Interfacial charge transfer complex formation between silver nanoparticles and aromatic amino acids†
Received
5th May 2022
, Accepted 6th June 2022
First published on 8th June 2022
Abstract
The optical properties of surface-modified silver nanoparticles (Ag NPs) with aromatic amino acids tryptophan (Trp) and histidine (His) were examined using the cluster model for density functional theory (DFT) and time-dependent density functional theory (TD-DFT) calculations. Also, the redistribution of electronic charges upon chemisorption of ligand molecules onto silver's surfaces is determined. The obtained theoretical data, on one side, undoubtedly indicate the the formation of an interfacial charge transfer (ICT) complex between silver and this type of ligand, and, on the other side, partial oxidation of surface silver atoms accompanied by an increase of electron density in ligand molecules. The ICT complex formation, based on noble metal nanoparticles, has never been reported previously to the best of our knowledge. The experimental spectroscopic measurements support the theoretical data. A new absorption band in the visible spectral range appears upon surface modification of Ag NPs, and, when exposed to air, oxidation of surface-modified Ag NPs is significantly faster than the oxidation of the unmodified ones.
1. Introduction
Silver nanoparticles (Ag NPs), free-standing and immobilized by various matrices, continuously attract the interest of the research community due to their potential applications in diverse fields. The applications of Ag NPs in microelectronics, optics, and sensing rely on their tunable optical properties in the visible spectral range and non-linear optical properties.1–5 On the other side, Ag NPs display antimicrobial activity, and their potential use in biomedical, food packaging, environmental, and textile areas is rapidly developing.6–10
Although the morphology-dependent (shape and size) optical properties of Ag NPs are in detail documented in the literature,1 open issues remain. In this contribution, we want to bring the attention to one old problem dated to the eighties of the last century. Herd et al.11 reported that the addition of pyridine and its derivative 4-dimethyl-amino-pyridine to silver colloids induced color changes in solutions due to the appearance of a new long-wavelength absorption band in the visible spectral region (λ > 500 nm). This group of authors attributed the observed optical changes to the aggregation of primary colloidal particles but pointed out that they do not coalesce, forming larger solid particles. Later on, in our contribution,12 the same effect was observed upon surface modification of Ag NPs with amino acid histidine and its aromatic residue imidazole. However, we believed that optical effects do not induce the aggregation or growth of silver particles because there was no increase in the scattering of the colloidal solutions upon the addition of nucleophilic molecules. On the other hand, the thorough investigation by Henglein's group concerning the influence of adsorption of sulfur-containing anionic species such as S2−,13,14 HSCH2COO−,15 and HS(CH2)2COO− onto Ag NPs,15 as well as CN−,14 indicated only an asymmetric broadening of the surface plasmon band into the long-wavelength region without the formation of a new absorption band.
Besides optical effects, the presence of nucleophilic molecules on silver nanoparticles’ surfaces induces significant changes in the reactivity of the nanoparticles. The Fermi level of surface-modified Ag NPs is shifted toward more negative potentials, and, as a consequence, they oxidize in the presence of electron acceptors or when exposed to oxygen.12–15 The enhanced oxidation of the surface-modified Ag NPs was explained by the partial oxidation of surface silver atoms, combined with increased electron density in the interior of a particle.13
The formation and optical properties of interfacial charge transfer (ICT) complexes between wide-bandgap-oxides, such as TiO2, and small colorless organic molecules are well-documented in the literature.16–18 Density functional theory (DFT) calculations are a frequent tool to support experimental findings.19–21 A recent study by Huseyinova et al.22 concerning the electrochemical synthesis of highly monodisperse Cu5 clusters in water without surfactants or protective agents intensified, considering their importance in catalytic processes, the use of DFT calculations to better understand the nature of subnanometer metallic clusters. So far, the properties of alloyed Ag–Au clusters23 and small metallic clusters, such as Ag5, deposited on metal oxide surfaces are evaluated using DFT and TD-DFT calculations.24–26 However, except for the analysis of the enhanced Raman scattering of a pyridine-tetrahedral Ag20 system using TD-DFT,27 to the best of our knowledge, the ICT complex formation between metallic particles and ligand molecules has neither been studied nor experimentally reported in the literature.
In this study, based on thorough DFT calculations, we provide the evidence that the ICT complex formation between Ag NPs and aromatic amino acids (tryptophan (Trp) and histidine (His)) takes place. In the light of this new finding, the explanation of optical properties and enhanced reactivity upon surface modification of Ag NPs is straightforward. The DFT data are supported experimentally with spectroscopic and transmission electron microscopy (TEM) characterization.
