Low-energy Ga2O3 polymorphs with low electron effective masses†
Received
17th November 2021
, Accepted 22nd February 2022
First published on 8th March 2022
Abstract
We predict three Ga2O3 polymorphs with P21/c or Pnma symmetry. The formation energies of P21/c Ga2O3, Pnma-I Ga2O3, and Pnma-II Ga2O3 are 57 meV per atom, 51 meV per atom, and 23 meV per atom higher than that of β-Ga2O3, respectively. All the polymorphs are shown to be dynamically and mechanically stable. P21/c Ga2O3 is a quasi-direct wide band gap semiconductor (3.83 eV), while Pnma-I Ga2O3 and Pnma-II Ga2O3 are direct wide band gap semiconductors (3.60 eV and 3.70 eV, respectively). Simulated X-ray diffraction patterns are provided for experimental confirmation of the predicted structures. The polymorphs turn out to provide low electron effective masses, which is of great benefit to high-power electronic devices.
Introduction
Wide band gap semiconductors are important for devices requiring high frequency, temperature, and/or power.1 Many commercial applications use the third generation semiconductors GaN and SiC. However, those suffer from high manufacturing costs and limitations of the achievable performance.2,3
Gallium oxide, especially thermodynamically stable β-Ga2O3, attracts more and more attention, because its high breakdown field leads to a higher Baliga figure of merit for application in high-power electronic devices than provided by SiC and GaN.4 The most famous polymorphs of Ga2O3 are the α-, β-, γ-, δ-, and ε-phases,5,6 which are similar in properties such as the band gap, elastic modulus, and electron effective mass.7 The hexagonal structure of ε-Ga2O3 with space group P63mc enables hetero-epitaxial growth on GaN, AlN, ZnO, and Al2O3.8 While theoretical studies focus on the orthorhombic Pna21 phase,7,9–12 experiments show that ε-Ga2O3 is pyroelectric with large polarization and piezoelectric coupling, being able to host a high density electron gas. Various theoretical studies address the physical properties of the β-phase and experimental studies the preparation of non-β-phases.12–25
In the present work, we predict three Ga2O3 polymorphs, which we call P21/c Ga2O3, Pnma-I Ga2O3, and Pnma-II Ga2O3 according to their space groups. The formation energy of Pnma-II Ga2O3 is found to be 3 meV per atom lower than that of α-Ga2O3. A systematic investigation of the predicted polymorphs is performed, including the stability, electronic states, and carrier effective mass.
Computational details
Based on density functional theory, all calculations are performed with the Cambridge Serial Total Energy Package.26 We use the generalized gradient approximation of Perdew–Burke–Ernzerhof for structure optimization and the Heyd–Scuseria–Ernzerhof hybrid functional for calculating electronic properties. Ultrasoft pseudopotentials27 and the Broyden–Fletcher–Goldfarb–Shanno scheme are adopted. Brillouin zone integrations are performed on Monkhorst–Pack 8 × 5 × 14, 6 × 12 × 4, and 7 × 13 × 3 k-grids for P21/c Ga2O3, Pnma-I Ga2O3, and Pnma-II Ga2O3, respectively. Elastic constants are calculated by the strain-stress method. Phonon spectra are obtained by density functional perturbation theory.28
Results and discussion
The crystal structures of the predicted Ga2O3 polymorphs are shown in Fig. 1. In each case the unit cell contains 8 Ga and 12 O atoms. In P21/c Ga2O3 and Pnma-I Ga2O3 the Ga atoms are 4- or 5-coordinated and the O atoms are 3-coordinated, whereas in Pnma-II Ga2O3 the Ga atoms are 4- or 6-coordinated and the O atoms are 3-coordinated (α-Ga2O3: O atoms 4-coordinated and Ga atoms 6-coordinated; β-Ga2O3: O atoms 3- or 4-coordinated and Ga atoms 4- or 6-coordinated). The lattice parameters of the predicted polymorphs are compared to those of α-Ga2O3 and β-Ga2O3 in Table 1. The theoretical values of α-Ga2O3 and β-Ga2O3 show excellent agreement with the experiment (deviation less than 1.8%), demonstrating reliability of the employed theoretical methodology. The densities of the predicted polymorphs are smaller than those of α-Ga2O3 and β-Ga2O3.
