Assessing the source of the photochemical formation of hydroxylating species from dissolved organic matter using model sensitizers†
Received
16th August 2021
, Accepted 29th November 2021
First published on 1st December 2021
Abstract
Dissolved organic matter (DOM) is ubiquitous in natural waters and can facilitate the chemical transformation of many contaminants through the photochemical production of reactive intermediates, such as singlet oxygen (1O2), excited triplet state DOM (3DOM*), and hydroxylating species (˙OH and other intermediates of similar reaction chemistry). The formation mechanism of most reactive intermediates is well understood, but this is not the case for the formation of hydroxylating species from DOM. To investigate this chemistry, DOM model sensitizers were irradiated with two different probe compounds (benzene and benzoic acid) at two irradiation wavelengths (254 and 320 nm). The ability of DOM model sensitizers to hydroxylate these arene probes was assessed by measuring rates of formation of the hydroxylated probe compounds (phenol and salicylic acid). Multiple classes of model sensitizers were tested, including quinones, hydroxybenzoic acids, aromatic ketones, and other triplet forming species. Of these classes of model sensitizers, only quinones and hydroxybenzoic acids had a hydroxylating capacity. Methanol quenching experiments were used to assess the reactivity of hydroxylating species. These results have several implications for the systems tested. First, they suggest that the hydroxylating intermediate produced from hydroxybenzoic acid photolysis may not be hydroxyl radical, but a different hydroxylating species. Also, these data prompted investigation of whether quinone photoproducts have a hydroxylating capacity. These results confirm that hydroxybenzoic acids and quinones are important to the photochemical production of hydroxylating species from DOM, but the mechanism by which this occurs for these classes of sensitizers is still elusive.
Environmental significance
The photochemistry of DOM is of interest to environmental scientists. Photochemical reactions within DOM form a series of reactive intermediates, including hydroxylating species such as ˙OH. However, the formation pathway for these species continues to be an active area of research. Here, we explore the mechanism by which these species are formed. These results will support current efforts in the community to better understand DOM photochemistry.
|
Introduction
Dissolved organic matter (DOM) is the primary light absorbing species in natural waters and has been shown to facilitate the chemical transformation of various contaminants through the production of reactive intermediates (RI).1–5 These RI, which include singlet oxygen (1O2), excited triplet state DOM (3DOM*), and hydroxylating species (˙OH and other intermediates of similar reaction chemistry), can react with many contaminants, making them important participants in the photodegradation of contaminants in natural waters and in engineered systems.6–12
The formation mechanisms for most RI are well understood,10,13,14 however, this is not the case for the formation of hydroxylating species from DOM. DOM photohydroxylation mechanisms can be differentiated experimentally by the involvement (or lack thereof) of hydrogen peroxide and/or dissolved oxygen.2 The H2O2-dependent pathway involves Fenton-like reactions1,15,16 and results in the direct formation of ˙OH, whereas the H2O2-independent pathway involves the production of hydroxylating species directly through photochemical reactions involving DOM. The precise mechanism by which hydroxylating species are produced from DOM photolysis (H2O2-independent pathway) has remained elusive, both in terms of the chemical moieties involved and identity of the oxidant. Although early studies attributed DOM photohydroxylation reactions to ˙OH,2,17 a study by Page et al.18 suggested that the production of hydroxylating species from DOM photolysis may not exclusively be ˙OH, prompting the acknowledgement of yet-unknown hydroxylating species that are also formed through photochemical reactions of DOM. These so-called ˙OH-like species have been invoked,19–21 which have lower, but not well-defined, reactivity compared to ˙OH.21 A primary difficulty in teasing apart the role of ˙OH versus so-called ˙OH-like species is that photohydroxylation reactions of many organic molecules result in identical products.13
The identity of ˙OH-like species is somewhat ambiguous, but the current understanding is that these species are either excited states or excited state complexes of chromophores within DOM. Previous studies have employed model sensitizers to assess potential mechanisms for the H2O2-independent pathway by which ˙OH-like species are produced.10,19,21–25 These sensitizers are selected based on their presence in DOM (e.g. quinones, aromatic ketones, aromatic acids) and whether they are photochemically active. Even if these groups are part of larger molecular weight structures, it is believed that the use of the model sensitizers is still warranted as it could be expected that these functionalities will still represent the primary photochemically active part of DOM. Notably, DOM absorbance can be interpreted using a superposition model or a charge-transfer model.26 The use of individual model sensitizers invokes the superposition model, which is justified in this study because the irradiation wavelengths chosen (254 and 320 nm) excite local states, not charge transfer states, which are hypothesized to occur mainly at 350 nm excitation or longer.27 Also, it should be noted that recent studies28,29 have shown that charge-transfer transitions may not be as prevalent as originally hypothesized.27