2. Results and discussion
2.1. Optical properties of surface-modified Ag NPs with amino acids
To elucidate the effect of chemisorbed amino acid molecules on the optical properties of Ag NPs, we used DFT and TD-DFT calculations. We parallelly analyzed the amino acids that are adsorbed onto the surface of Ag NPs, via the aromatic residues of indole (Trp) and imidazole (His) and compared them to those that do not possess aromatic side groups such as alanine (Ala). We presume that the coordination of Trp and His occurs via the deprotonated nitrogen atom of indole and imidazole, respectively, forming a direct N–Ag bond. This assumption is in accordance with the published DFT calculations concerning the charge-transfer complex between silver ions and 5-amino tetrazole28 and charge-transfer complexes between silver ions and various bipyridines.29 The optimized structures of the H/Ag19 cluster and three L/Ag19 complexes are presented in Fig. 1. The distances between the ligators’ N-atoms from the indole and imidazole rings and the corresponding Ag atoms are estimated to be 2.12 and 2.11 Å, respectively. The obtained lengths for the N–Ag bonds agree with the literature data29 for the charge-transfer complex between silver ions and bipyridines (0.22 nm). However, the optimized structure of the Ala/Ag19 cluster indicates that the coordination of alanine to the Ag NPs surface is through CO2 groups with estimated O–Ag distances of 2.36 and 2.32 Å (Fig. 1). On the other side, Manzoor et al.30 indicated that optimized geometries between non-aromatic amino acids (alanine and glycine) and small metallic clusters (Ag4 and Au4) involve an M–N bond rather than an M–O bond.
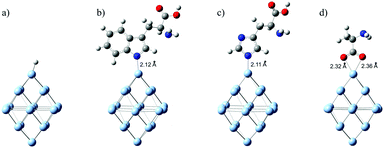 |
| Fig. 1 The optimized structures of (a) H/Ag19, (b) Trp/Ag19, (c) His/Ag19, and (d) Ala/Ag19 clusters. Atoms are represented as follows: gray: carbon; small white: hydrogen; red: oxygen; blue: nitrogen and pale blue: silver. | |
Because under the experimental conditions (pH ∼ 9), these amino acids are deprotonated, we calculated Gibbs free energy of adsorption considering amino acids as anions and Ag19 clusters as mono-cationic species to produce neutral complexes. The computed solvent-corrected Gibbs free energies of adsorption of Ala, His, and Trp to the Ag19 cluster are −0.35, −0.18, and −0.18 eV, respectively.
The electronic excitation spectra of L/Ag19 (L = Trp, His, Ala) complexes were calculated at the TD-CAM-B3LYP/def2-SVP level of theory as shown in Fig. 2. The spectra of the isolated amino acids are included in ESI† (Fig. S1) to demonstrate that their absorptions do not overlap with ICT transitions but rather lie in the UV region (<300 nm). The spectrum of the H/Ag19 cluster is shifted to the higher energies compared to the Trp/Ag19 and His/Ag19 spectra and does not considerably intersect with them (see the inset to Fig. 2), except for the broad absorption bands of amino acid–silver complexes that lie in the visible spectral region and arise from ICT transitions. At variance, the Ala/Ag19 spectrum almost coincides with that of H/Ag19, indicating the absence of ICT transitions. Ag19-based clusters do not exhibit plasmon resonance because the spectra evolve from molecular-like to plasmon-like shapes with the size of the nanocluster.31 Also, the literature data indicate that silver clusters consisting of at least 92 Ag-atoms display a plasmonic peak whose position depends on the size.32
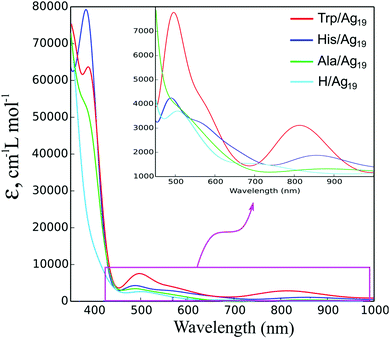 |
| Fig. 2 The electronic excitation spectra of H/Ag19 clusters and L/Ag19 complexes (L = Trp, His, Ala), calculated by convolution with full width at a half maximum of 2500 cm−1; inset: expanded spectra in the wavelength range 450–1000 nm. | |
The relevant data concerning the calculated excitation energies, oscillator strengths, and electronic configurations for L/Ag19 complexes (L = Trp, His) are collected in Table 1. The analysis of the wavefunctions and orbital contributions to the electronic transitions of the Trp/Ag19 system revealed that the two most pronounced transitions mostly arise from the ligand HOMO orbital to CBM+2 and CBM+5 of the cluster, 806.8 nm (f = 0.0184) and 502.1 nm (f = 0.0439), respectively. For the His/Ag19 complex, low and high energy peaks at 863 nm (f = 0.0045) and 489.2 nm (f = 0.0282) are mainly associated with electron promotion from the ligand HOMO to CBM+3 and CBM+7 of the cluster, respectively. In the case of the Ala/Ag19 complex, ICT transitions are not observed, except for small contributions of ligand's electron densities, which take part in some of the transition densities.
