DOI:
10.1039/D1CE01261D
(Paper)
CrystEngComm, 2022,
24, 169-181
Four MOFs with isomeric ligands as fluorescent probes for highly selective, sensitive and stable detection of antibiotics in water†
Received
18th September 2021
, Accepted 8th November 2021
First published on 10th November 2021
Abstract
The pollution and damage caused by antibiotics in water have aroused serious concern. Metal–organic frameworks (MOF), which have been hotly investigated as a novel kind of sensing materials, have been tentatively applied to the detection of antibiotics in recent years. In this work, four new Zn(II)/Cd(II) coordination polymers, [Cd(opda)(mbib)(H2O)] (1), [Cd(opda)(pbib)(H2O)] (2), [Cd(ppda)(mbib)] (3), and [Zn2(mpda)2(mbib)2]·2H2O (4), (H2opda = 1,2-phenylenediacetic acid, H2mpda = 1,3-phenylenediacetic acid, H2ppda = 1,4-phenylenediacetic acid, mbib = 1,3-bis(imidazolyl)benzene, pbib = 1,4-bis(imidazolyl)benzene), have been synthesized using bis(imidazole) and phenylenediacetate isomers under hydrothermal conditions. The flexible pda and bib ligands in 1–3 afford 2D (4,4)-connected layers, which are further stacked in an offset fashion with an ABAB sequence to propagate into a 3D supramolecular architecture through strong C–H⋯π and C–H⋯O interactions. In contrast, the flexible cis-conformation mpda and mbib ligands in 4 can be seen as “V”-type building blocks, linking Zn(II) atoms to form a 1D loop chain. The structural diversity of these complexes is mainly attributed to the different coordination modes and isomer conformations of the flexible phenylenediacetic acid and bis(imidazole) ligands. The application of the fluorescence properties of the four complexes for detecting the antibiotics cefixime (CEF) and tetracycline (TEC) and the related mechanisms have been researched. Complexes 1–4 exhibit high quenching percentages in low-concentration aqueous solutions, with values of 94.9%, 97.8%, 95.5%, and 97.2% (toward CEF) and 97.7%, 96.7%, 96.4%, and 97.4% (toward TEC), respectively. Compared with 1–3, complex 4 has a higher quenching percentage, which may be because 4 is a 3D porous supramolecular network. The detection limits of complexes 1–4 have been measured to be 0.278 μM, 0.379 μM, 0.52 μM, and 0.171 μM (for CEF) and 0.384 μM, 0.189 μM, 0.421 μM, and 0.137 μM (for TEC), which surpass/approach those of most of the reported MOF-based fluorescent sensing materials. Systematic analysis of the sensing mechanism reveals that photoinduced electron transfer and the inner filter effect contribute to the realization of the sensing process.
Introduction
Since the advent of penicillin in the 1920s, antibiotic drugs have been widely used in the treatment of various diseases caused by infection by bacteria and pathogenic microorganisms, and have had good effects in improving human resistance to disease.1 Cefixime (CEF) is the first cephalosporin antibiotic that can be taken orally, and is a third-generation antibiotic. Because of its high efficiency and low tendency to cause allergic reactions, CEF is widely used in clinical practice. Tetracycline (TEC) is now widely used in livestock and aquaculture on account of its good water solubility, fast metabolism and wider antibacterial spectrum. After bio-metabolism, antibiotics enter the soil or water via excrement. As a new type of pollutant, the real harm of antibiotic abuse and environmental antibiotic pollution is the increase in the resistance of bacteria. Thus, the safety and sensitive testing of antibiotic drugs have become a hot topic. For instance, Zhang et al. determined the occurrence of antibiotics in urban rivers and their association with antibiotic resistance.2 To combat the threat of antibiotic-resistant bacterial infections to public health, Gupta et al. highlighted multiple nanoparticle-based approaches that could eliminate bacterial infections in their tutorial review, providing crucial insight into the design of elements that play critical roles in creating antimicrobial nanotherapeutics.3 Goswami et al. synthesized an amine-grafted mixed-linker MOF (CSMCRI-2) that exhibits exceptional turn-on and ultra-fast nanomolar detection of sulfadiazine and AMP.4 Goswami et al. designed and built a dual-functionalized, three-dimensional Zn(II) framework (CSMCRI-1) that functions as a unique tetrasensoric fluorescent probe that can selectively detect nitrofurazone antibiotics as well as the nitro-aromatic explosive 2,4,6-trinitrophenol.5 Seal et al. reported the non-penetrated framework CSMCRI-4, which displays extremely selective and ultrafast luminescent sensing of the lethal pesticide 2,6-dichloro-4-nitroaniline (DCNA) and the antibiotic nitrofurazone (NZF) with notable quenching and low limits of detection.6 Zhou et al. investigated a dual-functional platform for the detection and removal of the antibiotic TEC based on a highly stable luminescent zirconium-based MOF.7 To date, many methods for the testing of antibiotic drugs have appeared due to the continuous improvement in detection technologies, such as high-performance liquid chromatography (HPLC),8 liquid chromatography–tandem mass spectrometry (LC-MS),9 Raman spectroscopy (RS),10 ion mobility spectroscopy (IMS),11 field-effect transistors, paper-based microfluidics12 and electrochemical biosensors,13 among others.14 However, these methods require not only expensive equipment, but also rigorous testing conditions.15,16 Among the many technologies for monitoring antibiotics, fluorescent probes have drawn great attention due to their advantages, including high sensitivity, real-time monitoring, and simple equipment. In addition, in the real world, mixtures of antibiotics often exist. As a result, the multiplexed detection of antibiotics in water solution is significant but challenging.
Metal–organic frameworks (MOFs) have been considered by many researchers to be the most promising chemical sensors because of their crystalline nature, extraordinary surface areas, high porosities, finely tunable pore surface properties, potential scalability to industrial scale, outstanding fluorescence properties, and relatively long emission wavelengths, among other advantages.17–26 Most of these fluorescent MOFs detect anions, cations and organic pollutants in water quickly, conveniently and efficiently. For instance, Wu et al. presented a tetraphenylethylene-immobilized MOF as a highly sensitive fluorescent sensor for the detection of Cr2O72− and nitroaromatic explosives,27 Fu et al. presented a water-stable MOF that could be used to capture Cr(VI)-oxyanions via ion exchange with high capacity and selectivity,28 Qin et al. investigated pH-stable Eu- and Tb-organic-frameworks that could detect TNP,29 Liu et al. presented two MOFs, [Co4(μ3-OH)2(L)(BTB)2(H2O)3]·5.6H2O and [Cd3(L)2(BTB)2(μ2- H2O)]·7.4H2O, that can be considered as potential luminescent probes for the detection of ketone molecules,30–32 and Xie et al. demonstrated a stable luminescent MOF as a dual-functional material that could be used to encapsulate Ln3+ ions for white-light emission and to detect nitroaromatic explosives.33 Few reports of the use of Ln-MOFs, d10-MOFs or d10-MOFs/Ln3+ nanostructures as sensing materials for the detection of antibiotics are found in the literature.34–36 Moreover, multiselective antibiotic sensors based on MOFs are rarely reported.37 Thus, the rational synthesis of d10-MOFs for multiselective sensing of antibiotic materials is still challenging.
The rational design and synthesis of d10-MOFs for fluorescence detection are intriguing in the field of fluorescence probes. In our recent work, a series of d10-MOFs based on flexible phenylenediacetate isomers (pda) and different bis(imidazole) ligands exhibited not only the best catalytic activity toward methylene blue (MB) under visible light irradiation, but also the best luminescence properties and sensing behaviors for o-NP (o-nitrophenol) and Cr2O72− in aqueous solution.38–40 We are systematically investigating the fluorescence properties of phenylenediacetate isomer MOF materials with different coordination groups, conformations, and flexibility. In this paper, by employing mixtures of different flexible phenylenediacetate building blocks in the presence of bis(imidazolyl)benzene ligands (mbib/pbib), four 1D/2D coordination polymers 1–4 have been synthesized and structurally characterized. Furthermore, their luminescence properties and sensing behaviors have also been investigated in water solution. The as-synthesized samples of these MOFs could be used directly as CEF and TEC sensors because of their sensitive and specific fluorescence responses. To our knowledge, these are the first fluorescent d10-MOF materials for the detection of the antibiotics CEF and TEC. More interestingly, among these isomer complexes, complex 4 has the best fluorescence quenching effect on CEF and TEC, which may be because compound 4 has a 3D porous supramolecular network. Specifically, the quenching mechanism of the complexes 1–4 to detect CEF and TEC have been investigated in detail. UV-vis experiments showed that the absorption spectra of CEF and TEC had the greatest overlap with the emission spectrum of the as-obtained fluorescent materials (complexes 1–4), which is favorable for the absorption of the emission light. Theoretical calculations confirmed that the LUMO energy levels of CEF and TEC are lower than that of complexes 1–4, making donor–acceptor electron transfer possible. Obviously, the present work provides new materials for the detection of the antibiotics CEF and TEC in aqueous systems, which has potential applications in water-quality monitoring.
