In situ insight into the self-assembly evolution of ABA-type block copolymers in water during the gelation process using infrared spectroscopy and near-infrared spectroscopy†
Received
18th February 2022
, Accepted 20th June 2022
First published on 20th June 2022
Abstract
As a kind of thermo-responsive hydrogel, amphiphilic block copolymers are widely investigated. However, the molecular mechanism of their structural change during the gelation process is still limited. Here, a well-controlled triblock copolymer poly(N,N-dimethylacrylamide)-b-poly(diacetone acrylamide)-b-poly(N,N-dimethylacrylamide) (PDMAA-b-PDAAM-b-PDMAA) was synthesized. Its optical microrheology results suggest a gelation temperature range from 42 to 50 °C, showing a transition from viscosity to elasticity. The morphological transition from spheres to worms occurs. Temperature-dependent IR spectra through two-dimensional correlation spectroscopy (2D-COS) and the Gaussian fitting technique were analyzed to obtain the transition information of the molecular structure within the triblock copolymer. The N-way principal component analysis (NPCA) on the temperature-dependent NIR spectra was performed to understand the molecular interaction between water and the copolymer. The intramolecular hydrogen bonds within the hydrophobic PDAAM block tend to dissociate with temperature, resulting in improved hydration and a relative volume increase of the PDAAM block. The dissociation of intermolecular hydrogen bonds within the PDAAM block was the driving force for the morphological transition. Moreover, the hydrophilic PDMAA block dehydrates with temperature, and three stages can be found. The dehydration rate of the second stage with temperature from 42 to 50 °C was obviously higher than those in the lower (first stage) and higher (third stage) temperature ranges.
1. Introduction
As a kind of important soft matter, hydrogels have been widely utilized in various fields, such as tissue engineering scaffolds,1,2 cell cultures,3 and drug delivery and controlled release,4,5 due to their particular features and unique properties. Of the polymers showing a reversible sol–gel transition performance, poly(N-isopropylacrylamide) (PNIPAM) and poly(ethylene oxide) (PEO) are the most studied, whose homopolymers have a thermo-responsive performance.6,7 However, the block copolymer can self-assemble into various morphologies and show a sol–gel transition with temperature in a specific selective solvent,8–10,57 even if the homopolymers of each block are not temperature-sensitive. Through the temperature-dependent volume variation of both hydrophobic and hydrophilic domains, amphiphilic block copolymers can self-assemble into worm-like micelles above a certain temperature in water, with a core formed by the hydrophobic block and a micellar corona formed by the hydrophilic block. The self-assembled block copolymer micelles can also depress the surface free energy of the system and act as gel-forming fibrils.11
It has been well accepted that the self-assembled morphology of block copolymers is determined by the packing parameter (P), given by the following equation:12
where
v is the volume of the hydrophobic block,
lc is the chain length of the hydrophobic block, and
a0 represents the effective interfacial area of the block junction. When
P < 1/3 and 1/3 <
P < 1/2, spherical and worm-like morphologies can be obtained, respectively. Thus, it means that once the gradually increasing value of
P with temperature exceeds 1/3, the morphological transition from spheres to worms would occur and in turn result in a gelation process.
Via reversible addition–fragmentation chain transfer (RAFT) polymerization, Arms
et al. synthesized the amphiphilic block copolymers of poly(glycerol-monomethacrylate)-
b-poly(2-hydroxypropyl methacrylate) (PGMA-
b-PHPMA) and reported the gelation phenomenon of their aqueous solution during the heating process.
13 They also found the reversible sol-gel transition of poly(lauryl methacrylate)-
b-poly(benzyl methacrylate) (PLMA-
b-PBzMA) in the
n-dodecane solvent.
14 A classic view is that the entanglement of worm-like micelles after heating leads to the gelation of the amphiphilic block copolymer.
15 However, Lovett
et al. reported that the percolation network formed by multiple inter-worm contacts results in the formation of a hydrogel.
16 Cui
et al. used a dynamic Monte Carlo simulation to further analyze the thermo-responsive gelation and found that a hydrophobic channel evolved from the semi-bald micelle might play a key role in the gelation process.
17 Combining with the light scattering technique, Zaccone
et al. developed a hydrodynamic model to quantify the microscopic association or dissociation rates of thermosensitive nanoparticles, which can successfully explain the switchable behavior of PNIPAM through the hydrophobic–hydrophilic transition.
18,19 However, for the amphiphilic block micellar system and its thermo-responsive performance, little knowledge is obtained about the molecular mechanism of structural evolution in the gelation process with temperature, especially about the molecular interactions within the copolymer chains and between copolymer chains and water molecules.
Recently, infrared (IR) spectroscopy with temperature disturbance has become a powerful technique to study the molecular structure of thermo-responsive polymers.20–24 The spectral information of the polar groups in polymers, such as NH, CH, and CO groups, is very significant to reflect the variation of molecular structure in a phase transition. Near-infrared (NIR) spectroscopy has been proved to be a powerful tool to investigate the molecular interactions in the water-containing system,25–28 such as hydration and dehydration.29,30 For example, the effect of urea on promoting dehydration of the PNIPAM solution has been explored by temperature-dependent NIR, and the reduction of water molecules with three hydrogen bonds (S3) in the solution caused by urea may be the main reason for the acceleration.31 In our previous study, the ABA-type triblock copolymers of poly(N,N-dimethylacrylamide)-b-poly(diacetone acrylamide)-b-poly(N,N-dimethylacrylamide) (PDMAA-b-PDAAM-b-PDMAA) were found to have a reversible sol-gel transition with temperature in water.32 It is worth noting that the DAAM is a water-soluble monomer and forms a water-insoluble homopolymer at a mean degree of polymerization (DP) as low as 50,46 which forms a partly hydrophobic core instead of completely hydrophobic in the block copolymer. By controlling the chain length of hydrophilic and hydrophobic blocks, the transition temperature (Tgel) could be adjusted, even to a temperature lower than the freezing point of water.33 Following these results, it can be assumed that the exchange of hydrogen bonding within polymer chains, as well as the interaction between polymer and water, would give crucial effects on the structure design of such materials.