Table 1 Calculated excitation energies and the oscillator strength f of the five most pronounced ICT transitions for Trp/Ag19 and His/Ag19 complexes. Wavefunctions and contributions to the electronic transitions are presented in the last column. HOMOs denote ligand orbitals, while CBM and VBM denote Ag-centered orbitals
Complex |
Wavelength (nm) |
Oscillator strength |
Wave-function |coefficient|2 ≥ 10% |
Trp/Ag19 |
873.9 |
0.0049 |
HOMO → CBM+3 (50%) |
806.8 |
0.0184 |
HOMO → CBM+2 (46%) |
HOMO → CBM+1 (15%) |
593.6 |
0.0076 |
HOMO → CBM+5 (34%) |
VBM → CBM+4 (14%) |
557.3 |
0.0069 |
VBM → CBM+2 (86%) |
502.1 |
0.0439 |
HOMO → CBM+5 (28%) |
HOMO → CBM+7 (15%) |
His/Ag19 |
863.0 |
0.0045 |
HOMO → CBM (10%) |
HOMO → CBM+3 (62%) |
661.1 |
0.0042 |
HOMO → CBM+2 (44%) |
596.4 |
0.0049 |
HOMO → CBM+6 (35%) |
VBM → CBM+5 (23%) |
534.3 |
0.0083 |
VBM → CBM+3 (62%) |
489.2 |
0.0282 |
HOMO → CBM+8 (42%) |
VBM → CBM+3 (30%) |
The relevant orbitals, included in the pronounced transitions of Trp/Ag19 and His/Ag19 clusters, are presented in the ESI† (Fig. S2 and S3). Differences between L/Ag19 (L = Trp, His, Ala) clusters and the bare Ag cluster (H/Ag19) are noticeable in the spectral region from 350 to 400 nm. There are transitions in this region associated with HOMO−1 and HOMO−2 to the conduction band levels of L/Ag19. Also, there are transitions from hybrid valence band maximum (VBM) levels, which contain electron densities from amino acids (denoted with an asterisk in Fig. S2 and S3, ESI†) to the conduction band (CB). In contrast, the H/Ag19 cluster does not display such peaks. So, it is evident that the broad absorption bands of Trp/Ag and His/Ag predominantly arise from charge-transfer transitions from the HOMO orbitals of amino acids to the CB of silver nanoparticles. For the Ala/Ag19 system, HOMO → CBM and VBM-2 (with densities from Ala) to CBM+3/CBM+6 transitions are in the UV region at 363, 384, and 397 nm, respectively (Fig. S4, ESI†).
The calculated total and partial densities of states (DOS/PDOS) for Trp/Ag19 and His/Ag19 complexes are shown in Fig. 3. These DOS energy diagrams are like those calculated for the Ag clusters of similar sizes with several low energy surface states between the Fermi level and d-band (4d valence bands of silver).33 The HOMO levels of adsorbed Trp and His molecules are instilled near the approximated Fermi level of the Ag19 cluster (Fig. 3), while the HOMO level of Ala has located deeply inside the VB region (Fig. S5(B), ESI†). For comparison, the DOS energy diagram of the H/Ag19 cluster is presented in Fig. S5(A) (ESI†).
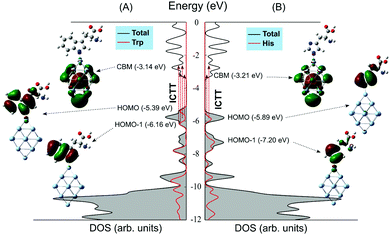 |
| Fig. 3 Total and partial densities of states (DOS) and spatial electron distributions of selected orbitals of ICT complexes between the Ag19 cluster and (A) Trp and (B) His amino acids are calculated at the CAM-B3LYP/def2-SVP level. Gray: carbon, white: hydrogen, blue: nitrogen, red: oxygen, bluish: silver atoms. | |
The absorption spectra and morphology of pristine Ag NPs, synthesized using NaBH4, are compared with surface-modified ones with amino acids (Trp, His, and Ala) to validate theoretical predictions. It is important to stress when comparing theoretical and experimental data that the experimental measurements were carried out on nanometer-sized silver particles and not on the theoretically modeled subnanometer-sized silver cluster. The neat silver sol has a surface plasmon resonance band at about 390 nm, typical for the nanometer-sized Ag particles (Fig. 4). Analysis of TEM data (ESI,† Fig. S6), taking into account about 150 particles, indicated that the pristine Ag particles are nearly spherical with an average diameter of 5.5 ± 1.6 nm. So, there is an agreement between microscopic and spectroscopic findings. On the other hand, the experimentally observed absorption bands of colloidal Trp/Ag and His/Ag particles lie in the visible spectral region (Fig. 4). Similar optical effects are described in the literature after surface-modification of noble metal particles, Ag34–36 and Au.37,38 However, in all these studies, the red absorption shift, caused by the functionalization of noble metal particles, is a consequence of the particle aggregation and formation of either chains or even networks. To determine whether or not the optical changes in Trp/Ag and His/Ag colloids are induced by the agglomeration of particles or the formation of ICT complexes, thorough TEM measurements were performed. Samples for microscopy measurements were taken 45 min after ligand addition to colloids, at the moment when optical changes are visible with the naked eye. The representative TEM image of Trp/Ag NPs is shown in Fig. 5(a). The TEM image of surface-modified Ag NPs with Trp does not differ from the neat ones (compare Fig. 5(a) with Fig. S6 in the ESI†). The size distribution of Ag NPs remained the same upon surface modification with Trp, so there is no growth or noticeable agglomeration accompanied by the appearance of short-chain structures such as doublets and triplets. In addition, the high-resolution lattice image of a single Trp/Ag particle is presented in Fig. 5(b), showing (111) fringes, while the selected-area diffraction pattern (SAED) revealed diffraction rings belonging to (111), (200), (220), (311) and (222) planes from the face-centered cubic phase of silver (Fig. 5(c)).