Experimental section
Materials and methods
Commercially obtained reagents and solvents were used. The hydrothermal reactions were performed in a 25 mL Teflon-lined stainless-steel autoclave under autogenous pressure. The IR spectra were recorded as KBr pellets using a Nicolet Avatar-360 spectrometer in the range of 4000 to 400 cm−1. Elemental analyses for C, H, and N were carried out using a Flash 2000 elemental analyzer. Thermalgravimetric analyses (TGA) were carried out using an SDT Q600 thermogravimetric analyzer. A platinum pan was used for heating the sample with a heating rate of 10 °C min−1 under a N2 atmosphere. Fluorescence spectra were recorded using a Hitachi F4500 fluorescence spectrophotometer equipped with a xenon lamp at room temperature. Both the excitation and emission band passes were set at 5 nm. Powder X-ray diffraction (PXRD) measurements were performed using a Bruker D8-ADVANCE X-ray diffractometer with Cu Kα radiation (λ = 1.5418 Å). Simulation of the PXRD spectra was carried out using the single-crystal data and the diffraction-crystal module of the Mercury (Hg) program, which is available free of charge via the internet at http://www.iucr.org. The fluorescence lifetime was tested using a FLSP920 transient steady-state fluorometer.
Synthesis of the complexes
[Cd(opda)(mbib)(H2O)] (1).
A mixture of H2opda (0.0210 g, 0.1 mmol), Cd(NO3)2·4H2O (0.0308 g, 0.10 mmol), mbib (0.0194 g, 0.1 mmol) and KOH (5.6 mg, 0.1 mmol) was added to water (12 mL) in a 25 mL Teflon-lined stainless-steel vessel. The mixture was heated at 160 °C for 72 h. After the reaction mixture was slowly cooled to room temperature, colourless block crystals of 1 were obtained (yield: 45% based on Cd). Elemental analysis calcd.(%) for C22H20CdN4O5: C 49.59, H 3.78, N 10.51; found (%): C 49.38, H 3.59, N 10.35. IR(KBr pellet, cm−1): 3455 m, 3141 w, 2379 w, 1608 s, 1577 s, 1519 m, 1396 m, 1352 s, 1112 m, 950 m, 853 m, 654 s.
[Cd(opda)(pbib)(H2O)] (2).
Complex 2 was synthesized by a procedure similar to that of 1, except pbib replaced mbib. Colourless block crystals of 2 were obtained (yield: 40% based on Cd). Elemental analysis calcd. (%) for C22H20CdN4O5: C 49.59, H 3.78, N 10.51; found (%): C 49.43, H 3.74, N 10.38. IR (KBr pellet, cm−1): 3451 m, 3128 m, 2379 w, 1616 s, 1526 m, 1367 s, 1283 m, 1237 m, 1089 s, 951 m, 837 w, 712 s.
[Cd(ppda)(mbib)] (3).
Complex 3 was synthesized by a procedure similar to that of 1, except ppda replaced opda. Colourless block crystals of 3 were obtained (yield: 46% based on Cd). Elemental analysis calcd. (%) for C22H18CdN4O4: C 51.33, H 3.52, N 10.88; found (%): C 51.09, H 3.34, N 10.71. IR (KBr pellet, cm−1): 3123 m, 1591 s, 1532 s, 1456 m, 1376 (s), 1291 m, 1272 m, 1089 s, 950 m, 839 m, 719 s.
[Zn2(mpda)2(mbib)2]·2H2O (4).
Complex 4 was synthesized by a procedure similar to that of 1, except H2mpda replaced H2opda, and Zn(NO3)2 replaced Cd(NO3)2 (yield: 40% based on Zn). Elemental analysis calcd. (%) for C44H40Zn2N8O10: C 54.39, H 4.15, N 11.53; found (%): C 54.21, H 3.98, N 11.37. IR (cm−1): 3506 m, 3143 m, 1582 s, 1373 s, 1252 m, 1142 m, 1066 m, 969 m, 751 s, 668 m, 541 m.
Crystal structural determination
X-ray single-crystal diffraction data for two complexes were collected using a Bruker Smart 1000 CCD area-detector diffractometer with Mo-Kα radiation (λ = 0.71073 Å) in ω scan mode. The crystal structure was solved by direct methods using SHELXS-2014 and least-squares refined with SHELXL-2014 using anisotropic thermal displacement parameters for all non-hydrogen atoms.41,42 Metal atoms were located from the E-maps, and the other non-hydrogen atoms were located in successive difference Fourier syntheses and refined with anisotropic thermal parameters on F2. Further details of the structural analysis are summarized in Table 1. Selected bond distances and bond angles are listed in Table S1.†
Table 1 Crystallographic data and details of diffraction experiments for complexes 1–4
Complex |
1
|
2
|
3
|
4
|
R
1 = Σ||Fo| – |Fc||/Σ|Fo|, wR2 = [Σw(Fo2 – Fc2)2/Σw(Fo2)2]1/2.
|
Formula |
C22H20CdN4O5 |
C22H20CdN4O5 |
C22H18CdN4O4 |
C44H40Zn2N8O10 |
Mass |
532.82 |
532.82 |
514.80 |
971.58 |
Crystal system |
Orthorhombic |
Triclinic |
Triclinic |
Monoclinic |
Space group |
Pnna
|
P![[1 with combining macron]](https://www.rsc.org/images/entities/char_0031_0304.gif) |
P![[1 with combining macron]](https://www.rsc.org/images/entities/char_0031_0304.gif) |
P21/n |
a (Å) |
24.4715(7) |
8.7652(19) |
9.907(5) |
7.9711(4) |
b (Å) |
8.9399(3) |
10.148(2) |
10.860(5) |
38.293(2) |
c (Å) |
9.9133(3) |
12.958(3) |
11.410(6) |
13.9006(7) |
α (°) |
90 |
73.895(3) |
114.119(6) |
90 |
β (°) |
90 |
77.424(3) |
107.767(7) |
96.4690(10) |
γ (°) |
90 |
75.347(4) |
97.924(7) |
90 |
V (Å3) |
2168.76(12) |
1057.7(4) |
1017.1(9) |
4215.9(4) |
Z
|
4 |
2 |
2 |
4 |
D
c (g cm−3) |
1.632 |
1.673 |
1.681 |
1.531 |
μ (mm−1) |
1.049 |
1.075 |
1.111 |
1.208 |
R
int
|
0.0431 |
0.0154 |
0.0103 |
0.0291 |
Goof |
1.092 |
1.187 |
1.077 |
1.125 |
R
1
/wR2 [I > 2σ(I)] |
0.0289/0.0613 |
0.0252/0.0667 |
0.0330/0.0976 |
0.0423/0.0894 |
R
1
/wR2 (all data) |
0.0456/0.0662 |
0.0306/0.0306 |
0.0400/0.1094 |
0.0648/0.1008 |
Fluorescent probe detection experiment
First, finely ground samples of crystalline complexes 1–4 (3 mg each) were placed in 10 mL water and treated by ultrasonication for 1 h. The aqueous solutions of complexes 1–4 were allowed to stand for 1 day to form a stable suspension. Secondly, six common antibiotic stock solutions (roxithromycin (ROX), tetracycline (TEC), chloramphenicol (CHL), gentanicin sulfate (GEN), penicilin sodium (PEN), lincomycin hydrochloride (LIN)) were prepared using water and two antibiotic stock solutions (cefixime (CEF) azithromycin (AZI)) were prepared using ethanol/water (1
:
10) at a concentration of 1 mM, respectively. The prepared aqueous solutions of complexes 1–4 were used for the initial luminescence spectra and fluorescence experiments. The antibiotic was added to the prepared aqueous solutions of complexes 1–4 each time. We obtained the relationship between the quenching rate and the quantity of CEF/TEC solution added. The suspension was stirred at a constant rate during the experiment to maintain homogeneity.