In this work, we synthesized the ABA-type triblock copolymer micellar system of PDMAA-b-PDAAM-b-PDMAA. The dynamic light scattering and optical microrheology results indicated a gelation temperature range from 42 to 50 °C. By using temperature-dependent IR and NIR spectroscopy, both the variation of molecular interaction within copolymer and between copolymer and water were analyzed in detail. It is found that the intramolecular hydrogen bonds within the hydrophobic PDAAM block would dissociate with temperature, which acted as the main driving force for gelation. The dehydration of the hydrophilic block is mainly caused by weakened interaction between the water molecules and PDMAA with temperature.
2. Materials and methods
2.1 Materials
RAFT agent S,S′-bis(R,R′-dimethyl-R′′-acetic acid)-trithiocarbonate (BDAAT) was self-synthesized and purified according to the literature.34 The materials used are as follows: diacetone acrylamide (DAAM, Shanghai Macklin Biochemical Co., Ltd), N,N-dimethylacrylamide (DMAA, Shanghai Macklin Biochemical Co., Ltd), 4,4′-azobis (4-cyanovaleric acid) (ACVA, Shanghai Macklin Biochemical Co., Ltd), 1,4-dioxane (>99%, Beijing Chemical Works), and n-hexane (>99%, Beijing Chemical Works). Deionized water was self-prepared in our laboratory.
2.2 Synthesis of PDMAA-b-PDAAM-b-PDMAA triblock copolymers
The ABA-type triblock copolymer of PDMAA-b-PDAAM-b-PDMAA with a designed chain structure was synthesized via RAFT polymerization, where the PDMAA block and the PDAAM block have a DP at 40 and 50, respectively. The hydrophilic chain transfer agent of PDMAA-CTA was firstly synthesized by using ACVA and BDAAT as the initiator and RAFT agent, respectively. The solution of ACVA, BDAAT, DMAA, and 1,3,5-trioxane (as an internal standard) in 1,4-dioxane (20 wt%) with a molar ratio of 0.2
:
1:40
:
1 was added into a glass tube with a magnetic stir bar. RAFT polymerization was carried out at 70 °C for 3 h after refrigerating and filling with nitrogen for three cycles. Then, the solution was precipitated using n-hexane and further dried in a vacuum oven at 45 °C overnight to obtain yellow PDMAA-CTA powder. Furthermore, the solution of ACVA, PDMAA-CTA, and DAAM in 1,4-dioxane (20 wt%) with a molar ratio of 0.2
:
1:50 was added into a glass tube. And the reaction was carried out for 4 h at 70 °C after deoxygenation. Finally, the yellow powder of PDMAA-b-PDAAM-b-PDMAA was obtained after precipitation purification. Aqueous solutions containing 10, 15, and 20 wt% of the synthesized product were prepared using redistilled water as the solvent.
2.3 Dynamic light scattering (DLS) and microrheological measurement
The temperature-dependent Z-average size of copolymer particles (20 wt% aqueous dispersion) was analyzed from 35 to 60 °C using a Malvern Zetasizer Nano-ZS. The sample temperatures were stepwise raised with an increment of 1 °C at a rate of 1 °C min−1 and kept at each temperature for 2 min before repeating measurements 3 times. And the results were determined by the average value. The microrheological properties of 20 wt% copolymer solution for 4 ml were measured with a Rheolaser Master (Formulation, l’Union, France). Since the solution was transparent at low temperatures, four drops of silicone acrylic emulsion were added into the system as a tracer. The transparent solution was measured from 38 to 55 °C with 0.25 °C min−1 to get a better result.
2.4 Temperature-dependent IR and NIR spectra
For IR measurements, the sample of PDMAA-b-PDAAM-b-PDMAA·D2O solution was sealed between two CaF2 tablets for 15 μm using a tetrafluoroethylene gasket. All the temperature-dependent IR spectra were recorded utilizing a Spectrum-100 (PerkinElmer, the U. S.) IR spectrometer equipped with a DTGS detector. In order to obtain an acceptable signal-to-noise ratio, 20 scans at a resolution of 4 cm−1 were accumulated. A Linkam THMS600 was used to control the temperature from 30 to 65 °C (the interval was 1 °C). The heating rate was 1 °C min−1. The system was kept to rest for 2 min before the test to stabilize the temperature. To ensure that the spectral intensity is appropriate, the intensity of OD bands of D2O overflows.
All NIR spectra were recorded using a Vertex 70 spectrometer (Bruker Optics Inc., Ettlingen, Germany) equipped with a tungsten-halogen light source and an InGaAs detector. The cuvette with a length of 0.5 mm was used. The spectra were measured at 4 cm−1 resolution from 12
000 to 4000 cm−1 and the spectrum of empty cells was used as a reference. 64 scans were accumulated. The temperature in the experiment was controlled with a model 2216e temperature controller (Bruker Optics Inc., Ettlingen, Germany). The precision of the equipment for temperature control was ±0.1 °C. The spectra of water and aqueous PDMAA-b-PDAAM-b-PDMAA solution were measured at temperatures from 30 to 76 °C with a step of 2 °C. For each temperature, the spectrum was measured at every minute within 20 min and the spectra measured at 11 to 20 min were adopted in the analysis due to the stable temperature after 10 min.
2.5 Techniques and methods for spectral analysis
Two-dimensional correlation spectroscopy (2D-COS) analysis on IR spectra was carried out using 2D Shige software, ver. 1.3 (Shigeaki Morita, Kwansei-Gakuin University, Japan, 2004–2005). The results were plotted as contour maps, in which the yellow color represents positive correlation intensities and the blue color represents negative correlation intensities, respectively.
Gaussian peak via genetic algorithm was performed to fit the IR spectrum. To obtain a better fitting result, a single-peak Gaussian function was used to represent the absorption band of a single component, and the overlapping absorption peaks were decomposed into a linear combination of multiple Gaussian peaks. More illustrations about the genetic algorithm can be seen in the ESI.†
Continuous wavelet transform (CWT) is employed for removing the variant background in the NIR spectra and enhancing the resolution of the spectra. As generally used, symmlet with vanishing moment 2 (Sym2) was adopted. The scale parameter was set to 30 in this study for enhancing the smoothing effect. The result obtained by CWT with the Sym2 filter was an approximate equivalence of the second derivative.35
N-way principal component analysis (NPCA) was performed to observe the spectral changes of the polymer and water from the NIR spectra measured at different temperatures and times for the solutions of different concentrations. The arrangement of a four-way data array and the calculation were conducted in the same way as in the previous works.36 The loadings and the scores of time, temperature and concentration can be obtained. The four matrices represent the features contained in the spectra and the weights of the features at each time, temperature and concentration, respectively. Therefore, spectral features can be observed from the loadings and the corresponding variation with time, temperature and concentration can be shown by the scores, respectively.