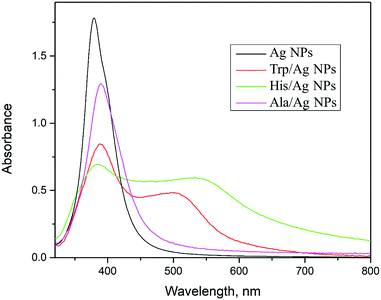 |
| Fig. 4 Absorption spectra of 1 × 10−4 M Ag colloid, before and after surface modification with amino acids (Trp, His, Ala); concentration of Trp, His, and Ala was 4.0 mM; spectra were recorded 45 min after surface modification. | |
 |
| Fig. 5 Typical TEM data from Trp/Ag colloid. The specimen was transferred to the TEM grid 45 min after surface modification: (a) bright-field TEM image; (b) high-resolution lattice image of a single Ag particle, showing (111) fringes; (c) selected-area diffraction pattern with prominent Ag peaks indexed. | |
Based on the TEM data, we believe that the appearance of absorption in the visible spectral range in the surface-modified Trp/Ag and His/Ag particles is a consequence of ICT complex formation and differs from the plasmon resonance of noble metal nanoparticles that may also induce similar optical effects. The experimentally observed absorption bands of colloidal Trp/Ag and His/Ag particles in the visible spectral region (Fig. 4) can be ascribed to the ICT transition from the amino acids donor levels (HOMO, HOMO−1) to the conduction band of Ag NPs, which is mainly composed of empty 5s and 5d states. Besides, the absence of the absorption band in the visible spectral region for the experimentally measured Ala/Ag spectrum (Fig. 4) is in accord with the calculated position of its donor levels (Fig. S5, ESI†), pinned at more negative potentials compared to Trp- and His-functionalized particles. The donor orbitals of Ala (HOMO and HOMO−1) have highly negative potentials (−7.27 and −7.91 eV, respectively), and consequently, they do not participate in ICT transitions.
To briefly conclude, the experimentally observed optical changes in surface-modified Ag NPs with aromatic amino acids are supported by DFT calculations, indicating the formation of ICT complexes. So far, DFT calculations, based on molecular complexes between ionic silver and ligands28,29 or pyridine-Ag20 clusters27 are applied to provide a better understanding of the surface-enhanced Raman scattering. Although the cluster model designed in this study (L/Ag19) to mimic the behavior of the metal–ligand interface is the largest one, there are a few drawbacks to be overcome. First, the L/Ag19 clusters do not exhibit plasmon resonance, and second, the long-wavelength excitation peak (>800 nm), predicted by DFT calculations, is not experimentally observed. Usage of the next larger in size meaningful cluster (Ag55) that we designed to understand the adsorption of carbohydrate molecules on Ag NPs39 takes enormous computational time and does not bring any novelty compared to the Ag19 cluster.
2.2. Enhanced reactivity of surface-modified Ag NPs with amino acids
To evaluate the explanation proposed a long time ago by the Henglein group13 for enhanced dissolution of surface-modified Ag NPs, based on the partial oxidation of surface Ag atoms upon functionalization with nucleophilic molecules, we performed the following calculations. Bader's method40 is applied to calculate the electronic charges in the atomic basins to quantify charge redistribution on atoms upon ligands’ chemisorption. For the three L/Ag19 complexes (L = Trp, His, Ala), we compared the values obtained for amino acids and Ag19 clusters before chemisorption with values obtained for the L/Ag19 systems. The topmost Ag atom loses electrons upon the chemisorption of all three ligands, as shown in Fig. 6. In the case of Trp/Ag19 and His/Ag19 complexes, the N atom is bound to the topmost Ag atom, while in the case of Ala/Ag19, two O atoms are bound to the topmost Ag atom. The coordination in Ala/Ag19 results in the most efficient charge transfer in all inspected cases of 0.32 electrons, redistributed between the two nearest O atoms. However, the charge redistribution between O atoms is not symmetric, as the O atom that is 0.05 Å closer to the topmost Ag atom gains 0.30 electrons, while the other gains only 0.12 electrons. In the case of Trp/Ag19 and His/Ag19 clusters, the topmost Ag atoms lose 0.23 and 0.24 electrons, respectively, close to the value obtained for the Ala/Ag19 system.