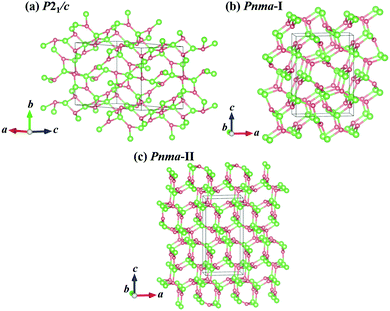 |
| Fig. 1 Crystal structures. Red spheres represent O atoms and green spheres represent Ga atoms. | |
Table 1 Lattice parameters and densities
Ga2O3 |
|
a (Å) |
b (Å) |
c (Å) |
β (°) |
ρ (g cm−3) |
α |
Theory |
4.988 |
|
13.625 |
|
6.46 |
|
Exp.29 |
4.983 |
|
13.433 |
|
6.47 |
β |
Theory |
12.452 |
3.083 |
5.876 |
103.7 |
5.68 |
|
Exp.30 |
12.230 |
3.040 |
5.800 |
103.7 |
5.94 |
P21/c |
Theory |
9.770 |
8.762 |
5.810 |
149.6 |
4.95 |
Pnma-I |
Theory |
7.073 |
3.311 |
9.835 |
|
5.41 |
Pnma-II |
Theory |
5.900 |
3.093 |
12.126 |
|
5.63 |
The phonon spectra of the predicted polymorphs are shown in Fig. 2. Absence of imaginary frequencies indicates dynamical stability. Fig. 2(d) gives the formation energies of selected polymorphs with respect to that of β-Ga2O3 (minimum). The values of the predicted polymorphs are much lower than those of polymorphs from the Materials Project (mp). We calculate for mp-13134 a value of 280 meV per atom in agreement with the mp-result of 284 meV per atom. The formation energies of P21/c Ga2O3, Pnma-I Ga2O3, and Pnma-II Ga2O3 are only moderately higher (57 meV per atom, 51 meV per atom, and 23 meV per atom, respectively) than that of β-Ga2O3. The formation energy of Pnma-II Ga2O3 is even 3 meV per atom lower than that of α-Ga2O3. To verify the stability of the predicted polymorphs at operating temperature, ab initio molecular dynamics simulations are performed at 1000 K (5 ps with a time step of 1 fs). The results in Fig. 3 show no indication of structural instability.
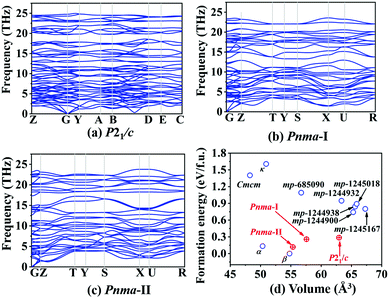 |
| Fig. 2 (a–c) Phonon spectra. (d) Formation energies with respect to β-Ga2O3. | |
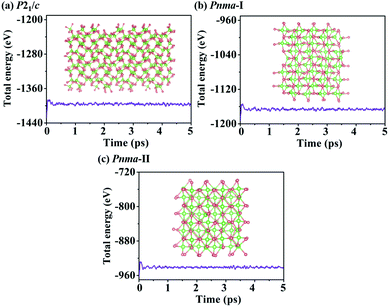 |
| Fig. 3
Ab initio molecular dynamics simulations at 1000 K: total energy and final structures. | |
The elastic matrix of monoclinic P21/c Ga2O3 has 13 independent constants and the elastic matrices of orthorhombic Pnma-I and Pnma-II Ga2O3 have 9 independent constants. The obtained elastic matrices and the Born mechanical stability conditions are given in the ESI† material. The elastic constants in Table 2 show that the Born mechanical stability criteria are fulfilled for all the predicted polymorphs. The bulk modulus B, shear modulus G, and Young's modulus E of Pnma-II Ga2O3 are found to resemble the values of β-Ga2O3 (Table 2).