3. Results and discussion
3.1 Synthesis and characterization of PDMAA-b-PDAAM-b-PDMAA
Via a two-step RAFT polymerization as sketched in Scheme S1, the PDMAA-b-PDAAM-b-PDMAA triblock copolymers with a well-controlled DP and a relatively narrow PDI (∼1.29) were synthesized proved by the 1H-NMR curves (Fig. S1, ESI†), and GPC result (Fig. S2, ESI†). The DP of the hydrophilic and hydrophobic blocks are at 40 and 50, respectively. The temperature-dependent DLS result of the aqueous solution (20 wt%) from 35 to 60 °C in Fig. 1a suggests an obvious transition of Z-average size, attributed to the gelation with a Tgel of around 50 °C. It is also consistent with a typical profile of LCST-type sol–gel transition,32 which can be caused by the variation of self-assembled morphology from spheres to worms.
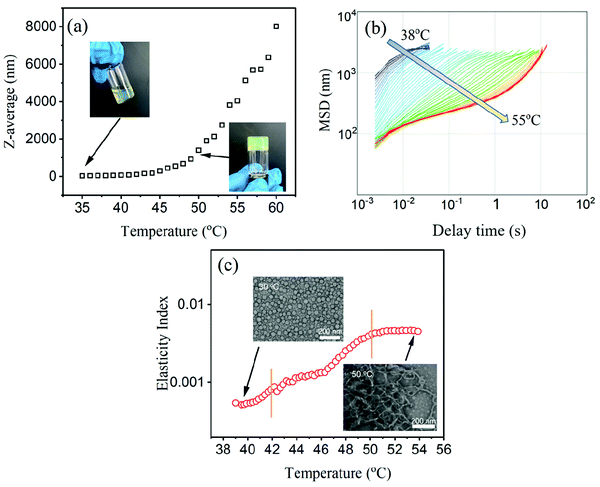 |
| Fig. 1 Characterization results of PDMAA-b-PDAAM-b-PDMAA 20 wt% aqueous solution from 38 to 55 °C: (a) plots of Z-average size; (b) MSD curves measured by microrheology; (c) plots of EI calculated by MSD curves. | |
Microrheology was employed to analyze the microscopic motion state of the dispersed phases through the fluctuation of backscattered light. Rheological characteristics of polymer dispersions are described by plotting the mean square displacement (MSD) of the induced tracer particles as a function of delay time. A linear MSD curve represents a viscous state, and a nonlinear one with a clear platform shows elastic behavior.37 The linear curves gradually turn into the nonlinear ones in Fig. 1b, indicating a transition from a viscous system to an elastic system. For the MSD curves measured by microrheology, the appearance of the plateau region is due to the obstructed particle motion in the copolymer dispersion. The elasticity index (EI) in Fig. 1c is determined by the reciprocal of this plateau value at the short decorrelation timescales, which can reflect the tightness of the fibrillar network of the copolymer dispersion. The increase of EI suggests that the fibrillar network becomes tighter with the improved temperature. Moreover, compared with the results of the torque rheometer shown in Fig. S3 in the ESI,† there are the same variation trends of EI as well as the storage modulus (G′) and the loss modulus (G′′) measured microrheology with the increase of temperature.
The TEM images of the copolymer dispersion in Fig. 1c and Fig. S4 (ESI†) clearly suggest the self-assembled morphology transformation from spherical micelles at low temperature (30 °C) to worm-like micelles at high temperature (50 °C). The fibrillar network becomes tighter as the number of worms increases, and the gelation occurs at 50 °C. This self-assembled morphology transformation to form a fibrillar hydrogel is also very similar to the previous report.38 Above 50 °C, the system remains stable due to the formation of a hydrogel. It can be found from DLS and microrheology results that the gelation of PDMAA-b-PDAAM-b-PDMAA is a continuous transformation process from 42 to 50 °C.
3.2 Temperature-dependent IR spectra in gelation transition
Temperature-dependent IR spectra of PDMAA-b-PDAAM-b-PDMAA copolymer solution (20 wt%) were recorded from 30 to 65 °C. To avoid the influence of OH stretching bending vibration around 3300 and 1640 cm−1, D2O was used as the solvent, instead of H2O. Three spectral regions of the NH stretching vibration (3700–3100 cm−1), the CH stretching vibration (3100–2800 cm−1), and the CO stretching vibration (1740–1570 cm−1) were focused.
The IR bands of 2975 and 2937 cm−1 are due to the antisymmetric stretching vibration of the CH3 and CH2 groups in Fig. 2a, respectively.39 According to Fig. S6a (ESI†), the C–H band around 2975 cm−1 is attributed to the hydrophobic PDAAM block. Compared with the C–H band at 2973 cm−1 of copolymer powder (Fig. S6d, ESI†), the C–H band of copolymer solution moves to 2975 cm−1 (Fig. S6c, ESI†). The C–H bands in a high wavenumber indicate that the hydrophobic PDAAM block of copolymer solution is relatively hydrated instead of completely hydrophobic. For the well-investigated thermo-responsive polymers, such as PNIPAM in water, a redshift of C–H bands usually occurs with temperature due to dehydration.40–42 However, for the aqueous copolymer in water in our work, the band at 2975 cm−1 moves to 2977 cm−1 with the temperature (Fig. S6c, ESI†), indicating a more hydrated PDAAM core of the copolymer during the gelation process. The intensity plots of 2975 cm−1 in Fig. 2e also show a clear inflection point around 42 °C, being identical to the microrheological result and corresponding to the appearance to form the worm-like micelles. The blueshift of the spectra may cause the intensity change.