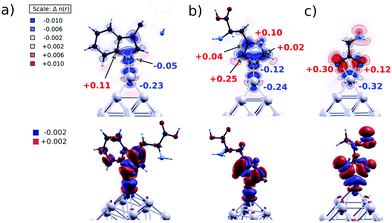 |
| Fig. 6 Electron densities induced upon chemisorption of (a) Trp, (b) His, and (c) Ala amino acids on the Ag19 cluster. The regions with an abundance (lack) of electrons are depicted in red (blue). The 3D plots of ±0.002 e Bohr−3 isosurfaces in the lower panel complement the in-plane plots from the upper panel. Numbers in the upper panel represent the changes of Bader charges in the atomic basins upon chemisorption. Dark gray: carbon, white: hydrogen, blue: nitrogen, red: oxygen, light gray: silver atoms. | |
Indeed, the absorption spectra of L/Ag colloids exposed to air change with time. Time-dependent absorption spectra of Trp/Ag colloids are shown in Fig. 7. Absorbance at the maximum of the surface plasmon resonance band (388 nm) and absorbance at the position of the ICT transition (540 nm) as a function of time are presented in the inset to Fig. 7. The time-dependent absorption spectra of His/Ag and Ala/Ag colloids are provided in the ESI† (Fig. S7 and S8). These are repeated measurements reported in our previous publication,12 and they fully agree. The simultaneous rise of absorbance of the ICT band (540 nm) and decrease of the surface plasmon resonance band (∼390 nm) in Trp/Ag and His/Ag colloids indicate that the ICT complex formation is a slow process occurring on a time scale smaller than one hour. In parallel, dissolution of Ag NPs takes place and prevails after about 30 min when both the plasmon resonance and ICT absorption start to diminish. Similar dissolution kinetics was also observed for Ala/Ag particles, indicating that complete oxidation of surface-modified Ag NPs with amino acids, under these conditions, occurs on a time scale of a few hours. On the other hand, the dissolution kinetics of pristine Ag NPs occurs on the time scale of days (see the inset to Fig. S8, ESI†). So, compared to unmodified Ag NPs, the dissolution kinetics of L/Ag NPs is significantly faster.
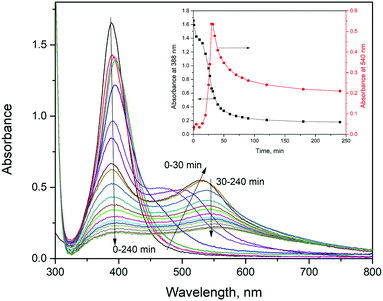 |
| Fig. 7 Absorption spectra of Trp/Ag colloids as a function of time; inset: absorbance at 388 and 540 nm as a function of time. | |
To summarize, the surface modification of Ag NPs with amino acids leads to redistribution of surface charges, regardless of whether the formation of an ICT complex takes place or not, and the net effect is partial oxidation of the surface Ag atoms prone to complete oxidation. In addition, Manzoor et al.30 indicated in their DFT study that co-adsorption of oxygen on complexes between Ag4 and aliphatic amino acids (alanine and glycine) is cooperative rather than competitive.
3. Experimental
3.1. DFT calculation
The Gaussian 09 program package is used for all density functional theory calculations, including geometry optimizations and TD-DFT calculations.41 The Ag19 cluster was employed as a model system for the DFT calculations to explain the optical properties and electronic structures of ICT complexes formed between aromatic amino acids (tryptophan (Trp) and histidine (His)) and silver nanoparticles. The octahedral silver cluster (Ag19) has Oh symmetry and was derived from the literature.42 The bare Ag19 cluster was capped with one H-atom on the top to achieve 20 electrons, a magic number for the cluster with an octahedral shape, and to keep calculations in restricted mode (closed-shell). The ground-state geometry optimization of L/Ag19 complexes was carried out in the gas phase by using the CAM-B3LYP (Coulomb-attenuating method) functional,43 which takes into account a long-range correction in combination with the split-valence polarized basis set (def2-SVP).44 The Ag19 structure with admolecules was fully optimized. This basis set gives very similar results to the much larger def2-TZVPP basis set for the H/Ag19 cluster. The frequency calculations were performed at the same level of theory to prove that the optimized structures present true minima. The electronic excitation spectra of all model systems (H/Ag19) clusters and L/Ag19 complexes (L = Trp, His, alanine (Ala)) were calculated at the same level of theory using the TD-DFT method to obtain the first 100 excitations.45 The convoluted electronic excitation spectra and the total and partial density of states (DOS/PDOS) were obtained using GaussSum software.46 The calculations of the electron densities induced by chemisorption were carried out using the Quantum ESPRESSO (QE) package based on plane waves and pseudopotentials.47,48 We used the GGA-PBE XC functional49 for these calculations and sampled the Brillouin zone only at the Γ-point. Although only the valence electrons were explicitly included, the employed pseudopotentials from PAW (Projector-Augmented Wave)50 sets allow full reconstruction of the total (core and valence) electron density. Plots of electron densities are produced using the XcrySDen package.51
The Gibbs free energies of adsorption were calculated as:
ΔGsol = Gcomplex − (GAg19+ + GAA−) |
where
Gcomplex,
GAg19+, and
GAA− are the Gibbs free energies of the corresponding surface complexes, free Ag
19 clusters as mono-cationic species, and free amino acid anions, respectively. Solvent effects were included by using gas-phase geometries and employing the SMD solvation model
52 with water as a solvent; this solvation energy was then added to the gas-phase results as single-point corrections. Negative values of Δ
Gsol correspond to favored adsorption.
3.2. Synthesis and characterization of surface-modified Ag NPs with aromatic amino acids
Silver colloids were prepared by reducing Ag+ with NaBH4, as described elsewhere.12 The silver colloids were combined with the ligand solutions (Trp, His, and Ala) to obtain surface-modified Ag NPs; typically, the final concentrations are 1 × 10−4 M Ag and 4.0 mM of ligand. A change of color from yellow to orange, red, and purple indicated the successful surface modification of Ag NPs.