Results and discussion
Structural descriptions
Structure of [Cd(opda)(mbib)(H2O)] (1) and [Cd(opda)(pbib)(H2O)] (2).
The structures of 1 and 2 are very similar, and hence can be treated together.
Single-crystal X-ray structure analysis reveals that the asymmetric units of 1 and 2 contain one Cd(II) atom, one fully deprotonated opda2− ligand and one bib ligand (mbib in 1, pbib in 2). As shown in Fig. 1a and Fig. S2a,† each Cd(II) atom is seven-coordinated by two N-atom donors from two bib ligands, five O atoms from two different pda2− ligands and one water molecule.
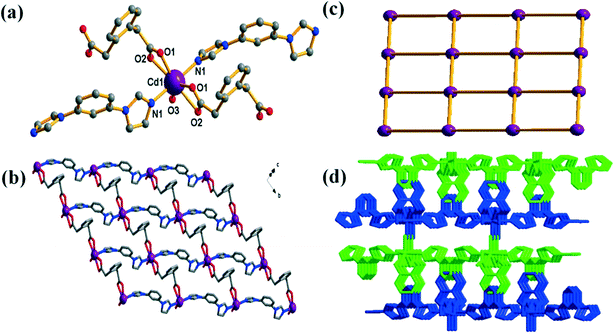 |
| Fig. 1 (a) Coordination environment of the Cd(II) atom in complex 1. All hydrogen atoms are omitted for clarity. Symmetry code: #1: x, −y − 1/2, −z + 1/2; #2: x, −y − 3/2, −z + 3/2; #3: x, −y + 1/2, −z + 1/2. (b) and (c) Ball-and-stick view of the 2D (4,4) layer in 1. (d) View of the 3D network of 1 formed by the 2D layers in an offset stacking fashion. | |
In 1 and 2, the opda ligand adopts an asymmetric trans-conformation with the two carboxylate groups, with angles of 114.26° and 114.26° about the two –CH2– groups for 1, and of 113.82° and 114.33° for 2. The bib ligands exhibit a planar conformation with dihedral angles of 41.96 and 42.23° between the benzene core and imidazole arms for 1, and of 33.19 and 37.07° for 2 (Fig. S1†). The opda ligand has a trans-conformation, and the two carboxy groups adopt a μ1–η1:η1 coordination mode, and thus, the ligand can be viewed as a linear building block (Scheme 1a and b). Both the opda2− and mbib/pbib linkers connect the Cd(II) centers into a 2D (4,4) net (Fig. 1b and c, S2b and S2c†). These layers are stacked in an offset fashion with an ABAB sequence to result in a 3D architecture through strong O–H⋯O hydrogen bonds and C–H⋯π interactions (Fig. 1d, S2d†).
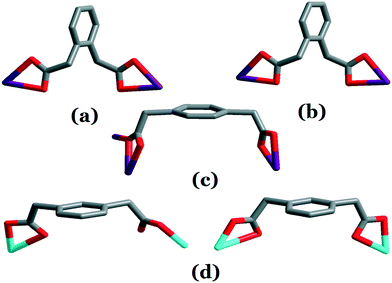 |
| Scheme 1 Coordination modes of the carboxylate ligands in this work. | |
Structure of [Cd(ppda)(mbib)] (3).
Single crystal X-ray structure analysis reveals that the asymmetric unit of 3 contains one Cd(II) atom, one fully deprotonated ppda2− ligand and one mbib ligand. As shown in Fig. 2a, each Cd(II) atom is seven-coordinated by two N-atom donors from two mbib ligands and five O atoms from three different ppda2− ligands. In 3, two such Cd(II) centers are bridged by a pair of carboxylate groups with the η–O,O′–μ–O,O binding mode, furnishing a centrosymmetric bimetallic unit with a Cd⋯Cd separation of 3.871 Å.
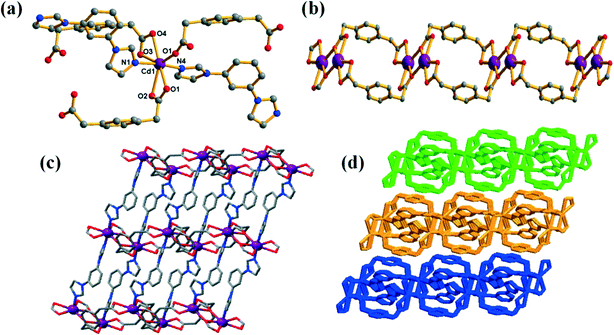 |
| Fig. 2 (a) Coordination environments of the Cd(II) atoms in complex 3. All hydrogen atoms are omitted for clarity. Symmetry code: #1: −x + 2, −y + 3, −z; #2: −x + 3, −y + 3, −z; #3: x + 1, y + 1, z; #4: x − 1, y − 1, z. (b) Ball-and-stick view of the 1D wavelike double-chain in 3. (c) View of 2D extended layer in 3. (d) View of 3D supramolecular architecture in 3. | |
In 3, the ppda ligand adopts an asymmetric cis-conformation with the two carboxylate groups, with angles of 110.75° and 109.23° about the two –CH2– groups. The mbib ligand exhibits a planar conformation with dihedral angles of 26.26 and 26.26° between the benzene core and imidazole arms (Fig. S1†). In 3, the ppda ligand has a cis-conformation, and the two carboxy groups adopt μ1–η1:η1, μ2–η2:η1 coordination modes; thus, the ligand acts as a “V”-type building block (Scheme 1c). Thus, the bimetallic nodes are linked by the μ5-pda ligands to generate a 1D wavelike double-chain (Fig. 2b). The parallel 1D wavelike double-chains are cross-linked by pairs of mbib ligands into 2D (4,4)-connected double-networks (Fig. 2c). As can be seen from the crystal packing diagram of 3, the adjacent 2D polymeric ribbon motifs are bound together by strong intermolecular hydrogen bonds and C⋯π interactions to create a 3D supramolecular architecture (Fig. 2d).
Structure of [Zn2(mpda)2(mbib)2]·2H2O (4).
Single-crystal X-ray diffraction analysis reveals that complex 4 crystallizes in the monoclinic system, P21/n space group. The asymmetric unit of 4 consists of two Zn(II) ions, two deprotonated mpda2− ligands, two mbib ligands, and two lattice water molecules. As shown in Fig. 3a, the central Zn(II) atoms have six-coordinated distorted octahedral and five-coordinated tetrahedral coordination geometry.
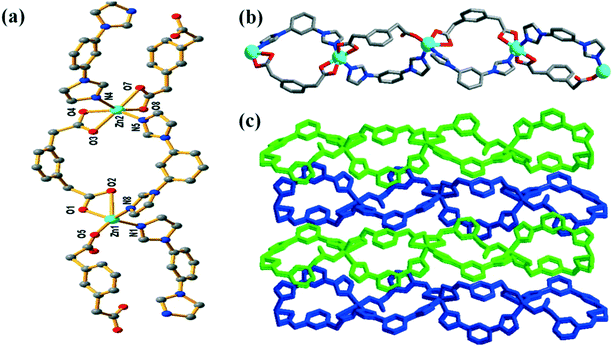 |
| Fig. 3 (a) Coordination environments of the Zn(II) atoms in complex 4. All hydrogen atoms are omitted for clarity. Symmetry code: #1: −x + 1/2, y − 1/2, −z + 1/2; #2 −x + 1/2, y + 1/2, −z + 1/2. (b) Ball-and-stick view of the 1D loop chain in 4. (c) View of the 3D supramolecular architecture in 4. | |
In 4, the mbib ligands exhibit a planar conformation with dihedral angles of 37.83 and 38.04° between the benzene core and imidazole arms, and thus acts as a “V”-type building block (Fig. S1†). In 1, the opda2− ligand has a trans-conformation with two carboxy groups located at two sides of the benzene ring plane, and two carboxy groups adopt the μ1–η1:η1 chelate coordination mode; thus, the ligand can be viewed as a linear building block (Scheme 1d). In contrast, the mpda ligand in 4 shows a cis conformation with the two carboxy groups on the same side of the benzene ring plane; the carboxy groups adopt the μ1–η1:η0 and μ1–η1:η1 coordination modes, and the ligand thus acts as a “V”-type building block (Scheme 1d). Thus, the two kinds of “V”-type building blocks link the Zn(II) atoms, giving rise to a 1D loop containing a polymeric ribbon running in the a direction (Fig. 3b). Adjacent 1D polymeric ribbon motifs are bound together by strong intermolecular hydrogen bonds to create a 2D supramolecular layer. In addition, an extended 3D porous supramolecular network is formed through π⋯π interactions between benzene rings of the mbib and mpda ligands with a face-to-face orientation (Fig. 3c). The surface area, pore size and pore volume (Vt) of complex 4 were assessed using Brunauer–Emmett–Teller (BET) analysis. Fig. S3† shows that complex 4 had a typical type IV isotherm with a H4 hysteresis loop in the P/P0 range of 0.24–1, indicating that it had a aggregates of particles forming slit shaped pores with uniform size.43 As listed in Table S2,† the calculated BET surface area and pore size of complex 4 were 38.76 m2 g−1 and 2.68 nm, respectively.
Synthetic chemistry and structural diversity
In total, four Zn(II)/Cd(II) coordination polymers containing a bis(imidazolyl) benzene (bib) and a phenylenediacetate (pda) ligand have been prepared and structurally characterised. The compounds contain one of the three structural isomers of bib (obib = 1,2-bis(imidazolyl)benzene, mbib = 1,3-bis(imidazolyl)benzene, pbib = 1,4-bis(imidazolyl)benzene) and one of the three structural isomers of pda (opda = 1,2-phenylenediacetate, mpda = 1,3-phenylenediacetate, ppda = 1,4-phenylenediacetate). In all four crystal structures, the cadmium(II) center has a higher coordination number, reflecting the larger size of cadmium(II) compared to that of zinc(II). The mpda and ppda ligands both show examples of both cis and trans geometries (complex 3 and 4). In contrast, opda only shows trans conformations, which is likely to be a consequence of the cis conformations being sterically disfavoured (complex 1 and 2). The two imidazole rings are at opposite positions relative to the benzene core (Fig. S1†), and the Cd⋯Cd separation through the pbib ligand in 2 is longer than that through the mbib ligand in 1 (13.349 Å for 1, 14.973 Å for 2). For the three coordination polymers (1–3), all the pda and bib ligands act as bridging building blocks extending a 1D polymer chain containing Cd-pda into 2D (4,4)-connected network structures, which are further stacked in an offset fashion with an ABAB sequence to propagate into a 3D supramolecular architecture through strong C–H⋯π and C–H⋯O interactions. In contrast, the mpda and mbib ligands in 4 can be seen as “V”-type building blocks, linking Zn(II) atoms to form a 1D loop chain. In this sense, the auxiliary N-donor ligands and coordination modes of the metal ions and multicarboxylate ligands play an important role in governing the coordination clusters and the final polymer structures in these assembled systems.
PXRD results and thermal analysis of complexes 1–4
In order to confirm the phase purity of the bulk materials, the powder X-ray diffraction (PXRD) patterns of complexes 1–4 were recorded at room temperature. The PXRD of samples of complexes 1–4 after water stability testing and after being recycled for antibiotic detection were also recorded. As shown in Fig. S4,† the peak positions of the experimental PXRD and computer-simulated patterns are in agreement with each other, which confirm its phase purity and show the stability of the compounds after antibiotic detection. The differences in the intensity of some of the diffraction peaks may be attributed to the preferred orientation of the crystalline powder samples.
Thermal analysis of complexes 1–4
To examine the stability of complexes 1–4, a thermalgravimetric analysis (TGA) experiment was performed by heating the corresponding samples from room temperature to 800 °C with a heating rate of 10 °C min−1 under a nitrogen atmosphere, as shown in Fig. S5.† The TGA curves exhibit a weight loss of 3.32% (25–300 °C) for 1, 3.33% (25–300 °C) for 2, and 3.65% (25–300 °C) for 4, corresponding to the release of coordinated water molecules and/or free water molecules (calcd. 3.38%, 3.38%, 3.70%). For 3, because there are no water molecules in the framework, there is a plateau region ranging from 20 °C to about 300 °C, followed by a sudden decrease in weight. For complexes 1–4 the final residues of 24.53%, 24.58%, 25.35%, and 17.23% are close to the calculated values of 24.10%, 24.10%, 24.94%, and 16.76% based on CdO/ZnO.
Luminescence properties
Since complexes 1–4 are constructed with a d10-electron-configured Zn2+/Cd2+ ion and fluorescent multi-carboxyl and bis(imidazole) organic ligands containing aromatic or conjugated π moieties, it is highly possible that they exhibit photoactivity. To examine the luminescence properties of these d10 metal complexes, the luminescence spectra of complexes 1–4 and the free opda, mpda, ppda, mbib and pbib ligands were measured at room temperature. As shown in Fig. 4, the free opda, mpda and ppda ligands display main emission peaks at 397 nm (λex = 330 nm), 488 nm (λex = 376 nm) and 479 nm (λex = 384 nm), respectively. The mbib and pbib ligands display main emission bands at 402 nm (λex = 350 nm) and 338 nm (λex = 301 nm). In contrast, the emission peaks of complexes 1 [312 nm (λex = 270 nm)], 2 [369 nm (λex = 302 nm)], 3 [396 nm (λex = 333 nm)], and 4 [329 nm (λex = 277 nm)] are close to those of the pda ligands, and their waveforms are similar. This indicates that the photoluminescence of 1–4 could be mainly attributed to pda-ligand-centred electronic excitation perturbed by the Zn2+/Cd2+ ion and other components. These shifts of the emission bands can be attributed to the deprotonation effect of pda and the coordination interactions of the organic ligands toward the Zn2+/Cd2+ ions, as well as the C–H⋯π interactions in the networks of the complexes.43 The emission intensities of 1–4 are much stronger than that of a free pda ligand; that is, the formation of complexes enhances the fluorescence of the ligand. This phenomenon is a typical example of aggregation-induced emission (AIE) and may be explained by the coordination to the metal ions in the complexes restricting the deformation of the ligand and the induced nonradiative relaxation.44,45
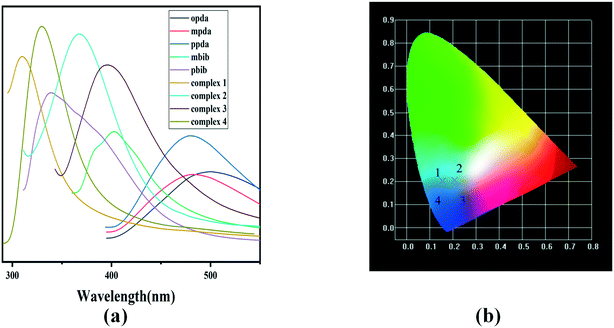 |
| Fig. 4 (a) Solid-state emission spectra of 1–4, and free opda, mpda, ppda, mbib and pbib ligands at room temperature, and (b) CIE-1931 chromaticity diagram of 1–4. | |
Sensing of CEF and TEC
Because of the excellent water stability and strong fluorescence of the four complexes 1–4, their application in sensing/detecting the antibiotics CEF and TEC in water systems were explored. First, the sensing/detecting ability of the four complexes toward different antibiotics were investigated by adding aqueous solutions of CEF, ROX, TEC, CHL, AZI, GEN, PEN, or LIN to suspensions of the four complexes (Fig. 5a, S6a†). By studying the effects of the eight antibiotics on the fluorescence intensity, it was found that CEF and TEC had a very significant luminescence quenching effect. Other antibiotics led to only slight changes in the fluorescence intensity of the suspensions of complexes 1–4. Compared to complexes 1–3, complex 4 has a higher quenching percentage, which may be attributed to the higher adsorption capacity of complex 4 with its 3D porous structure (with 18.87 × 20.21 Å windows). The emission positions of the four MOF materials are similar, and they show good selective detection of CEF and TEC in aqueous solution; thus, the quenching mechanism may be the same.