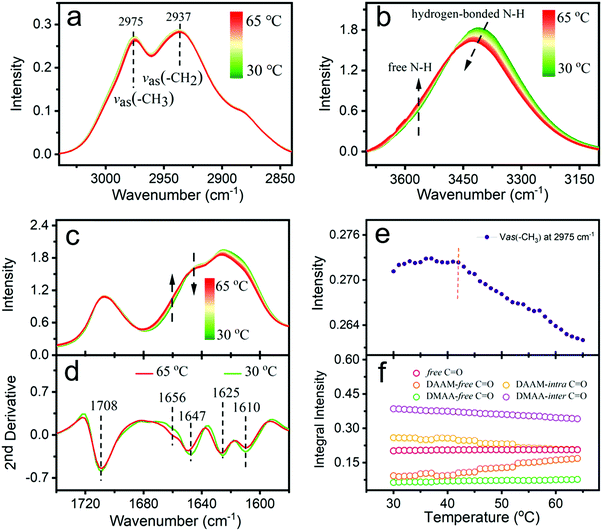 |
| Fig. 2 Temperature-dependent IR spectra of PDMAA-b-PDAAM-b-PMAA from 30 to 65 °C at (a) 3700–3100 cm−1, (b) 3100–2800 cm−1, and (c) 1740–1570 cm−1, and (d) the corresponding second-derivative curves. (e) Plot of intensity at 2975 cm−1 of the CH groups and (f) variation of the integral intensities for the C O band region in the IR spectra with temperature. | |
A bidirectional spectral intensity in the NH stretching region is clearly displayed in Fig. 2b, in which the IR bands can be roughly divided into two parts due to the free NH groups and the hydrogen-bonded NH groups of PDAAM at high and low frequency, respectively. The intensity of the high-frequency moiety increases, while the low one decreases, demonstrating that the hydrogen-bonded NH groups of PDAAM dissociate with temperature.
According to the IR spectra of PDMAA and PDAAM homopolymers in Fig. S7 (ESI†), the IR band assignment in the C
O stretching vibration region is summarized in Table 1, including the list of their abbreviations. A bidirectional band variation in the range of 1680–1640 cm−1 can be also obtained in Fig. 2c. In the second-derivative curve (Fig. 2d), the intensity due to DAAM-free C
O noticeably increases, indicating the dissociation of intramolecular hydrogen bonds within PDAAM and the strength of intramolecular hydrogen bonds is also weakened. It is also consistent with the intensity variation and band shift of NH groups.
Table 1 Band assignments of the PDMAA-b-PDAAM-b-PDMAA triblock copolymer in the C
O stretching vibration region
Wavenumber (cm−1) |
Abbreviation |
Assignments |
1708 |
free C O |
C O group of PDAAM away from backbone |
1656 |
DAAM-free C O |
C O groups of PDAAM close to backbone at free state |
1647 |
DAAM-intra C O |
hydrogen-bonded C O groups of PDAAM close to backbone |
1625 |
DMAA-free C O |
C O grouops of PDMAA at dehydration state |
1610 |
DMAA-inter C O |
C O grouops of PDMAA hydrated with water |
To quantitatively analyze the variation of each C
O band, Gaussian fitting of the genetic algorithm (Fig. S5, ESI†) was employed to fit the IR spectrum from 1740 to 1570 cm−1. More illustrations about the genetic algorithm can be seen in the supporting information. Here, initial peak positions are recognized by the second-derivation curve, and the results are plotted in Fig. 2f. The integral intensity of the free C
O band is almost constant during the gelation process. We can see directly that the DAAM-free C
O band increases and the DAAM-intra C
O band decreases with temperature, which is due to the dissociation of intramolecular hydrogen bonds within PDAAM. Therefore, the volume fraction of the hydrophobic block can be improved. Meanwhile, the intensity of the DMAA-inter C
O band decreases and that of the DMAA-free C
O band increases, suggesting the gradually weakening interaction between the hydrophilic PDMAA block and water. The PDMAA block undergoes a dehydration process, which depresses the stability of spherical micelles. It may lead to a decreasing volume fraction of the hydrophilic block. However, the driving force in the gelation process is still not clear.
3.3 2D-COS Analysis on IR Spectra
To further understand the molecular mechanism of structure evolution in the gelation process, it is necessary to clarify the variation order of each N–H, C–H, and C
O bands in the triblock copolymers. 2D-COS analysis was employed to analyze the temperature-dependent IR spectra,43 and the synchronous maps and the asynchronous maps due to N–H, C–H, and C
O bands are displayed in Fig. 3. NH groups are recognized by the asynchronous spectrum as the free NH groups and hydrogen-bonded at 3469 and 3349 cm−1, respectively.44 Meanwhile, four bands around 1708, 1656, 1647, and 1610 cm−1 attributed to C
O bands can be also found in the asynchronous spectrum. According to Noda's rule,45 the sequential orders of band variation of NH, CH, and CO groups are summarized in Table S1 (ESI†). It is very interesting that the four peaks around 3469, 1656, 3349, and 1647 cm−1, respectively attributed to free N–H bands, DAAM-free C
O, hydrogen-bonded N–H bands, and DAAM-intra C
O, start to change prior to DMAA-inter C
O (1610 cm−1). Then, the band at 2975 cm−1 changes, which represents the hydration of the PDAAM block. According to 2D-COS analysis, the dissociation of intramolecular hydrogen bonds within the hydrophobic PDAAM block firstly occurs, and then the variation of the hydrophilic PDMAA block takes place as a dehydration process. After that, the hydration of the PDAAM block occurs. Therefore, the dissociation of the hydrophobic PDAAM block might be the driving force for morphological transition and gelation.