Absorption spectra of silver colloids, pristine and surface-modified with amino acids (Trp, His, and Ala), were obtained as a function of time using a Shimadzu UV-Visible UV-2600 spectrophotometer. Dissolution of Ag NPs, pristine and surface-modified with Trp, His, and Ala, exposed to air at room temperature, was followed spectrophotometrically.
The JEOL JEM-2100 LaB6 instrument, operated at 200 kV, was used for transmission electron microscopy (TEM) imaging. TEM images were acquired using the Gatan Orius CCD camera at 2× binning. TEM samples were taken 45 min after the initiation of surface modification of pristine Ag NPs by amino acids.
4. Conclusions
The following conclusions can be discerned based on theoretical and experimental data. First, it is evident that the formation of an ICT complex is not exclusive to metal-oxides, but it can occur between Ag NPs and appropriately chosen ligands. Second, the ICT complex formation is induced by the coordinations of aromatic amino acids (Trp and His) with the silver's surfaces, similar to metal-oxide-based ICT complexes, where ligands are also aromatic molecules, preferably phenol derivatives. Third, the functionalization of Ag NPs with all studied amino acids (Trp, His, and Ala) induces redistribution of surface charges leading to enhanced dissolution rates of silver. Knowing how important is the release of silver ions for the antimicrobial activity of Ag NPs, this study offers a straightforward approach to overcoming some of the difficulties in the design of disinfection agents.
Author contributions
J. N. and D. S.: conceptualization. V. L., S. S. S. P. A. and D. S.: methodology, investigation, data curation, formal analysis, and validation; J. N.: supervision and resources; J. N. and D. S: writing the original draft; J. N., V. L. and D. S.: review and editing of the original draft.
Conflicts of interest
There are no conflicts to declare.
Acknowledgements
We want to express our gratitude to Professor Milivoj Belić for providing us the opportunity to perform DFT calculations using the RAAD2 supercomputer at the Texas A&M University at Qatar. Work at the Texas A&M University at Qatar was made possible by the NPRP11S-1126-170033 grant. This study was supported by the Ministry of Education, Science, and Technological Development of the Republic of Serbia (grant 451-03-1/2022-14/200017).
References
- P. Mulvaney, Surface plasmon spectroscopy of nanosized metal particles, Langmuir, 1996, 12, 788–800 CrossRef CAS.
- L. Yang, G. H. Li, J. G. Zhang, L. D. Zhang, Y. L. Liu and Q. M. Wang, Fine structure of the plasmon resonance absorption peak of Ag nanoparticles embedded in partially oxidized Si matrix, Appl. Phys. Lett., 2001, 78, 102–104 CrossRef CAS.
- J.-S. Lee, A. K. R. Lytton-Jean, S. J. Hurst and C. A. Mirkin, Silver nanoparticle-oligonucleotide conjugates based on DNA with triple cyclic disulfide moieties, Nano Lett., 2007, 7, 2112–2115 CrossRef CAS PubMed.
- S. Davidović, V. Lazić, I. Vukoje, J. Papan, S. P. Anhrenkiel and S. Dimitrijević,
et al., Dextran coated silver nanoparticles – Chemical sensor for selective cysteine detection, Colloids Surf., B, 2017, 160, 184–191 CrossRef PubMed.
- J. Li, H. Wang, Z. Li, Z. Su and Y. Zhu, Preparation and application of metal nanoparticals elaborated fiber sensors, Sensors, 2020, 20, 5155 CrossRef CAS PubMed.
- V. Ilić, Z. Šaponjić, V. Vodnik, B. Potkonjak, P. Jovančić and J. Nedeljković,
et al., The influence of silver content on antimicrobial activity and color of cotton fabrics functionalized with Ag nanoparticles, Carbohydr. Polym., 2009, 78, 564–569 CrossRef.
- S. Chernousova and M. Epple, Silver as antibacterial agent: Ion, nanoparticle, and metal, Angew. Chem., Int. Ed., 2013, 52, 1636–1653 CrossRef CAS PubMed.
- X.-F. Zhang, Z.-G. Liu, W. Shen and S. Gurunathan, Silver nanoparticles: Synthesis, characterization, properties, applications, and therapeutic approaches, Int. J. Mol. Sci., 2016, 17, 1534 CrossRef PubMed.
- A. Nešić, M. Gordić, S. Davidović, Ž. Radovanović, J. Nedeljković and I. Smirnova,
et al., Pectin-based nanocomposite aerogels for potential insulated food packaging application, Carbohydr. Polym., 2018, 195, 128–135 CrossRef PubMed.
- V. Lazić, V. Vivod, Z. Peršin, M. Stoiljković, I. S. Ratnayake and P. S. Ahrenkiel,
et al., Dextran-coated silver nanoparticles for improved barrier and controlled antimicrobial properties of nanocellulose films used in food packaging, Food Packag. Shelf Life, 2020, 26, 100575 CrossRef.
- S. M. Heard, F. Grieser, C. G. Barraclough and J. V. Sanders, The characterization of Ag sols by electron microscopy, optical absorption, and electrophoresis, J. Colloid Interface Sci., 1983, 93, 545–555 CrossRef CAS.
- V. V. Vuković and J. M. Nedeljković, Surface modification of nanometer-scale silver particles by imidazole, Langmuir, 1993, 9, 980–983 CrossRef.