Similar to other RI, ˙OH and other hydroxylating species can be quantified using probe compounds.13,30 Some probe compounds that have been used for the detection of hydroxylating species from DOM photolysis are dimethyl sulfoxide, methane, benzene, nitrobenzene, benzoic acid, p-chlorobenzoic acid, and terephthalic acid.13,19,23,31–33 These probe compounds react either by hydrogen atom abstraction (e.g., methane) or hydroxylation (e.g. DMSO, arenes).13 Ideally, probe compounds are selective for reaction with ˙OH or ˙OH-like species, but there is evidence that ˙OH-like species react with some probe compounds in a way that is indistinguishable from ˙OH reaction.18,20,21 Due to their lack of selectivity, most probe compounds measure the hydroxylating capacity of a given system, which encompasses the measurement of both ˙OH and other hydroxylating species. Methane is the only probe thought to be selective for ˙OH, due to its high H–C bond dissociation energy. For this reason, methane has been used to distinguish between ˙OH and ˙OH-like species,18,19 but its use also has practical limitations, such as a limited and to be gaseous at room temperature.
Some sensitizers that have been shown to produce hydroxylating species upon irradiation include quinones and hydroxybenzoic acids.19,21,22,32,34 Early studies of the photochemical production of a hydroxylating intermediate from quinones utilized a spin trap probe, 5,5-dimethyl-1-pyrroline-1-oxide (DMPO).32,34–36 These studies claimed that the ˙OH is formed via H-atom abstraction when triplet quinones react with water. However, a number of studies have shown that there are other photochemical pathways that occur in the presence of triplet quinones that yield the same product as the reaction between DMPO and ˙OH.37–39 For this reason, conclusions from earlier studies that utilized DMPO as a probe compound are not reliable. Other studies have been performed using dimethyl sulfoxide (DMSO) as a probe compound to assess the chemical behavior of the hydroxylating intermediate produced from 2-methyl-p-benzoquinone photolysis by measuring the production of methyl radicals from the reaction of the hydroxylating intermediate with DMSO.19,21 However, a few studies have presented data that brings into question the use of DMSO as a probe for hydroxylating species.39,40 Von Sonntag et al. tested DMSO as a probe for hydroxylating species produced from p-benzoquinone photolysis and did not detect methyl radicals, suggesting that there is no oxidizing intermediate and that p-benzoquinone undergoes direct photodegradation to form its photoproducts.39 Interestingly, Gorner utilized DMSO as a probe and did observe the production of methyl radical, but attributed it to the reaction of the triplet quinone with DMSO.40 This contradictory data makes DMSO a questionable probe compound and is the reason DMSO was not used in this study. Additionally, Gan et al. used methane as a probe and established that the hydroxylating intermediate produced from 2-methyl-p-benzoquinone photolysis is not ˙OH.19 The same study hypothesized that the unknown hydroxylating intermediate is a quinone–water exciplex. Overall, the identity of the hydroxylating species produced from quinone photolysis and the mechanism by which this happens are still in question. Considering hydroxybenzoic acids, one previous study that utilized benzene and methane as a probe and quencher, respectively, concluded that this class of sensitizers produces ˙OH when photolyzed at wavelengths between 290 and 330 nm.22 This study suggested that, among other possible mechanisms, photooxidation followed by water addition and subsequent ˙OH cleavage may produce ˙OH.22
The production of hydroxylating species from DOM photolysis in surface waters and engineered systems is important because these chemical species can facilitate the transformation of contaminants. Assessing the reactivity of ˙OH-like species and how it differs from that of ˙OH is key for understanding and predicting the degradation pathways of chemical contaminants in water. This study focused on three objectives. First, the production of hydroxylating species during photolysis of various classes of model sensitizers was investigated. Second, the possibility that different mechanisms are responsible for this hydroxylating capacity was assessed by utilizing different arene probe compounds and methanol as a quencher. Third, apparent quantum yields of hydroxylated probe compound formation from the sensitizers in this study were compared to apparent ˙OH quantum yields from DOM photolysis. Overall, this work contributes to a larger understanding of the photochemistry of DOM in natural waters and engineered systems as it applies to the fate and transport of contaminants.