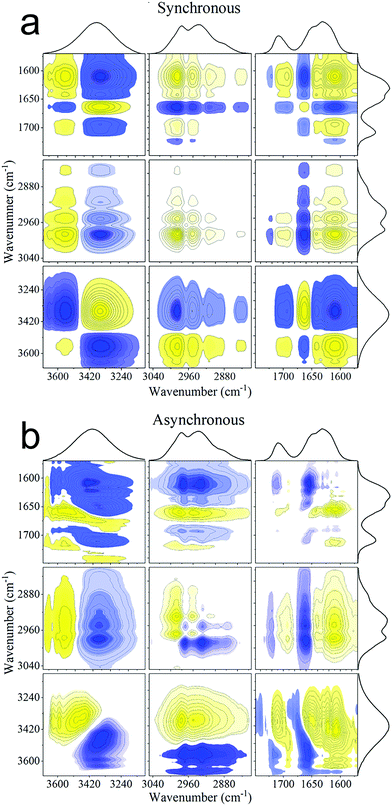 |
| Fig. 3 The synchronous (a) and asynchronous spectra (b) of ν(N–H), ν(C–H), and ν(C O) from 30 to 65 °C. | |
PDAAM is partially hydrated instead of completely hydrophobic. Thus, the influence on morphology from the hydrophobic block is greater than the influence from the hydrophilic block according to recent research by Borisov and co-workers,47 which is consistent with our 2D-COS analysis. The dissociation of intramolecular hydrogen bonds within the hydrophobic PDAAM block improves the stretching ability of polymer chains, which leads to a more hydrated core of copolymers. Then, the volume fraction of the hydrophobic core increases relative to that of the hydrophilic PDMAA stabilizer block, and the packing parameter P increases. Once the value of P is higher than 1/3, the self-assembled morphology transforms from spheres into worms, which manifests as gelation. The result that the dissociation of the hydrophobic PDAAM block plays a more significant role also explains the phase transition phenomenon of ABA-type triblock copolymers. That is adjusting the DP of the hydrophobic block can more precisely control the Tgel of copolymer solution than adjusting the DP of the hydrophilic block.33
3.4 Temperature-dependent NIR spectra
Temperature-dependent NIR spectroscopy was used to investigate the change in hydration of the copolymer during the morphological transition from spheres into worms. The structural change of the copolymer and the variation of water structures were analyzed. Fig. 4a shows the NIR spectra of the polymer solution (20 wt%) at different temperatures. The spectra in the range of 7400–5500 and 4950–4000 cm−1 corresponding the responses of OH, NH and CH were analyzed. A broad peak around 6900 cm−1 corresponds to the first overtone of OH, NH, and CH stretching vibration.48 Due to the overlapping, CWT with a filter of “Sym2” was adopted to enhance the resolution of the spectra; thus, the positive peaks in the raw spectra become negative ones. Fig. 4b depicts the transformed spectra from that in Fig. 4a. Obviously, two peaks at 7067 and 6824 cm−1 can be seen in Fig. 4b, which are assigned to the free OH (S0) in water molecules and the water structures with two (S2) hydrogen bonds, respectively.49 An increase and decrease of the two peaks can be observed, showing that the content of S0 increases and that of S2 decreases. It can be explained by the dissociation of hydrogen-bonded water with temperature.50 In Fig. 4b1, two peaks at 5954 and 5902 cm−1 can be found, which are related to CH3 groups of the copolymer.51 The intensity variation of the peak at 5954 cm−1 with temperature is plotted in Fig. S8 (ESI†). Two inflection points at 42 and 50 °C can be seen, which indicates the formation of the worms and the gelation, respectively. The result is also consistent with that of microrheological analysis. Another peak at 4901 cm−1 can be found in Fig. 4b2, compounding to NH groups of the copolymer.52 The redshift of the peak may indicate the breaking of the hydrogen bonds between the hydrophobic PDAAM blocks, which is consistent with the results of IR discussed above.
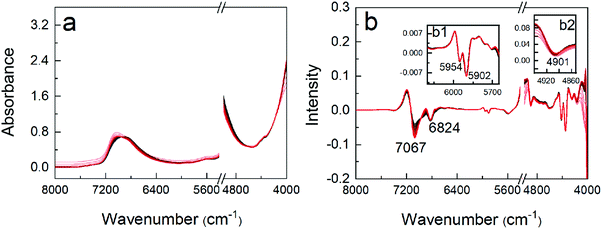 |
| Fig. 4 Measured (a) and transformed (b) NIR spectra with the magnified regions in 7200–5500 and 4950–4000 cm−1. The insets show the enlarged spectra in the range of 6400–5650 (b1), and 4950–4850 cm−1 (b2). | |
3.5 NPCA on NIR Spectra
For understanding the interaction of water and copolymer, NPCA was used to extract the information about the structural changes of the copolymer and the variation of water structures from temperature-dependent NIR spectra. The spectra measured from 30 to 68 °C with a duration of 20 min at each temperature for the solutions of different concentrations (20, 15, and 10 wt%) were analyzed. Fig. 5a and b show the results obtained by NPCA in the absorption range of CH groups, including the loadings of the first two principal components (PCs) and the corresponding scores in the time, concentration, and temperature dimensions, respectively. More than 99.97% of the spectral variances are accounted for by the two PCs. Two peaks at 5954/5902 (PC1) and 5900/5715 cm−1 (PC2) were obtained in Fig. 5a1 and b1, respectively, corresponding to the absorption of 2v3 and v2 + v3 of CH groups.58,59 For a clear comparison, the transformed spectra of homopolymer PDAAM (yellow curve) and PDAAM powder (purple curve) were also plotted in the two figures, respectively. PC1 is compared with the transformed spectra of homopolymer PDAAM, and similarities were found between them. The result indicates that PC1 contains spectral information of hydrated CH groups in PDAAM.60 In Fig. 5b1, the feature is similar to that in the purple curve, suggesting that the peaks in PC2 describe the unhydrated CH groups of the hydrophilic PDMAA block.