- A. Henglein, T. Linnert and P. Mulvaney, Reduction of Ag+ in aqueous polyanion solution: Some properties and reactions of long-lived oligomeric silver clusters and metallic silver particles, Ber. Bunsen-Ges., 1990, 94, 1449–1457 CrossRef CAS.
- P. Mulvaney, T. Linnert and A. Henglein, Surface chemistry of colloidal silver in aqueous solution: Observations on chemisorption and reactivity, J. Phys. Chem., 1991, 95, 7843–7846 CrossRef CAS.
- A. Fojtik, P. Mulvaney, T. Linnert, M. Giersig and A. Henglein, Formation and reduction of semiconductor-like micelles of silver-carboxy-alkane-thiolates in aqueous solution, Ber. Bunsen-Ges. Phys. Chem., 1991, 95, 770–777 CrossRef CAS.
- I. A. Janković, Z. V. Šaponjić, M. I. Čomor and J. M. Nedeljković, Surface modification of colloidal TiO2 nanoparticles with bidentate benzene derivatives, J. Phys. Chem. C, 2009, 113, 12645–12652 CrossRef.
- S. Higashimoto, T. Nishi, M. Yasukawa, M. Azuma, Y. Sakata and H. Kobayashi, Photocatalysis of titanium dioxide modified by catechol-type interfacial surface complexes (ISC) with substitued groups, J. Catal., 2015, 329, 286–290 CrossRef CAS.
- J. Fujisawa, S. Matsumura and M. Hanaya, A single Ti–O–C linkage induces interfacial charge-transfer transitions between TiO2 and a π-conjugated molecule, Chem. Phys. Lett., 2016, 657, 172–176 CrossRef CAS.
- T. D. Savić, I. A. Janković, Z. V. Šaponjić, M. I. Čomor, D. Ž. Veljković and S. D. Zarić,
et al., Surface modification of anatase nanoparticles with fused catecholate type ligands: A combined DFT and experimental study of optical properties, Nanoscale, 2012, 4, 1612–1619 RSC.
- T. D. Savić, Z. V. Šaponjić, M. I. Čomor, J. M. Nedeljković, M. D. Dramićanin and M. G. Nikolić,
et al., Surface modification of anatase nanoparticles with fused ring salicylate-type ligands (3-hydroxy-2-naphtoic acids): A combined DFT and experimental study of optical properties, Nanoscale, 2013, 5, 7601–7612 RSC.
- T. D. Savić, M. I. Čomor, J. M. Nedeljković, D. Ž. Veljković, S. D. Zarić and V. M. Rakić,
et al., The effect of substituents on the surface modification of anatase nanoparticles with catecholate-type ligands: A combined DFT and experimental study, Phys. Chem. Chem. Phys., 2014, 16, 20796–20805 RSC.
- S. Huseyinova, J. Blanco, F. G. Requejo, J. M. Ramallo-López, M. C. Blanco and D. Buceta,
et al., Synthesis of highly stable surfactant-free Cu5 clusters in water, J. Phys. Chem. C, 2016, 120, 15902–15908 CrossRef CAS.
- G. Barcaro, M. Broyer, N. Durante, A. Fortunelli and M. Stener, Alloying effects on the optical properties of Ag–Au nanoclusters from TDDFT calculations, J. Phys. Chem. C, 2011, 115, 24085–24091 CrossRef CAS.
- M. P. de Lara-Castells, C. Cabrillo, D. A. Micha, A. O. Mitrushchenkov and T. Vazhappilly,
Ab initio design of light absorption through silver atomic cluster decoration of TiO2, Phys. Chem. Chem. Phys., 2018, 20, 19110–19119 RSC.
- P. López-Caballero, J. M. Ramallo-López, L. J. Giovanetti, D. Buceta, S. Miret-Artés and M. A. López-Quintela,
et al., Exploring the properties of Ag5–TiO2 interfaces: Stable surface polaron formation, UV-Vis optical response, and CO2 photoactivation, J. Mater. Chem. A, 2020, 8, 6842–6853 RSC.
- L. Sementa, M. Stener and A. Fortunelli, Optical activity of metal nanoclusters deposited on regular and doped oxide supports from first-principles simulations, Molecules, 2021, 26, 6961 CrossRef CAS PubMed.
- L. Zhao, L. Jensen and G. C. Schatz, Pyridine-Ag20 cluster: A model system for studying surface-enhanced Raman scattering, J. Am. Chem. Soc., 2006, 128, 2911–2919 CrossRef CAS PubMed.
- S. Thomas, N. Biswas, S. Venkateswaran, S. Kapoor, S. Naumov and T. Mukherjee, Studies on adsorption of 5-amino tetrazole on silver nanoparticles by SERS and DFT Calculations, J. Phys. Chem. A, 2005, 109, 9928–9934 CrossRef CAS PubMed.
- J. K. Lim and S.-W. Joo, Excitation-wavelength-dependent charge transfer resonance of bipyridines on silver nanoparticles: Surface-enhanced Raman scattering study, Surf. Interface Anal., 2007, 39, 684–690 CrossRef CAS.
- D. Manzoor and S. Pal, Enhanced interaction of molecular oxygen with amino acids complexes of silver and gold clusters, Indian J. Chem., Sect. A: Inorg., Bio-inorg., Phys., Theor. Anal. Chem., 2014, 54A, 996–1000 Search PubMed.