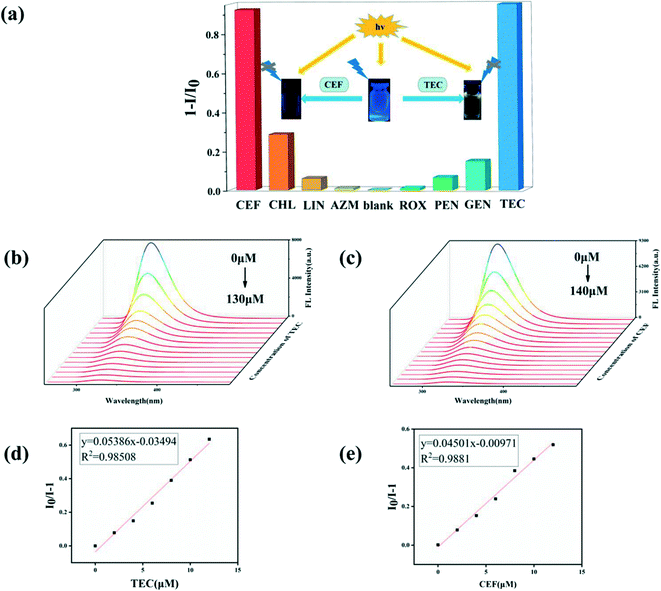 |
| Fig. 5 (a) Luminescence intensity of complex 4 (2 mL, prepared solution) in aqueous solutions with different antibiotics (0.001 M). (b) Concentration-dependent luminescence quenching of a suspension of complex 4 in water by TEC (excited at 252 nm). (c) Concentration-dependent luminescence quenching of a suspension of complex 4 in water by CEF (excited at 252 nm). (d) Stern–Volmer plot of a suspension of complex 2 quenched by TEC. (e) Stern–Volmer plot of a suspension of complex 2 quenched by CEF. | |
In addition, competitive fluorescence titration experiments were conducted in which the emission intensity of complexes 1–4 were consecutively recorded upon the gradual addition of CEF or TEC solution into a uniform aqueous suspension of the complex containing competing antibiotics. 10 μL of an interfering antibiotic (or a mixture of interfering antibiotics) was first added to a suspension of complex 1–4, and the I/I0 value decreased slightly; subsequently, 0.02 μM of CEF or TEC was added, and the I/I0 value decreased dramatically, indicating that other antibiotics did not interfere with the detection of CEF or TEC (Fig. 6a and b, S8a and S8b†). Therefore, complexes 1–4 have high sensitivity, selectivity, and resistance to interference from other coexisting antibiotics in detecting CEF or TEC in aqueous solution. More noteworthily, the fluorescence intensity of the suspensions of complexes 1–4 decreased significantly in the first 10 seconds after CEF or TEC was added and then maintained an almost constant value, suggesting that the CEF- or TEC-induced fluorescence quenching process is very fast (Fig. S9†). When cefixime was added in the presence of other analytes, an obvious quenching effect on the fluorescence of the complex was also observed, and the fluorescence maintained a stable value (Fig. S8a and S8b†). Moreover, the present fluorescent probe also exhibited good cycling stability. After five cycles, the quenching efficiency is decreased very little (see Fig. 6c and d, S8c and S8d†). These results indicate that complexes 1–4 can serve as sensitive sensors for the selective and rapid detection of CEF and TEC in water systems.
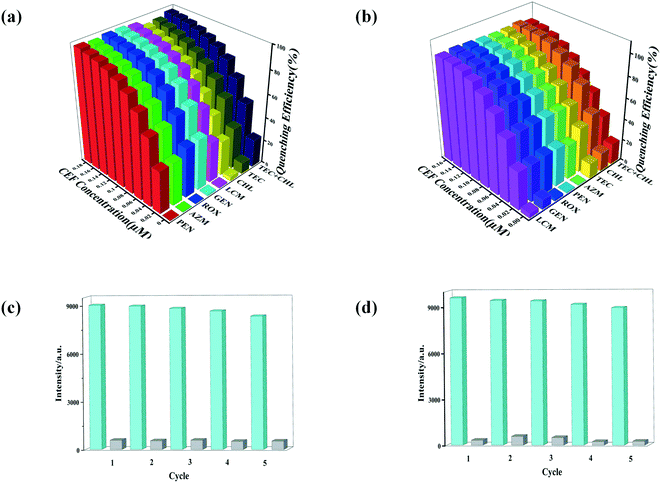 |
| Fig. 6 Luminescence quenching efficiency in an aqueous solution of complex 4 with 1 mM antibiotic upon adding different concentrations of CEF (a) and TEC (b). Cycle stability of complex 4 as a fluorescent probe for the detection of CEF (c) and TEC (d). | |
Furthermore, we explored the quantitative detection ability of complexes 1–4 toward CEF and TEC. Concentration-based quenching experiments were conducted by documenting the decrease in the emission intensity of suspensions of complexes 1–4 upon the addition of CEF or TEC, with incremental concentrations in the range of 2–160 μM. The titration curves show that the emission intensities of complexes 1–4 decrease with increasing TEC or CEF concentration (Fig. 5b and c, S7b and S7c†). The fluorescence quenching efficiency was then quantitatively evaluated using the Stern–Volmer equation, I0/I = Ksv [M] + 1, where I0 and I are the maximum luminescence intensities before and after the addition of the probe, [M] is the molar concentration of the probe solution, and Ksv is the quenching constant (M−1).46–50 On the basis of the Ksv values, the limit of detection (LOD) of complexes 1–4 toward CEF and TEC could be calculated using the formula 3σ/KSV, where σ is the standard deviation for three repeated luminescence measurements. Based on the luminescence intensities for complexes 1–4, the values of Ksv, R2, and LOD were determined and are listed in Table 2. Detailed SV (Stern–Volmer) equation analysis further reveals that there exist near-linear correlations between the quenching efficiency and the amount of antibiotics, indicating that either a static or dynamic mechanism exists in the quenching process (Fig. 5d and e, S7d and S7e†). As shown in Table 3, the sensitivity in this work is superior or similar to those of most of the reported fluorescence probes for CEF/TEC, and meets the standards of the World Health Organization (WHO) and Occupational Safety and Health Administration (OSHA) for the maximum allowable level of antibiotics in drinking water.57 To the best of our knowledge, no single d10-MOF material based fluorescence quenching method has been found for the detection of the antibiotics CEF and TEC. This result demonstrates that these four complexes can be highly effective and selective luminescent sensors for CEF and TEC, with improved or comparable performance to those of the reported d10-MOF probes.
Table 2
K
SV, R2, and LOD of complexes 1–4 toward CEF and TEC at room temperature
Antibiotic |
CEF |
TEC |
Complex |
1
|
2
|
3
|
4
|
1
|
2
|
3
|
4
|
K
SV (105 L mo−1) |
2.932 |
3.683 |
2.282 |
4.501 |
2.127 |
7.381 |
2.906 |
5.386 |
R
2
|
0.9857 |
0.9788 |
0.9971 |
0.9881 |
0.9791 |
0.9673 |
0.9977 |
0.9850 |
LOD (×10−7 M) |
2.78 |
3.79 |
5.3 |
1.71 |
3.84 |
1.89 |
4.2 |
1.37 |
LOD (ppb) |
141 |
192.3 |
269 |
86.8 |
170 |
84 |
186.6 |
60.9 |
Table 3 Comparison of luminescent materials for sensing the antibiotics CEF and TEC
Material |
Objective |
K
SV
|
LOD (ppb) |
Ref. |
Zn-PDC/Tb3+ |
CEF |
1.1 × 105 L mol−1 |
0.14 μM (72 ppb) |
51
|
Cd-MOF/Tb3+ |
CEF |
8.7 × 105 |
0.03 μM (26.7 nM) |
52
|
MIP@TbMOF-76 |
CEF |
|
0.05 μM (48.5 nM) |
53
|
[Zn3(L)2(1,4-bimb)3]n |
TEC |
1.98 × 105 |
0.15 μM |
54
|
In-sbdc |
TEC |
3.11 × 105 |
0.28 μM |
55
|
PCN-128Y |
TEC |
9.84 × 105 |
0.03 μM (30 nM) |
56
|
Fluorescence quenching mechanisms of CEF and TEC
Usually, photoinduced electron transfer (PET) and the inner filter effect (IFE) can cause the fluorescence quenching behavior of fluorescent MOF materials. When the fluorescent material bears a higher LUMO energy level than the analyte, electron transfer from the fluorescent material to the analyte occurs, which is considered as the PET effect causing the fluorescence quenching.58Fig. 7 lists the HOMO and LUMO energy levels of the various antibiotics and complexes 1–4 calculated according to the density functional theory (DFT) method.59 Compared with those of other antibiotics, the LUMO energy levels of CEF, CHL, PEN and TEC are lower than those of complexes 1–4. Electron transfer between the complex (donor) and CEF, CHL, PEN and TEC (acceptor) occurs, which prevents the excitation electron from returning to the ground state. This leads to different fluorescence quenching effects. However, the order of the fluorescence quenching effects is not in accordance with the LUMO energy levels of the antibiotics. In particular, CHL has the lowest LUMO energy level, but does not display the highest fluorescence quenching effect, implying that the PET effect is not the only reason for the fluorescence quenching in the current system.