Table 2 Elastic constants (GPa) and moduli (GPa)
Ga2O3 |
C11 |
C22 |
C33 |
C44 |
C55 |
C66 |
C12 |
C13 |
C23 |
B
|
G
|
E
|
α |
365 |
365 |
327 |
75 |
75 |
108 |
149 |
106 |
106 |
197 |
96 |
248 |
β |
199 |
312 |
298 |
39 |
77 |
95 |
112 |
125 |
62 |
155 |
69 |
180 |
P21/c |
175 |
253 |
204 |
45 |
59 |
47 |
62 |
66 |
88 |
116 |
53 |
138 |
Pnma-I |
210 |
269 |
257 |
53 |
73 |
51 |
90 |
109 |
122 |
152 |
62 |
164 |
Pnma-II |
311 |
300 |
193 |
81 |
96 |
41 |
59 |
116 |
120 |
154 |
71 |
185 |
The obtained electronic band structures in Fig. 4 show a quasi-direct band gap for P21/c Ga2O3 (3.83 eV) and direct band gaps for Pnma-I Ga2O3 (3.60 eV) and Pnma-II Ga2O3 (3.70 eV). Although these band gaps are smaller than that of β-Ga2O3 (4.60 eV),31 they exceed those of SiC (2.40 eV), GaN (3.20 eV), and ZnO (3.44 eV).32 Note that the calculated band gap of β-Ga2O3 is 4.60 eV, in perfect agreement with the experimental result of ref. 31.
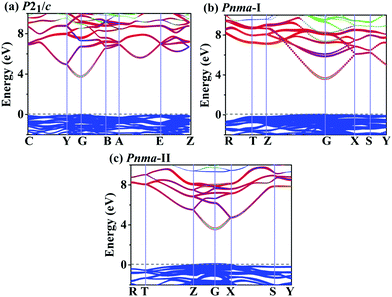 |
| Fig. 4 Electronic band structures. Red, green, cyan, and blue colors represent Ga s, Ga p, O s, and O p contributions, respectively. The high symmetry points are C (0.0, 0.5, 0.5), Y (0.0, 0.5, 0.0), G (0.0, 0.0, 0.0), B (0.5, 0.0, 0.0), A (0.5, 0.5, 0.0), E (0.5, 0.5, 0.5), and Z (0.0, 0.0, 0.5) for P21/c symmetry and R (−0.5, 0.5, 0.5), T (−0.5, 0.0, 0.5), Z (0.0, 0.0, 0.5), G (0.0, 0.0, 0.0), X (0.0, 0.5, 0.0), S (−0.5, 0.5, 0.0), and Y (−0.5, 0.0, 0.0) for Pnma symmetry. | |
We present the calculated electron and hole effective masses along the a-, b-, and c-axes in Table 3. The electron effective masses of Pnma-I Ga2O3 are similar to those of α-Ga2O3 and β-Ga2O3, those of P21/c Ga2O3 are a bit larger, and those of Pnma-II Ga2O3 are significantly larger by a factor of almost 2.5. We find hardly any anisotropy for the electron effective masses. For P21/c Ga2O3 the minimum appears not in a special direction, for Pnma-I Ga2O3 it appears along the a-axis, and for Pnma-II Ga2O3 it appears along the b-axis. The hole effective masses show significant anisotropy. For both P21/c Ga2O3 and Pnma-II Ga2O3 the minimum appears along the b-axis and for Pnma-I Ga2O3 it appears along the c-axis. P21/c Ga2O3 and Pnma-II Ga2O3 show heavy fermion behavior in specific directions (Table 3).
Table 3 Electron and hole effective masses (multiples of the free electron mass)
Ga2O3 |
m
a
|
Electron effective mass |
m
b
|
m
c
|
m
max
|
Direction |
m
min
|
Direction |
α |
0.21 |
0.21 |
0.21 |
0.21 |
[1, 1, 1] |
0.21 |
[1, 1, 1] |
β |
0.22 |
0.22 |
0.21 |
0.23 |
[0.68, −0.73, 0.02] |
0.21 |
[−0.22, −0.22, 0.95] |
P21/c |
0.26 |
0.26 |
0.26 |
0.26 |
[0, 1, 0] |
0.26 |
[−0.62, 0, 0.78] |
Pnma-I |
0.20 |
0.23 |
0.23 |
0.23 |
[0, 1, 0] |
0.20 |
[1, 0, 0] |
Pnma-II |
0.51 |
0.51 |
0.54 |
0.54 |
[0, 0, 1] |
0.51 |
[0, 1, 0] |
Ga2O3 |
m
a
|
Hole effective mass |
m
b
|
m
c
|
m
max
|
Direction |
m
min
|
Direction |
α |
2.54 |
2.54 |
0.80 |
3.47 |
[0.67, 0.67, 0.31] |
0.71 |
[0.25, 0.25, 0.93] |
β |
2.81 |
3.49 |
4.54 |
6.06 |
[0.25, 0.56, 0.79] |
2.57 |
[−0.85, −0.53, 0.04] |
P21/c |
2.55 |
2.26 |
5.03 |
>50 |
[−0.56, 0, 0.83] |
2.26 |
[0, 1, 0] |
Pnma-I |
2.29 |
2.29 |
0.31 |
2.29 |
[1, 0, 0] |
0.31 |
[0, 0, 1] |
Pnma-II |
4.07 |
0.37 |
>50 |
>50 |
[0, 0, 1] |
4.07 |
[0, 1, 0] |
The simulated X-ray diffraction patterns of α-Ga2O3, β-Ga2O3, P21/c Ga2O3, Pnma-I Ga2O3, and Pnma-II Ga2O3 are displayed in Fig. 5. The (111) and (400) peaks of β-Ga2O3 (at 34.7° and 29.5°, respectively) coincide with the mp-results. Though they belong to the same space group, the spectra of Pnma-I Ga2O3 and Pnma-II Ga2O3 reveal notable differences. The strongest peaks are (22–1) at 27.3° for P21/c Ga2O3, (111) at 33.5° for Pnma-I Ga2O3, and (112) at 35.9° for Pnma-II Ga2O3. The X-ray characteristics enable identification of the predicted polymorphs in future experiments.
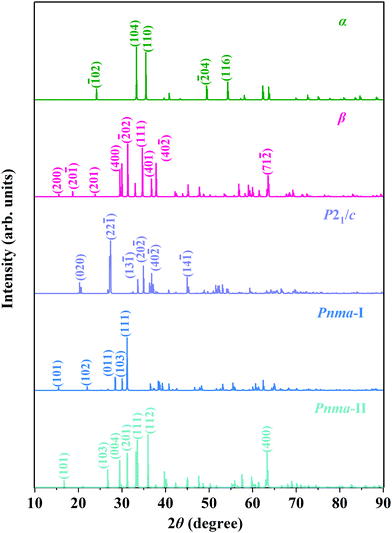 |
| Fig. 5 X-Ray diffraction patterns (Cu source with a wavelength of 1.5406 Å). | |
Conclusions
In conclusion, three low-energy Ga2O3 polymorphs with wide band gaps are predicted to be dynamically and mechanically stable. P21/c Ga2O3 and Pnma-I Ga2O3 realize a novel 5-coordination of the Ga atoms. The band gap is quasi-direct for P21/c Ga2O3 and direct for Pnma-I Ga2O3 and Pnma-II Ga2O3. The electron and hole effective masses show significant differences from those of α-Ga2O3 and β-Ga2O3. Simulated X-ray diffraction patterns enable experimental confirmation of the predicted structures. Remarkably, chemical vapor deposition can be used to synthesize a large variety of Ga2O3 polymorphs, adopting temperatures of 410 °C,33 550 °C,34 and 650 °C.35 When aiming for synthesis of the predicted polymorphs by this method, identifying a suitable growth temperature is the main experimental uncertainty. However, since structural stability is maintained beyond 1000 K, it is reasonable to expect that appropriate growth parameters can be established.
Data availability statement
The data that supports the findings of this study are available within the article and its supplementary material.
Conflicts of interest
The authors have no conflicts to disclose.
Acknowledgements
The authors acknowledge generous financial support from the National Natural Science Foundation of China (No. 61804120), China Postdoctoral Science Foundation (No. 2019TQ0243 and 2019M663646), Key Scientific Research Plan of the Education Department of Shaanxi Province (Key Laboratory Project) (No. 20JS066), and Young Talent Fund of the University Association for Science and Technology in Shaanxi, China (No. 20190110). The research reported in this publication was supported by funding from King Abdullah University of Science and Technology (KAUST).
References
-
C. G. Van de Walle, Wide-band-gap semiconductors, Elsevier, Amsterdam, 2012 Search PubMed.
- W. Kowbel, C. A. Bruce, K. L. Tsou, K. Patel, J. C. Withers and G. E. Youngblood, High thermal conductivity SiC/SiC composites for fusion applications, J. Nucl. Mater., 2000, 283–287, 570–573 CrossRef CAS.