Materials and methods
Selection of model sensitizers
Sensitizers were selected to include chemical species that have been shown to produce hydroxylating species upon photolysis, such as quinones19,21,41,42 and hydroxybenzoic acids.43 Additional compounds were selected as a negative control that exhibit triplet state photochemistry but were not expected to produce hydroxylating species. Model sensitizers selected include p-benzoquinone (PBQ), anthraquinone-2-sulfonic acid (A2S), 2,6-dimethoxy-p-benzoquinone (DPBQ), 4-hydroxybenzoic acid (4HBA), 2,4-dihydroxybenzoic acid (DHBA), umbelliferone (UMI), trans-cinnamic acid (TCA), 4-benzoylbenzoic acid (4BA), and 3-methoxyacetophenone (3MP). All sensitizers structures are presented in Fig. 1 with their corresponding triplet state energy and triplet state one electron reduction potentials. Although A2S may not the best model for quinone structures in DOM because of its reactivity towards phenol (the product of benzene hydroxylation), it was selected because previous studies have used this compound to explore quinone photochemistry and model DOM photochemistry.23,34,44
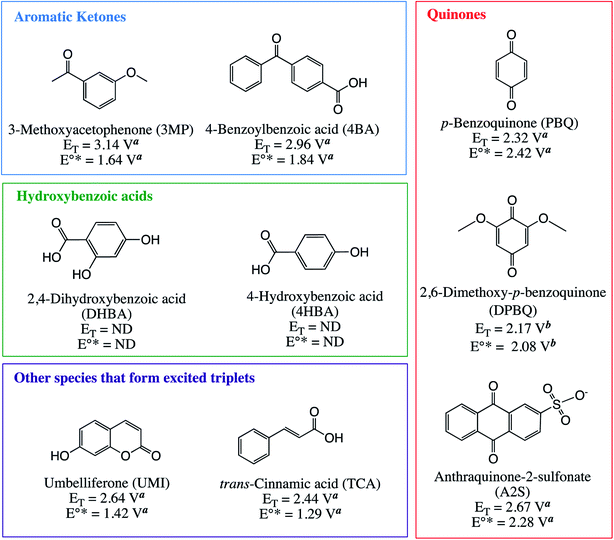 |
| Fig. 1 Model sensitizers used in this study, their structures, triplet state one electron reduction potentials (E°*), and triplet energies (ET). ND = no data, a McNeill and Canonica,10b Vaughan et al.2 | |
Chemicals and solutions
All chemicals were purchased from commercial sources and are listed in the ESI Table S1† with their respective CAS numbers and purities. Hydrogen peroxide, nitrate, and nitrite were used as sources of ˙OH. Solutions were prepared in MilliQ water, with the pH adjusted to 7.0 ± 0.1 using sodium hydroxide (∼20 mM). Samples were not prepared in buffers to avoid complicating the chemistry of the hydroxylating intermediates, which has been observed in systems containing p-benzoquinone in which the triplet p-benzoquinone reacts with phosphate to form an intermediate, p-benzoquinone-2-phosphate.39 During experiments performed with unbuffered solutions there was approximately 0.5 unit decrease in pH from the original pH of 7.0 ± 0.1. All model sensitizers besides UMI (pKa ∼ 7.8) would be unaffected by this pH change. In the case of UMI, a decrease in 0.5 pH units would change the fraction of deprotonated species from 0.13 to 0.044.