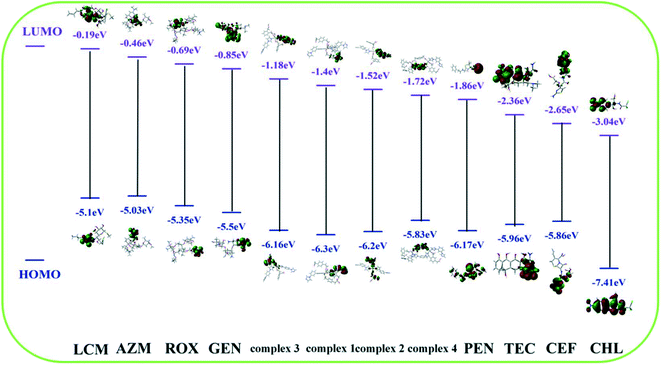 |
| Fig. 7 HOMO (π) and LUMO (π*) energy levels of complexes 1–4 and eight antibiotics. | |
When the excitation and/or emission light of the fluorescent material are absorbed by the analyte, the fluorescence IFE occurs.60–64Fig. 8 displays the absorption spectra of the tested antibiotics and the emission spectra of complexes 1–4 in aqueous solution. It is clearly found that the absorption peak of CEF and TEC located in the region of 275–320 nm overlaps well with the emission peak of complexes 1–4. This indicates that CEF/TEC can strongly absorb the light emitted by complexes 1–4. As a result, the luminescence of complexes 1–4 is highly sensitively quenched. The above facts imply that the IFE occurs between complexes 1–4 and CEF/TEC. Compared to those of complexes 1–3, the emission spectrum of complex 4 has greater overlap with the UV-vis absorption of CEF/TEC; this may be attributed to the higher adsorption capacity of complex 4 with a 3D porous structure. Obviously, PET and the IFE exist simultaneously between complexes 1–4 and CEF/TEC, which leads to high sensitivity and selectivity for the detection of CEF/TEC.
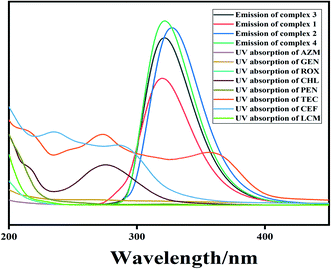 |
| Fig. 8 The UV absorption spectra of various antibiotics (0.1 mM) and the emission spectra of complexes 1–4 in aqueous solution. | |
As the fluorescence lifetime of complexes 1–4 remained unchanged with increasing TEC concentration, the sensing process should be a static quenching process rather than a dynamic quenching process, which is consistent with reports in the literature65 (Table S3†).
Conclusion
In summary, four new coordination polymers, 1–4, have been successfully synthesized under hydrothermal conditions via the reaction of Zn(II)/Cd(II) salt and bis(imidazole) ligands in the presence of different flexible phenylenediacetate isomers. For the three coordination polymers 1–3, all the pda and bib ligands act as bridging building blocks extending a 1D polymer chain containing (Cd-pda) into 2D (4,4)-connected network structures, which are further stacked in an offset fashion with an ABAB sequence to propagate into a 3D supramolecular architecture. The ppda and mbib ligands in 4 can be seen as “V”-type building blocks, linking Zn(II) atoms to form a 1D loop chain. In this sense, the auxiliary N-donor ligands and coordination modes of the metal ions and multicarboxylate ligands play an important role in governing the coordination clusters and final polymer structures in these assembled systems. The four complexes have been shown to be excellent discriminative probes for the highly selective and sensitive detection of the antibiotics CEF and TEC based on their sensitive fluorescence quenching. The diversities of the structures of the four complexes have some effects on their fluorescence properties to accelerate the quenching rate of the antibiotics CEF/TEC, especially for complex 4. These results indicate that the as-synthesized complexes 1–4 are favorable materials for simultaneous detection of the antibiotics CEF and TEC in water, and represent potential sensors for monitoring water quality.
Funding
This work was financially supported by the Natural Science Foundation of Yulin (CXY-2020-065), the Entrepreneurship and Innovation Training Program for College Students of Yan 'an University (D2020034), the Shaanxi University Students Entrepreneurship and Innovation Training Program (S201910719007), and the Graduate Education Innovation Project of Yan 'an University (YCX2021104).
Conflicts of interest
There are no conflicts to declare.
References
- T. P. Van Boeckel, S. Gandra, A. Ashok, Q. Caudron, B. T. Grenfell, S. A. Levin and R. Laxminarayan, Global antibiotic consumption 2000 to 2010: an analysis of national pharmaceutical sales data, Lancet Infect. Dis., 2014, 14, 742–750 CrossRef PubMed.
- Q. Zhang, A. Jia, Y. Wan, H. Liu, K. Wang, H. Peng, Z. Dong and J. Hu, Occurrences of three classes of antibiotics in a natural river basin: association with antibiotic-resistant escherichiacoli, Environ. Sci. Technol., 2014, 48, 14317–14325 CrossRef CAS.
- A. Gupta, S. Mumtaz, C. H. Li, I. Hussain and V. M. Rotello, Combatting antibiotic-resistant bacteria using nanomaterials, Chem. Soc. Rev., 2019, 48, 415–427 RSC.
- R. Goswami, S. C. Mandal, N. Seal, B. Pathak and S. Neogi, Antibiotic-Triggered Reversible Luminescence Switching in Amine Grafted Mixed-Linker MOF: Exceptional Turn-On and Ultra-Fast Nanomolar Detection of Sulfadiazine and AMP with Molecular Keypad-Lock Function, J. Mater. Chem. A, 2019, 7, 19471 RSC.
- R. Goswami, S. C. Mandal, B. Pathak and S. Neogi, Guest-Induced Ultrasensitive Detection of Multiple Toxic Organics and Fe3+ Ions in a Strategically Designed and Regenerative Smart Fluorescent Metal−Organic Framework, ACS Appl. Mater. Interfaces, 2019, 11, 9042 CrossRef CAS.
- N. Seal, R. Goswami, M. Singh, R. S. Pillai and S. Neogi, Ultralight Charged MOF as Fluoro-Switchable Monitor for Assorted Organo Toxins: Size-Exclusive Dye Scrubbing and Anticounterfeiting Application via Tb3+ Sensitization, Inorg. Chem. Front., 2021, 8, 296 RSC.
- Y. Zhou, Q. Yang, D. Zhang, N. Gan, Q. Li and J. Cuan, Detection and removal of antibiotic tetracycline in water with a highly stable luminescent MOF, Sens. Actuators, B, 2018, 262, 137–143 CrossRef CAS.
- P. Moudgil, J. S. Bedi, R. S. Aulakh, J. P. S. Gill and A. Kumar, Antibiotic residues and mycotoxins in raw milk in Punjab (India): a rising concern for food safety, Food Anal. Methods, 2019, 56, 5146–5151 CAS.
- M. Gbylik-Sikorska, A. Gajda, E. Nowacka-Kozak and A. Posyniak, The “force” of cloxacillin residue will be with you in various dairy products – the last experimental evidence, Food Control, 2021, 121, 107628 CrossRef CAS.
- Y. Wu, W. Yu, B. Yang and P. Li, Self-assembled two-dimensional gold nanoparticle film for sensitive nontargeted analysis of food additives with surface-enhanced Raman spectroscopy, Analyst, 2018, 143, 2363–2368 RSC.
- M. McCullagh, K. Giles, K. Richardson, S. Stead and M. Palmer, Investigations into the performance of travelling wave enabled conventional and cyclic ion mobility systems to characterise protomers of fluoroquinolone antibiotic residues, Rapid Commun. Mass Spectrom., 2019, 33, 11–21 CrossRef CAS PubMed.
- X. Y. Chen, Y. Liu, X. Fang, Z. Li, H. H. Pu, J. B. Chang, J. H. Chen and S. Mao, Ultratrace antibiotic sensing using aptamer/graphene-based field-effect transistors, Biosens. Bioelectron., 2019, 126, 664–671 CrossRef CAS.