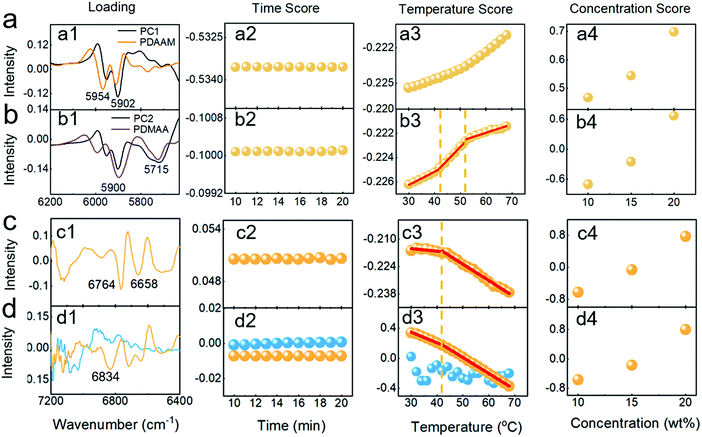 |
| Fig. 5 The first two PCs (a and b) in the region from 6200 to 5600 cm−1, and the third (c) and sixth (d) PCs in the region from 7200 to 6400 cm−1 obtained by NPCA from the spectra: the loading (a1–d1), time scores (a2–d2), temperature scores (a3–d3) and concentration scores (a4–d4); the lines in (b3–d3) were obtained by linear fitting in two or three stages. | |
Fig. 5a2 and b2 show the variation of the scores with time. The flat lines indicate that the structures of the hydrated hydrophobic PDAAM block and unhydrated hydrophilic PDMAA block are stable at a constant temperature. Fig. 5a3 shows the variation of temperature scores. An increase trend can be seen, which suggests that the content of hydrated CH groups of PDAAM increase during the transition. The change may be brought about by the dissociation of the intramolecular hydrogen bonds, making the PDAAM chains change from a compact state to an extended state. The increase in the rate can be seen at higher temperatures, indicating the formation of the gel. The result may be explained by that more water molecules interact with PDAAM, increasing the chance of the contact between the worms and promoting the gelation. Fig. 5b3 shows the variation of the temperature scores for the unhydrated hydrophilic PDMAA block. Two inflection points can be found, indicating the formation of the worm and the gel, respectively. Moreover, the content increase of unhydrated PDMAA indicates that the dehydration of the hydrophilic block may play an important role in the formation. Comparing the curves in three stages, it can be found that the dehydration at the first and the third stages is slower than that at the second stage. The result can be explained by that the contact area between the hydrophilic block and water is reduced due to the formation of worm-like micelles, making an acceleration of dehydration.53 After gelation, water molecules are confined in the network formed by the worms to make the water difficult to move, resulting in the decrease of the dehydration at the third stage.54Fig. 5a4–b4 display the variation of the two scores with concentration. Concentration scores increase with the concentration, which illustrates that spectral information contained in the PCs shown in Fig. 5 is positively correlated with the concentration. The spectral feature is derived from the interaction of the copolymer with water.
The variation of water structures was also studied by performing NPCA on the transformed spectra in the range of 7200–6400 cm−1. The PCs (yellow) obtained from the spectra of the copolymer solution and the PCs (blue) of pure water are drawn in Fig. S9 (ESI†). Obviously, new spectral features in PC3 and PC6 of copolymer solution can be observed and the results are drawn in Fig. 5c and d, respectively. Two peaks at 6764 and 6658 cm−1 can be found in Fig. 5c1, corresponding to the absorption of intramolecular hydrogen-bonded NH groups in the hydrophobic block.31Fig. 5d1 shows the loading of PC6; a peak at 6834 cm−1 can be found. According to the location of the peak,55 the peak arises from the absorption of the water molecules with two hydrogen bonds (S2). The spectral feature can only be found in the copolymer solution. Therefore, the peak may be brought about by the interaction of water and PDMAA/PDAAM, i.e., the S2 water molecule bridging the side chains of the PDMAA.56
The time and concentration scores shown in Fig. 5c2–d2 and c3–d3, respectively, demonstrate that the structures of hydrated NH groups and S2 water do not change at a constant temperature and the two structures are related to the copolymer. Fig. 5c3–d3 show the variation of the temperature scores for the two PCs. A slight decrease before 42 °C and an inflection point at the temperature can be seen in Fig. 5c3, indicating that the content of intramolecular hydrogen-bonded NH groups decreases with the formation of worms. The result further suggests that the intramolecular hydrogen bonds in the hydrophobic block stabilize the spherical micelle and the transition occurs after the hydrogen-bonding destroying. As for the temperature score in Fig. 5c3, an inflection point at 42 °C can also be seen in Fig. 5d3. The result may indicate that the destruction of the S2 water promotes the decrease of the relative space between the chains of the hydrophilic block, which contributes to the formation of worms.
3.6 Structural change during gelation transition
The structural change of the copolymer in an aqueous solution during the gelation is sketched in Fig. 6. Firstly, the copolymer exists stably in an aqueous solution in the form of the spherical micelles as shown at stage I. With the temperature increasing, the hydrophobic PDAAM block is gradually hydrated due to the weakening of the hydrogen bonds between the NH and CO groups in PDAAM, and the hydrophilic PDMAA block is dehydrated. When the temperature rises up to 42 °C, the transition from spherical to worm-like micelles occurs (stage II). The change may be brought by that the breaking of the intramolecular hydrogen bonds leads to the increase in relative volume of the PDAAM block, and more hydrated water molecules surround PDAAM. Above the temperature, more worms form and the contact area between the hydrophilic block and water decreases, resulting in the dehydration rate of the PDMAA block increasing. Finally, the gel network forms by the multiple contacts of lots of worms as shown at stage III, which traps the water molecules and makes the dehydration rate of the hydrophilic PDMAA block slow down.
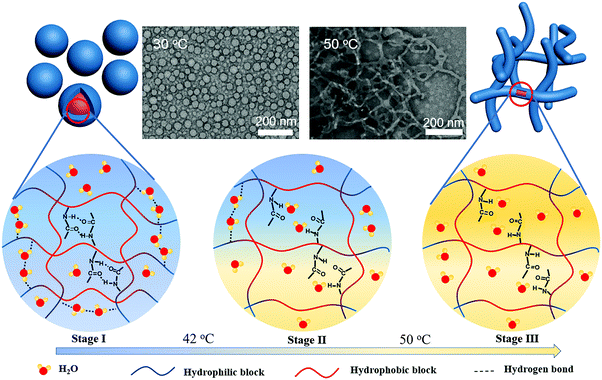 |
| Fig. 6 Schematic illustration of the PDMAA-b-PDAAM-b-PDMAA copolymer solution at molecular structural changes during the gelation process. | |
4. Conclusion
In this study, the temperature-dependent IR and NIR spectra are used to explore the molecular structure changes of an amphiphilic ABA-type copolymer of PDMAA-b-PDAAM-b-PDMAA in water. The copolymer shows a transition from sol to gel with a self-assembled morphology change from spheres to worms, even though both the homopolymers of PDMAA and PDAAM blocks do not have thermo-responsive properties. The gelation process is mainly determined by the dissociation of hydrogen bonds within the PDAAM block between the NH and the CO groups. Thus, the relative volume of the hydrophobic PDAAM block increases with temperature, resulting in the increase of packing parameter P and in turn the transformation from spherical micelles into worm-like micelles. The dehydration of the hydrophilic PDMAA block also occurs, which can be divided into three different stages with temperature. At stage I (low-temperature range) and stage III (high-temperature range), the dehydration rate is slow. However, at stage II (mid-temperature range), the dehydration is accelerated during the gelation, attributed to the decrease of the contact area between the hydrophilic PDMAA block and water with the morphology change. Moreover, the dehydration of the hydrophilic PDMAA block is caused by the destruction of the interaction between the S2 water molecules and the copolymer that acts as a bridging effect. Our research provides a unique insight into the gelation process of amphiphilic block copolymers. It also explains our previous research that adjusting the DP of the hydrophobic block can more accurately adjust the Tgel of the block copolymer solution.
Conflicts of interest
The authors declare that they have no known competing financial interests or personal relationships that could have appeared to influence the work reported in this paper.
Acknowledgements
This study was financially supported by the National Key Research and Development Program of China (Grant No. 2020YFE0100300). This study was also supported by the National Natural Science Foundation of China (No. 22174075), the Natural Science Foundation of Tianjin, China (20JCYBJC01480), the Frontiers Science Center for New Organic Matter, Nankai University (No. 63181206) and the Fundamental Research Funds for the Central Universities, Nankai University (No. 63211019).
References
- M. Parchehbaf-Kashani, H. Ansari, E. Mahmoudi, M. Barekat, M. Sepantafar, S. Rajabi and S. Pahlavan, ACS Appl. Bio Mater., 2021, 4, 4849–4861 CrossRef CAS PubMed.
- S. Cui, Y. Wei, Q. Bian, Y. Zhu, X. Chen, Y. Zhuang, M. Cai, J. Tang, L. Yu and J. Ding, ACS Appl. Mater. Interfaces, 2021, 13, 19778–19792 CrossRef CAS PubMed.
- J. Thiele, Y. Ma, S. M. Bruekers, S. Ma and W. T. Huck, Adv. Mater., 2014, 26, 125–148 CrossRef CAS PubMed.
- T. Wooster, S. Acquistapace, C. Mettraux, L. Donato and B. Dekkers, J. Colloid Interface Sci., 2019, 553, 308–319 CrossRef CAS PubMed.
- L. Zhang, R. Guo, M. Yang, X. Jiang and B. Liu, Adv. Mater., 2007, 19, 2988–2992 CrossRef CAS.
- Q. Wang, H. Tang and P. Wu, Langmuir, 2015, 31, 6497–6506 CrossRef CAS PubMed.
- D. Roy, W. L. Brooks and B. S. Sumerlin, Chem. Soc. Rev., 2013, 42, 7214–7243 RSC.
- S. Y. Khor, N. P. Truong, J. F. Quinn, M. R. Whittaker and T. Davis, ACS Macro Lett., 2017, 6, 1013–1019 CrossRef CAS PubMed.
- Y. Zhang, G. Han, M. Cao, T. Guo and W. Zhang, Macromolecules, 2018, 51, 4397–4406 CrossRef CAS.
- A. A. Cockram, T. J. Neal, M. J. Derry, O. O. Mykhaylyk, N. S. Williams, M. W. Murray, S. N. Emmett and S. P. Armes, Macromolecules, 2017, 50, 796–802 CrossRef CAS PubMed.
- N. J. Warren, M. J. Derry, O. O. Mykhaylyk, J. R. Lovett, L. P. Ratcliffe, V. Ladmiral, A. Blanazs, L. A. Fielding and S. P. Armes, Macromolecules, 2018, 51, 8357–8371 CrossRef CAS PubMed.
- M. Antonietti and S. Förster, Adv. Mater., 2003, 15, 1323–1333 CrossRef CAS.
- A. Blanazs, R. Verber, O. O. Mykhaylyk, A. J. Ryan, J. Z. Heath, C. I. Douglas and S. P. Armes, J. Am. Chem. Soc., 2012, 134, 9741–9748 CrossRef CAS PubMed.
- L. A. Fielding, J. A. Lane, M. J. Derry, O. O. Mykhaylyk and S. P. Armes, J. Am. Chem. Soc., 2014, 136, 5790–5798 CrossRef CAS PubMed.
- C. Dreiss, Soft Matter, 2007, 3, 956–970 RSC.
- J. R. Lovett, M. J. Derry, P. Yang, F. L. Hatton, N. J. Warren, P. W. Fowler and S. P. Armes, Chem. Sci., 2018, 9, 7138–7144 RSC.
- S. Cui, L. Yu and J. Ding, Macromolecules, 2019, 52, 3697–3715 CrossRef CAS.
- A. Zaccone, J. J. Crassous, B. Béri and M. Ballauff, Phys. Rev. Lett., 2011, 107, 168303 CrossRef PubMed.
- A. Zaccone, J. J. Zaccone and M. Ballauff, J. Chem. Phys., 2013, 138, 104908 CrossRef PubMed.
- S. Sun and P. Wu, J. Phys. Chem. B, 2011, 115, 11609–11618 CrossRef CAS PubMed.
- Q. Wang, H. Tang and P. Wu, J. Polym. Sci., Part B: Polym. Phys., 2016, 54, 385–396 CrossRef CAS.
- G. Wang and P. Wu, Langmuir, 2016, 32, 3728–3736 CrossRef CAS PubMed.
- Y. Zhou, H. Tang and P. Wu, Phys. Chem. Chem. Phys., 2015, 17, 25525–25535 RSC.
- L. Hou, K. Ma, Z. An and P. Wu, Macromolecules, 2014, 47, 1144–1154 CrossRef CAS.
- X. Cui, W. Cai and X. Shao, RSC Adv., 2016, 6, 105729–105736 RSC.
- L. Ma, X. Cui, W. Cai and X. Shao, Phys. Chem. Chem. Phys., 2018, 20, 20132–20140 RSC.