Quantification of hydroxylating species
Many probe compounds are available for detection of hydroxylating species, but probes relying on aromatic ring hydroxylation are among the most widely used in the aquatic photochemistry community.20,22,23,31 This was the main motivation for the choice of probes in this study, which were benzene and benzoic acid. Exploration of the chemistry of hydroxylating species with these two aromatic probes is important because of the frequency with which they are used to study the production of hydroxylating species from DOM. These results are important to previous studies that employ benzene or benzoic acid as probes for the production of hydroxylating species from DOM photolysis. The formation of hydroxylating species was detected by monitoring the hydroxylated product of the reaction between the probe compound and hydroxylating species. In the cases of benzoic acid and benzene, the formation of salicylic acid and phenol were monitored, respectively. Rate constants of ˙OH with benzene and benzoate are 7.8 × 109 and 5.9 × 109 M−1 s−1, respectively.7 It should be noted that we used benzene and benzoate as high concentration probes,13i.e., the majority (≥97%) of ˙OH produced reacted with benzene or benzoate (except for the experiments conducted in the presence of methanol as a quencher where the fraction of ˙OH produced reacting with the probe can be calculated using eqn (5), see below).
Analytical instrumentation
Analysis of salicylic acid and phenol was performed with an Agilent 1200 Series high performance liquid chromatography (HPLC) equipped with an Eclipse Plus XDB-C18 column with 4.6 × 150 mm dimensions and 5 μm particle size. The salicylic acid method employed an isocratic mobile phase of 30% acetonitrile and 70% pH 2.8, 10 mM H3PO4 with a flow rate of 1.0 mL min−1. Salicylic acid was quantified salicily acid using a fluorescence detector with excitation and emission wavelengths set to 320 nm and 410 nm, respectively. The typical retention time of salicylic acid in the system was approximately 6 min. The phenol method employed an isocratic mobile phase of 30% acetonitrile and 70% pH 2.8, 10 mM H3PO4 with a flow rate of 1.0 mL min−1. Phenol was quantified using a fluorescence detector with excitation and emission wavelengths set to 260 nm and 310 nm, respectively. The typical retention time of phenol in the system was approximately 5 min. Limit of quantification determined by the signal/noise method for phenol and salicylic acid were of 6 nM for both methods.
Irradiation experiments
A Rayonet RPR-100 (Southern New England Ultraviolet Company) was used for irradiations, employing either 254 or 320 nm lamps. The samples were placed in ≈9 mL stoppered quartz tubes (diameter 1.25 cm) on a carrousel rotating in the center of the photoreactor and were irradiated from the side. The 254 nm lamps emit light ranging from 249 nm to 258 nm. Quantum yield calculations for experiments using 254 nm lamps were treated as monochromatic. The 320 lamps emit light broadly centered around 313 nm (270–350 nm), with secondary emission peak extending up to 400 nm. Quantum yield calculations for experiments using 320 nm lamps were treated as polychromatic. Lamp spectra overlaid with absorption spectra of the sensitizers can be found in the ESI, Fig. S1.† The Rayonet RPR-100 employs a fan to control temperature. Photon irradiance was quantified daily using chemical actinometry: uridine actinometry was used for 254 nm irradiations45 and p-nitroanisole/pyridine (PNA/PYR) actinometry was used for 320 nm irradiations.46 More information about actinometry procedure can be found in ESI Text S1.† Irradiance spectra of the lamps were collected using a spectroradiometer and can be found in the ESI, Fig. S1 and S2.†
Irradiation experiments at 254 nm were performed with 1 mM benzoic acid as the probe compound and experiments at 320 nm were performed using 3 mM benzene as the probe compound. At these concentrations the probe compounds act as rate of formation probes, rendering the fraction of conversion of the probes to be negligible. For experiments performed using 254 nm lamps the concentration of sensitizers was adjusted to be optically matched at an initial optical density of 0.3. Concentrations of sensitizers in experiments normalizing absorbance to 0.3 ranged from approximately 10–100 μM. The total absorbance of these experimental solutions, including benzoic acid, was 0.83, leaving the sensitizers accounting for ∼37% of the light absorbed by the experimental solutions. For 254 nm experiments, solution temperature was not controlled due to the short irradiation times required with little temperature variation, between 23 and 28 °C. For experiments performed using 320 nm lamps, sensitizers concentrations were 20 μM. Longer irradiation times were required for 320 nm experiments and a shift in temperature was observed as a result of longer irradiation time. For this reason, prior to experimentation the solution temperature was adjusted to 30 ± 3 °C using a water-jacketed Petri dish, which is the steady-state temperature of the photochemical reactor.