- A. Nilghaz and X. Lu, Development of paper-based microfluidic device for the determination of nitrite in meat, Anal. Chim. Acta, 2019, 1046, 163 CrossRef CAS.
- T. Wang, H. Yin, Y. Zhang, L. Wang, Y. Du, Y. Zhuge and S. Ai, Electrochemical aptasensor for ampicillin detection based on the protective effect of aptamer-antibiotic conjugate towards DpnII and Exo III digestion, Talanta, 2019, 197, 42–48 CrossRef CAS PubMed.
- K. Daware, R. Shinde, R. S. Kalubarme, M. Kasture, A. Pandey, C. Terashima and S. W. Gosavi, Development of optical sensing probe for Hg (II) ions detection in ground water using Au, Hexanedithiol and Rhodamine B nanocomposite system, Sens. Actuators, B, 2018, 265, 547–555 CrossRef CAS.
- C. H. Chen, X. S. Wang, L. Li, Y. B. Huang and R. Cao, Highly selective sensing of Fe3+ by an anionic metalorganic framework containing uncoordinated nitrogen and carboxylate oxygen sites, Dalton Trans., 2018, 47, 3452–3458 RSC.
- S. S. Zhao, J. Yang, Y. Y. Liu and J. F. Ma, Fluorescent aromatic tag-functionalized MOFs for highly selective sensing of metal ions and small organic molecules, Inorg. Chem., 2016, 55, 2261–2273 CrossRef CAS.
- Z. S. Qin, W. W. Dong, J. Zhao, Y. P. Wu, Q. Zhang and D. S. Li, A water-stable Tb(III)-based metal–organic gel (MOG) for detection of antibiotics and explosives, Inorg. Chem. Front., 2018, 5, 120–126 RSC.
- X. Liu, D. Huang, C. Lai, G. Zeng, L. Qin, H. Wang, H. Yi, B. Li, S. Liu, M. Zhang, R. Deng, Y. Fu, L. Li, W. Xue and S. Chen, Recent advances in covalent organic frameworks (COFs) as a smart sensing material, Chem. Soc. Rev., 2019, 48, 5266–5302 RSC.
- W. P. Lustig, S. Mukherjee, N. D. Rudd, A. V. Desai, J. Li and S. K. Ghosh, Metal–organic frameworks: functional luminescent and photonic materials for sensing applications, Chem. Soc. Rev., 2017, 46, 3242–3285 RSC.
- X. L. Yang, X. Chen, G. H. Hou, R. F. Guan, R. Shao and M. H. Xie, A multiresponsive metal–organic framework: direct chemiluminescence, photoluminescence, and dual tunable sensing applications, Adv. Funct. Mater., 2016, 26, 393–398 CrossRef CAS.
- S. Pramanik, C. Zheng, X. Zhang, T. J. Emge and J. Li, New microporous metal−organic framework demonstrating unique selectivity for detection of high explosives and aromatic compounds, J. Am. Chem. Soc., 2011, 133, 4153–4155 CrossRef CAS.
- W. P. Lustig, S. Mukherjee, N. D. Rudd, A. V. Desai, J. Li and S. K. Ghosh, Metal–organic frameworks: functional luminescent and photonic materials for sensing applications, Chem. Soc. Rev., 2017, 46, 3242–3285 RSC.
- Y. Zhang, S. Yuan, G. Day, X. Wang, X. Yang and H. C. Zhou, Luminescent sensors based on metal-organic frameworks, Coord. Chem. Rev., 2018, 354, 28–45 CrossRef CAS.
- H. Furukawa, K. E. Cordova, M. O'Keeffe and O. M. Yaghi, ChemInform abstract: the chemistry and applications of metal-organic frameworks, Science, 2013, 341, 1230444 CrossRef.
- C. Xu, C. Bi, Z. Zhu, R. Luo, X. Zhang, D. Zhang, C. Fan, L. Cui and Y. Fan, Metal–organic frameworks with 5,5'-(1,4-xylylenediamino) diisophthalic acid and varied nitrogen-containing ligands for selectively sensing Fe(III)/Cr(VI) and nitroaromatic, CrystEngComm, 2019, 21, 2333–2344 RSC.
- X. X. Wu, H. R. Fu, M. L. Han, Z. Zhou and L. F. Ma, Tetraphenylethylene immobilized metal–organic frameworks: highly sensitive fluorescent sensor for the detection of Cr2O72– and nitroaromatic explosives, Cryst. Growth Des., 2017, 17, 6041–6048 CrossRef CAS.
- H. R. Fu, N. Wang, J. H. Qin, M. L. Han, L. F. Ma and F. Wang, Spatial confinement of a cationic MOF: a SC–SC approach for high capacity Cr(vi)-oxyanion capture in aqueous solution, Chem. Commun., 2018, 54, 11645–11648 RSC.
- Z. S. Qin, W. W. Dong, J. Zhao, Y. P. Wu, Q. C. Zhang and D. S. Li, A water-stable Tb(III)-based metal–organic gel (MOG) for detection of antibiotics and explosives, Inorg. Chem. Front., 2018, 5, 120–126 RSC.
- J. H. Qin, H. R. Wang, M. L. Han, X. H. Chang and L. F. Ma, pH-stable Eu- and Tb-organic-frameworks mediated by an ionic liquid for the aqueous-phase detection of 2,4,6-trinitrophenol (TNP), Dalton Trans., 2017, 46, 15434–15442 RSC.
- D. Zhao, X. H. Liu, Y. Zhao, P. Wang, Y. Liu, M. Azam, S. I. Al-Resayes, Y. Lu and W. Y. Sun, Fluorescent sensing and selective adsorption properties of metal-organic frameworks with mixed tricarboxylate and 1H-imidazol-4-yl-containing ligands, J. Mater. Chem. A, 2017, 5, 15797–15807 RSC.
- S. Srivastava, B. K. Gupta and R. Gupta, Lanthanide-based coordination polymers for the size-selective detection of nitroaromatics, Cryst. Growth Des., 2017, 17, 3907–3916 CrossRef CAS.
- W. Xie, S. R. Zhang, D. Y. Du, J. S. Qin, S. J. Bao, J. Li, Z. M. Su, W. W. He, Q. Fu and Y. Q. Lan, Stable luminescent metal-organic frameworks as dual-functional materials to encapsulate ln(3+) ions for white-light emission and to detect nitroaromatic explosives, Inorg. Chem., 2015, 54, 3290–3296 CrossRef CAS.
- M. L. Han, G. X. Wen, W. W. Dong, Z. H. Zhou, Y. P. Wu, J. Zhao, D. S. Li, L. F. Ma and X. H. Bu, A multi-responsive luminescent sensor based on a super-stable sandwich-type terbium(III)-organic framework, J. Mater. Chem. C, 2017, 5, 8469–8474 RSC.
- C. B. Fan, X. Zhang, N. N. Li, C. G. Xu, R. X. Wu, B. Zhu, G. L. Zhang, S. Y. Bi and Y. H. Fan, Zn-MOFs based luminescent sensors for selective and highly sensitive detection of Fe3+ and tetracycline antibiotic, J. Pharm. Biomed. Anal., 2020, 113444 CrossRef CAS.
- J. H. Wei, J. W. Yi, M. L. Han, B. Li, S. Liu, Y. P. Wu, L. F. Ma and D. S. Li, A new water-stable terbium(III)-organic framework as a chemosensor for inorganic ions, nitro-compounds and antibiotics in aqueous solutions, Chem. – Asian J., 2019, 14, 3694–3701 CrossRef CAS.
- M. K. Yu, Y. Xie, X. Y. Wang, Y. X. Li and G. M. Li, Highly water-stable dye@Ln-MOFs for sensitive and selective detection toward antibiotics in water, ACS Appl. Mater. Interfaces, 2019, 11, 21201–21210 CrossRef CAS.
- M. L. Zhang, Y. J. Zheng, M. Liu, Y. X. Ren, Z. X. Wang, J. Cao and J. J. Wang, Two zinc(II) coordination polymers based on flflexible co-ligands featuring assembly imparted sensing abilities for Cr2O72− and o-NP, RSC Adv., 2019, 9, 21086–21094 RSC.