- J. Kim, D. Tahara, Y. Miura and B. G. Kim, First-principle calculations of electronic structures and polar properties of (κ,ε)-Ga2O3, Appl. Phys. Express, 2018, 11, 061101 CrossRef.
- M. Higashiwaki, K. Sasaki, H. Murakami, Y. Kumagai, A. Koukitu, A. Kuramata, T. Masui and S. Yamakoshi, Recent progress in Ga2O3 power devices, Semicond. Sci. Technol., 2016, 31, 034001 CrossRef.
- R. Roy, V. G. Hill and E. F. Osborn, Polymorphism of Ga2O3 and the system Ga2O3–H2O, J. Am. Chem. Soc., 1952, 74, 719–722 CrossRef CAS.
- J. Furthmüller and F. Bechstedt, Quasiparticle bands and spectra of Ga2O3 polymorphs, Phys. Rev. B, 2016, 93, 115204 CrossRef.
- S. Yoshioka, H. Hayashi, A. Kuwabara, F. Oba, K. Matsunaga and I. Tanaka, Structures and energetics of Ga2O3 polymorphs, J. Phys.: Condens. Matter, 2007, 19, 346211 CrossRef.
- H. Y. Playford, A. C. Hannon, E. R. Barney and R. I. Walton, Structures of uncharacterised polymorphs of gallium oxide from total neutron diffraction, Chem. – Eur. J., 2013, 19, 2803–2813 CrossRef CAS PubMed.
- I. Cora, F. Mezzadri, F. Boschi, M. Bosi, M. Čaplovicová, G. Calestani, I. Dodony, B. Pecz and R. Fornari, The real structure of ε-Ga2O3 and its relation to κ-phase, CrystEngComm, 2017, 19, 1509–1516 RSC.
- M. B. Maccioni and V. Fiorentini, Phase diagram and polarization of stable phases of (Ga1−xInx)2O3, Appl. Phys. Express, 2016, 9, 041102 CrossRef.
- M. Kracht, A. Karg, J. Schörmann, M. Weinhold, D. Zink, F. Michel, M. Rohnke, M. Schowalter, B. Gerken, A. Rosenauer, P. J. Klar, J. Janek and M. Eickhoff, Tin-assisted synthesis of ε-Ga2O3 by molecular beam epitaxy, Phys. Rev. Appl., 2017, 8, 054002 CrossRef.
- K. Yamaguchi, First principles study on electronic structure of β-Ga2O3, Solid State Commun., 2004, 131, 739–744 CrossRef CAS.
- S. Ohira, N. Suzuki, N. Arai, M. Tanaka, T. Sugawara, K. Nakajima and T. Shishido, Characterization of transparent and conducting Sn-doped β-Ga2O3 single crystal after annealing, Thin Solid Films, 2008, 516, 5763–5767 CrossRef CAS.
- G. Q. Pei, C. T. Xia, Y. J. Dong, B. Wu, T. Wang and J. Xu, Studies of magnetic interactions in Mn-doped β-Ga2O3 from first-principles calculations, Scr. Mater., 2008, 58, 943–946 CrossRef CAS.
- W. Z. Xiao, L. L. Wang, L. Xu, Q. Wan and B. S. Zou, Electronic structure and magnetic interactions in Ni-doped β-Ga2O3 from first-principles calculations, Scr. Mater., 2009, 61, 477–480 CrossRef CAS.
- Y. J. Zhang, J. L. Yan, G. Zhao and W. F. Xie, First-principles study on electronic structure and optical properties of Sn-doped β-Ga2O3, Physica B, 2010, 405, 3899–3903 CrossRef CAS.
- W. Z. Xiao, L. L. Wang, L. Xu, Q. Wan and A. L. Pan, Electronic structure and
magnetic properties in nitrogen-doped-Ga2O3 from density functional calculations, Solid State Commun., 2010, 150, 852–856 CrossRef CAS.
- A. Kumar and U. Singisetti, First principles study of thermoelectric properties of β-gallium oxide, Appl. Phys. Lett., 2020, 117, 262104 CrossRef CAS.