Two corrections were made to experimental data. First, because benzoic acid absorbs strongly at 254 nm (Fig. S2†), the light screening factor for these experimental solutions was calculated and quantum yields were corrected for light screening following the procedure by Wenk et al.4 The equation used for these calculations is as follows,
|  | (1) |
where
ελ,PC and
ελ,sens are the molar extinction coefficients for the PC and sensitizers in M
−1 cm
−1 and [PC] and [Sens] are the concentrations of PC and sensitizers in M. This resulted in a light screening factor of 0.43 applied to all solutions containing sensitizers normalized to absorbance of 0.3. Using benzene with the 320 nm lamps did not present the same issue, as benzene does not absorb strongly in the wavelength range emitted by these lamps, shown in ESI Fig. S2.
† Second, for all experiments with sensitizers, rates of formation of the hydroxylated probe compound were corrected for formation of the hydroxylated probe compound from the direct photolysis of the probe compound itself. Additionally, control experiments were performed with DHBA to determine whether direct photolysis of DHBA results in salicylic acid, chromatograms for HPLC analysis of these experimental solutions are shown in ESI Fig. S3.
† This data shows that the direct photolysis of DHBA does not produce salicylic acid and, therefore, will not affect the quantum yield results. For the experiments using 4HBA as photosensitizer and benzoate as the hydroxylating species probe, one consideration is that 4HBA is itself one of the reaction products between benzoate and hydroxylating species.
47 As 4HBA concentration decreased during irradiation (Fig. S5
†) we conclude that 4HBA formation from benzoate hydroxylation can be safely neglected in the experiments analysis.
It is important to note that experimental limitations required use of different probe compound at 254 nm and 320 nm irradiation. Specifically, salicylic acid absorbs in the wavelength range emitted by the 320 nm lamps (Fig. S2†) and undergoes non-negligible direct photolysis (Fig. S4†), which precluded use of 320 nm lamps with benzoic acid as a probe compound. At 254 nm, benzoic acid screens most of the incoming light, minimizing direct photolysis of the salicylic acid photoproduct. The hydroxylated product of benzene (phenol) does not absorb at 320 nm (Fig. S2†). For all experiments with sensitizers, rates of formation of the hydroxylated probe compound were corrected for the production of the hydroxylated probe compound from the direct photolysis of the probe compound itself.
Methanol was added as a quencher in some experiments to assess whether the ˙OH-like species reacts with methanol similarly to ˙OH. In these experiments, methanol was spiked into solutions prior to irradiation at concentrations ranging between 0 and 0.1 M. Methanol reacts with ˙OH with a second-order reaction rate constant of 9.7 × 108 M−1 s−1.7 It is hypothesized that the reaction between methanol and other hydroxylating species to be different than that of ˙OH because hydroxyl radicals are non-selective and react with many molecules at near diffusion-controlled rates. A similar method was used in a study of DOM photochemistry by Leresche et al.48
Reaction pathways involving methanol or triplet quinones have been shown to produce superoxide, a fraction of which will dismutate to H2O2, which can undergo direct photolysis to form ˙OH.49–52 Calculations were performed to estimate the rate of formation of ˙OH from these pathways considering both 254 and 320 nm irradiation (see ESI Text S2†), the highest resulting values being on an order of 10−12 M s−1 with specific values varying based on the concentration of H2O2. This value is ∼2–3 orders of magnitude lower than the rates of hydroxylated probe compound formation observed in this study (ESI Table S3†). Based on these calculations, H2O2 is not expected to contribute significantly to observed rates of formation of hydroxylated probe compounds.
Quantum yield analysis
In order to compare the photoreactivity of sensitizers, apparent ