
Click here to see how this site uses Cookies. View our privacy policy here.