- S. Pan, H. Chung, M. A. Arnold and G. W. Small, Anal. Chem., 1996, 68, 1124–1135 CrossRef CAS PubMed.
- J. Lee, K. Chang, C.-H. Jun, R.-K. Cho, H. Chung and H. J. Lee, Chemom. Intell. Lab. Syst., 2015, 147, 139–146 CrossRef CAS.
- R. Tsenkova, J. Near Infrared Spectrosc., 2009, 17, 303–313 CrossRef CAS.
- E. Chatani, Y. Tsuchisaka, Y. Masuda and R. Tsenkova, PLoS One, 2014, 9, e101997 CrossRef PubMed.
- B. Ma, L. Wang, L. Han, W. Cai and X. Shao, Spectrochim. Acta, Part A, 2021, 253, 119573 CrossRef CAS PubMed.
- H. Shi, T. Qiu, H. D. Ou-Yang, H. Xu, Q. Lu, Y. Zheng, K. Liu, L. He, L. Guo and X. Li, J. Colloid Interface Sci., 2019, 545, 220–230 CrossRef CAS PubMed.
- J. Chen, T. Qiu, L. Guo, L. He and X. Li, Macromol. Rapid Commun., 2021, 2100024 CrossRef CAS PubMed.
- J. T. Lai, D. Filla and R. Shea, Macromolecules, 2002, 35, 6754–6756 CrossRef CAS.
- X. Shao and C. Ma, Chemom. Intell. Lab. Syst., 2003, 69, 157–165 CrossRef CAS.
- X. Cui, J. Zhang, W. Cai and X. Shao, Chemom. Intell. Lab. Syst., 2017, 170, 109–117 CrossRef CAS.
- X. Wang, X. Li, D. Xu, Y. Zhu, Y. Cao, X. Li and B. Sun, LWT – Food Sci. Technol., 2019, 109, 457–466 CrossRef CAS.
- E. Prince and E. Kumacheva, Nat. Rev. Mater., 2019, 4, 99–115 CrossRef.
- G. Su, T. Zhou, X. Liu and Y. Ma, Polym. Chem., 2017, 8, 865–878 RSC.
- Y. Park, C. Hashimoto, Y. Ozaki and Y. M. Jung, J. Mol. Struct., 2016, 1124, 144–150 CrossRef CAS.
- L. Hou and P. Wu, Langmuir, 2016, 18, 15593–15601 CAS.
- Y. Okada and F. Tanaka, Macromolecules, 2005, 38, 4465–4471 CrossRef CAS.
- I. Noda, Appl. Spectrosc., 1993, 47, 1329–1336 CrossRef CAS.
- W. Liang, J. Zhang, T. Zhou and A. Zhang, Vib. Spectrosc., 2016, 86, 160–172 CrossRef.
-
I. Noda and Y. Ozaki, Two-dimensional correlation spectroscopy: applications in vibrational and optical spectroscopy, John Wiley & Sons, 2005 Search PubMed.
- Y. Jiang, N. Xu, J. Han, Q. Yu, L. Guo, P. Gao, X. Lu and Y. Cai, Polym. Chem., 2015, 6, 4955–4965 RSC.
- A. M. Rumyantsev, F. A. M. Leermakers, E. B. Zhulina, I. I. Potemkin and O. V. Borisov, Langmuir, 2019, 35, 2680–2691 CrossRef CAS PubMed.
- B. Czarnik-Matusewicz and S. Pilorz, Vib. Spectrosc., 2006, 40, 235–245 CrossRef CAS.
- H. Maeda, Y. Ozaki, M. Tanaka, N. Hayashi and T. Kojima, J. Near Infrared Spectrosc., 1995, 3, 191–201 CrossRef CAS.
- A. A. Gowen, J. M. Amigo and R. Tsenkova, Anal. Chim. Acta, 2013, 759, 8–20 CrossRef CAS PubMed.
- X. Shao, X. Cui, Y. Liu, Z. Xia and W. Cai, ChemistrySelect, 2017, 2, 10027–10032 CrossRef CAS.
- P. Wu and H. W. Siesler, Macromol. Symp., 1999, 143, 323–336 CrossRef CAS.
- Y. Shen, B. Liu, J. Cui, J. Xiang, M. Liu, Y. Han and Y. Wang, J. Phys. Chem. Lett., 2020, 11, 7429–7437 CrossRef CAS PubMed.
- S. Le Caër, S. Pin, S. Esnouf, Q. Raffy, J. P. Renault, J.-B. Brubach, G. Creff and P. Roy, Phys. Chem. Chem. Phys., 2011, 13, 17658–17666 RSC.
- R. Tsenkova, J. Munćan, B. Pollner and Z. Kovacs, Front. Chem., 2018, 6, 363 CrossRef PubMed.
- A. Kundu, P. K. Verma and M. Cho, J. Phys. Chem. Lett., 2017, 8, 3040–3047 CrossRef CAS PubMed.
- D. Liu, W. Cai, L. Zhang, C. Boyer and J. Tan, Macromolecules, 2020, 53, 1212–1223 CrossRef CAS.
- D. H. Dagade and S. S. Barge, ChemistrySelect, 2017, 2, 11703–11712 CrossRef CAS.
- Y. Maeda, T. Kubota, H. Yamauchi, T. Nakaji and H. Kitano, Langmuir, 2007, 23, 11259–11265 CrossRef CAS PubMed.
- Y. Maeda, H. Yamauchi and T. Kubota, Langmuir, 2009, 25, 479–482 CrossRef CAS PubMed.
Footnotes |
† Electronic supplementary information (ESI) available: The synthetic route to PDMAA-b-PDAAM-b-PDMAA, 1H-NMR, and GPC analysis, definition of EI, TEM photos, illustration information about genetic algorithm, IR spectra of PDMAA and PDAAM, 2D-COS analysis of IR spectra, intensity plot of NIR spectra and NPCA from 7200 to 6400 cm−1. See DOI: https://doi.org/10.1039/d2cp00822j |
‡ These authors contributed equally to this work and should be considered co-first authors |
|
This journal is © the Owner Societies 2022 |
Click here to see how this site uses Cookies. View our privacy policy here.