- Y. J. Zhang, J. L. Yan, Q. S. Li, C. Qu, L. Y. Zhang and T. Li, Structural and optical properties of N-doped β-Ga2O3 films deposited by RF magnetron sputtering, Physica B, 2011, 406, 3079–3082 CrossRef CAS.
- Y. J. Zhang, J. L. Yan, Q. S. Li, C. Qu, L. Y. Zhang and W. F. Xie, Optical and structural properties of Cu-doped β-Ga2O3 films, Mater. Sci. Eng., B, 2011, 176, 846–849 CrossRef CAS.
- L. Y. Zhang, J. L. Yan, Y. J. Zhang, T. Li and X. W. Ding, First-principles study on electronic structure and optical properties of N-doped p-type β-Ga2O3, Sci. China: Phys., Mech. Astron., 2012, 55, 19–24 CrossRef CAS.
- S. Seacat, J. L. Lyons and H. Peelaers, Orthorhombic alloys of Ga2O3 and Al2O3, Appl. Phys. Lett., 2020, 116, 232102 CrossRef CAS.
- W. S. Li, D. Saraswat, Y. Y. Long, K. Nomoto, D. Jena and H. G. Xing, Near-ideal reverse leakage current and practical maximum electric field in β-Ga2O3 Schottky barrier diodes, Appl. Phys. Lett., 2020, 116, 192101 CrossRef CAS.
- J. B. Varley, A. Perron, V. Lordi, D. Wickramaratne and J. L. Lyons, Prospects for n-type doping of (AlxGa1−x)2O3 alloys, Appl. Phys. Lett., 2020, 116, 172104 CrossRef.
- Z. A. Jian, S. Mohanty and E. Ahmadi, Deep UV-assisted capacitance-voltage characterization of post-deposition annealed Al2O3/β-Ga2O3 (001) MOSCAPs, Appl. Phys. Lett., 2020, 116, 242105 CrossRef CAS.
- S. J. Clark, M. D. Segall, C. J. Pickard, P. J. Hasnip, M. J. Probert, K. Refson and M. C. Payne, First principles methods using CASTEP, Z. Kristallogr., 2005, 220, 567–570 CAS.
- D. Vanderbilt, Soft self-consistent pseudopotentials in a generalized eigenvalue formalism, Phys. Rev. B: Condens. Matter Mater. Phys., 1990, 41, 7892–7895 CrossRef PubMed.
- S. Baroni, S. D. Gironcoli, A. D. Corso and P. Giannozzi, Phonons and related crystal properties from density-functional perturbation theory, Rev. Mod. Phys., 2001, 73, 515–562 CrossRef CAS.
- M. Marezio and J. P. Remeika, Bond lengths in the α-Ga2O3 structure and the high-pressure phase of Ga2−xFexO3, J. Chem. Phys., 1967, 46, 1862–1865 CrossRef CAS.
- S. Geller, Crystal structure of β-Ga2O3, J. Chem. Phys., 1960, 33, 676–684 CrossRef CAS.
- H. H. Tippins, Optical absorption and photoconductivity in the band edge of β-Ga2O3, Phys. Rev., 1965, 140, A316–A319 CrossRef.
- F. Tran and P. Blaha, Accurate band gaps of semiconductors and insulators with a semilocal exchange-correlation potential, Phys. Rev. Lett., 2009, 102, 226401 CrossRef PubMed.
- M. Orita, H. Hiramatsu, H. Ohta, M. Hirano and H. Hosono, Preparation of highly conductive, deep ultraviolet transparent β-Ga2O3 thin film at low deposition temperatures, Thin Solid Films, 2002, 411, 134–139 CrossRef CAS.
- Y. Oshima, E. G. Víllora, Y. Matsushita, S. Yamamoto and K. Shimamura, Epitaxial growth of phase-pure ε-Ga2O3 by halide vapor phase epitaxy, J. Appl. Phys., 2015, 118, 085301 CrossRef.
- F. Boschi, M. Bosi, T. Berzina, E. Buffagni, C. Ferrari and R. Fornari, Hetero-epitaxy of β-Ga2O3 layers by MOCVD and ALD, J. Cryst. Growth, 2016, 443, 25–30 CrossRef CAS.
Footnote |
† Electronic supplementary information (ESI) available. See DOI: 10.1039/d1cp05271c |
|
This journal is © the Owner Societies 2022 |
Click here to see how this site uses Cookies. View our privacy policy here.