- C. M. Aikens, S. Li and G. C. Schatz, From discrete electronic states to plasmons: TDDFT optical absorption properties of Agn (n = 10, 20, 35, 56, 84, 120) tetrahedral clusters, J. Phys. Chem. C, 2008, 112, 11272–11279 CrossRef CAS.
- G. Barcaro, L. Sementa, A. Fortunelli and M. Stener, Optical properties of silver nanoshells from time-dependent density functional theory calculations, J. Phys. Chem. C, 2014, 118, 12450–12458 CrossRef CAS.
- N. A. Duchêne and A. Rochefort, Quantum size effects of agn clusters on carbon nanotubes, J. Phys. Chem. C, 2019, 123, 28769–28776 CrossRef.
- Y. Yang, J. Shi, T. Tanaka and M. Nogami, Self-assembled silver nanochains for surface-enhanced
Raman scattering, Langmuir, 2007, 23, 12042–12047 CrossRef CAS PubMed.
- I. S. Chae, S. W. Kang and Y. S. Kang, Olefin separation via charge transfer and dipole formation at the silver nanoparticle–tetracyanoquinoid interface, RSC Adv., 2014, 4, 30156–30161 RSC.
- S. S. Bayram, K. Lindfors and A. S. Blum, Tunable longitudinal modes in extended silver nanoparticle assemblies, Beilstein J. Nanotechnol., 2016, 7, 1219–1228 CrossRef CAS PubMed.
- S. Lin, M. Li, E. Dujardin, C. Girard and S. Mann, One-dimensional plasmon coupling by facile self-assembly of gold nanoparticles into branched chain networks, Adv. Mater., 2005, 17, 2553–2559 CrossRef CAS.
- Y. Yang, S. Matsubara, M. Nogami, J. Shi and W. Huang, One-dimensional self-assembly of gold nanoparticles for tunable surface plasmon resonance properties, Nanotechnology, 2006, 17, 2821–2827 CrossRef CAS.
- I. Vukoje, V. Lazić, D. Sredojević, M. M. Fernandes, S. Lanceros-Mendez and S. P. Ahrenkiel,
et al., Influence of glucose, sucrose, and dextran coatings on the stability and toxicity of silver nanoparticles, Int. J. Biol. Macromol., 2022, 194, 461–469 CrossRef CAS PubMed.
-
R. W. F. Bader, Atoms in Molecules: a Quantum Theory, Oxford University Press, New York, 2000 Search PubMed.
-
M. J. Frisch, et al., Gaussian 09, revision D.01, Gaussian Inc., Wallingford CT, 2009 Search PubMed.
- G.-T. Bae and C. M. Aikens, Time-dependent density functional theory studies of optical properties of ag nanoparticles: Octahedra, truncated octahedra, and icosahedra, J. Phys. Chem. C, 2012, 116, 10356–10367 CrossRef CAS.
- T. Yanai, D. P. Tew and N. C. Handy, A new hybrid exchange–correlation functional using the Coulomb-attenuating method (CAM-B3LYP), Chem. Phys. Lett., 2004, 393, 51–57 CrossRef CAS.
- F. Weigend and R. Ahlrichs, Balanced basis sets of split valence, triple zeta valence and quadruple zeta valence quality for H to Rn: Design and assessment of accuracy, Phys. Chem. Chem. Phys., 2005, 7, 3297–3305 RSC.
- M. A. L. Marques and E. K. U. Gross, Time-dependent density functional theory, Annu. Rev. Phys. Chem., 2004, 55, 427–455 CrossRef CAS PubMed.
- N. M. O’Boyle, A. L. Tenderholt and K. M. Langner, A Library for package-independent computational chemistry algorithms, J. Comput. Chem., 2008, 29, 839–845 CrossRef PubMed.
- P. Giannozzi,
et al., QUANTUM ESPRESSO: A modular and open-source software project for quantum simulations of materials, J. Phys.: Condens. Matter, 2009, 21, 395502 CrossRef PubMed.
- P. Giannozzi,
et al., Advanced capabilities for materials modelling with Quantum ESPRESSO, J. Phys.: Condens. Matter, 2017, 29, 465901 CrossRef CAS PubMed.
- J. P. Perdew, K. Burke and M. Ernzerhof, Generalized gradient approximation made simple, Phys. Rev. Lett., 1996, 77, 3865–3868 CrossRef CAS PubMed.
- P. E. Blöchl, Projector augmented-wave method, Phys. Rev. B: Condens. Matter Mater. Phys., 1994, 50, 17953–17979 CrossRef PubMed.
- A. Kokalj, XCrySDen-a new program for displaying crystalline structures and electron densities, J. Mol. Graphics Modell., 1999, 17, 176–179 CrossRef CAS PubMed.
- A. V. Marenich, C. J. Cramer and D. G. Truhlar, Universal solvation model based on solute electron density and on acontinuum model of the solvent defined by the bulk dielectric constant and atomic surface tensions, J. Phys. Chem. B, 2009, 113, 6378–6396 CrossRef CAS PubMed.
|
This journal is © the Owner Societies 2022 |
Click here to see how this site uses Cookies. View our privacy policy here.