- M. L. Zhang, Y. J. Zheng, M. Liu, Y. X. Ren, Z. X. Wang, J. Cao and J. J. Wang, Two Cd(II)/Mn(II) coordination polymers showing dual responsive flfluorescence sensing for Fe3+ and o-NAL, J. Solid State Chem., 2019, 277, 693–700 CrossRef CAS.
- M. L. Zhang, Y. Bai, X. G. Yang, Y. J. Zheng, Y. X. Ren, J. J. Wang, M. L. Han, F. F. Li and L. F. Ma, Dense π-stacking of flexible ligands fixed in interpenetrating Zn(II) MOF exhibiting long-lasting phosphorescence and efficient carrier transport, Dalton Trans., 2020, 49, 9961–9964 RSC.
-
G. M. Sheldrick, SHELXL-2014/7, Program for Crystal Structure Determination, University of Gottingen, Germany, 2014 Search PubMed.
-
G. M. Sheldrick, SHELXL-2014/7, Program for Crystal Structure Refinement, University of Gottingen, Germany, 2014 Search PubMed.
- B. Barros, J. Chojnacki, A. Macedo Soares, J. Kulesza, L. Lourenço and S. Junior, Thermostability and photophysical properties of mixed-ligand carboxylate/benzimidazole Zn(II)-coordination polymers, Mater. Chem. Phys., 2015, 162, 364–371 CrossRef CAS.
- J. Meisner, J. Karwounopoulos, P. Walther, K. Johannes and N. Stefan, The lewis pair polymerization of lactones using metal halides and N-heterocyclic
olefins: theoretical insights, Molecules, 2018, 23, 432–446 CrossRef.
- W. Hong, L. Li, R. Xue, X. Xu, H. Wang and J. Zhou, One-pot hydrothermal synthesis of Zinc ferrite/reduced graphene oxide as an efficient electrocatalyst for oxygen reduction reaction, J. Colloid Interface Sci., 2017, 485, 175–182 CrossRef CAS.
- R. Lv, J. Wang, Y. Zhang, H. Li, L. Yang, S. Liao, W. Gu and X. Liu, An amino-decorated dual-functional metal–organic framework for highly selective sensing of Cr(III) and Cr(VI) ions and detection of nitroaromatic explosives, J. Mater. Chem. A, 2016, 4, 15494–15500 RSC.
- T. He, Y. Z. Zhang, X. J. Kong, J. Yu, X. L. Lv, X. Q. Wu, Z. J. Guo and J. R. Li, Tuning water sorption in highlystable Zr(IV)-metal–organic frameworks through local functionalization of metal cluster, ACS Appl. Mater. Interfaces, 2018, 10, 16650–16659 CrossRef CAS.
- J. N. Hao and B. Yan, New. Ln3+ post-functionalized metal–organic frameworks for color tunable emission and highly sensitive sensing of toxic anions and small molecules, J. Chem., 2016, 40, 4654–4661 CAS.
- S. Yao, T. Xu, N. Zhao, L. Zhang, Q. Huo and Y. Liu, An anionic metal-organic framework with ternary building units for rapid and selective adsorption of dyes, Dalton Trans., 2017, 46, 3332–3337 RSC.
- W. J. Gong, R. Yao, H. X. Li, Z. G. Ren, J. G. Zhang and J. P. Lang, Luminescent cadmium(II) coordination polymers of 1,2,4,5-tetrakis(4-pyridylvinyl)benzene used as efficient multi-responsive sensors for toxic metal ions in water, Dalton Trans., 2017, 46, 16861–16871 RSC.
- H. Pan, S. F. Wang, X. Y. Dao and Y. H. Ni, Fluorescent Zn-PDC/Tb3+ coordination polymer nanostructure: a candidate for highly selective detections of cefifixime antibiotic and acetone in aqueous system, Inorg. Chem., 2018, 57(3), 1417–1425 CrossRef CAS.
- G. X. Qin, J. Wang, L. Li, F. F. Yuan, Q. Q. Zha, W. B. Bai and Y. H. Ni, Highly water-stable Cd-MOF/Tb3+ ultrathin fluorescence nanosheets for ultrasensitive and selective detection of cefixime, Talanta, 2021, 221, 12142 CrossRef.
- H. Eskandari, M. Amirzehni, H. Asadollahzadeh, J. Hassanzadeh and P. A. Eslami, MIP-capped terbium MOF-76 for the selective fluorometric detection of cefixime after its preconcentration with magnetic graphene oxide, Sens. Actuators, B, 2018, 275, 145–154 CrossRef CAS.
- C. B. Fan, X. Zhang, N. N. Li, C. G. Xua, R. X. Wu, B. Zhu, G. L. Zhang, S. Y. Bi and Y. H. Fan, Zn-MOFs based luminescent sensors for selective and highly sensitive detection of Fe3+ and tetracycline antibiotic, J. Pharm. Biomed. Anal., 2020, 188, 113444 CrossRef CAS PubMed.
- X. M. Du, Q. W. Wang, Q. Liu, D. Ning, B. Zhao, Y. Li and W. J. Ruan, A dual-emissive MOF for the simultaneous detection of tetrachlorobenzoquinone isomers in their mixtures, J. Mater. Chem. C, 2019, 7, 8626–8633 RSC.
- Y. Zhou, Q. Yang, D. N. Zhang, N. Gan, Q. P. Li and J. Cuan, Detection and removal of antibiotic tetracycline in water with a highly stable luminescent MOF, Sens. Actuators, B, 2018, 262, 137–143 CrossRef CAS.
- X. Y. Xu and B. Yan, Eu( III )-functionalized ZnO@MOF heterostructures:integration of pre-concentration and efficient charge transfer for the fabrication of a ppb-level sensing platform for volatile aldehyde gases in Vehicles, J. Mater. Chem. A, 2017, 5, 2215–2223 RSC.
- A. Mallick, B. Garai, M. A. Addicoat, P. St. Petkov, T. Heine and R. Banerjee, Solid state organic amine detection in a photochromic porous metal organic framework, Chem. Sci., 2015, 6, 1420–1425 RSC.
-
M. J. Frisch, G. W. Trucks and H. B. Schlegel, Gaussian 09, Revision E.01,Gaussian, Inc., Wallingford CT, 2013 Search PubMed.
- B. B. Lu, W. Jiang, J. Yang, Y. Y. Liu and J. F. Ma, Resorcin[4]arene-based microporous metal–organic framework as an efficient catalyst for CO2 cycloaddition with epoxides and highly selective luminescent sensing of Cr2O72−, ACS Appl. Mater. Interfaces, 2017, 9, 39441–39449 CrossRef CAS PubMed.
- D. Gao, Z. Wang, B. Liu, L. Ni, M. Wu and Z. Zhang, Resonance energy transfer-amplifying fluorescence quenching at the surface of silica nanoparticles toward ultrasensitive detection of TNT, Anal. Chem., 2008, 80, 8545–8553 CrossRef CAS PubMed.
- S. S. Jin, X. Han, J. Yang, H. M. Zhang, X. L. Liu and J. F. Ma, Luminescent coordination polymers based on a new resorcin[4]arene-functionalized tetracarboxylate: Highly selective luminescent detection of metal cations, anions and small organic molecules, J. Lumin., 2017, 188, 346–355 CrossRef CAS.
- Z. J. Lin, H. Q. Zheng, H. Y. Zheng, L. P. Lin, Q. Xin and R. Cao, Efficient capture and effective sensing of Cr2O72−from water using a zirconium metal–organic framework, Inorg. Chem., 2017, 56, 14178–14188 CrossRef CAS.
- Y. Rachuri, B. Parmar, K. Bisht and E. Suresh, Multiresponsive adenine-based luminescent Zn(II) coordination polymer for detection of Hg2+and trinitrophenol in aqueous media, Cryst. Growth Des., 2017, 17, 1363–1372 CrossRef CAS.
- A. Buragohain, M. Yousufuddin, M. Sarma and S. Biswas, 3D luminescent amidefunctionalized cadmium tetrazolate framework for selective detection of 2,4,6- trinitrophenol, Cryst. Growth Des., 2016, 16, 842–851 CrossRef CAS.
Footnote |
† Electronic supplementary information (ESI) available. CCDC 2124292–2124295 for 1–4. For ESI and crystallographic data in CIF or other electronic format see DOI: 10.1039/d1ce01261d |
|
This journal is © The Royal Society of Chemistry 2022 |
Click here to see how this site uses Cookies. View our privacy policy here.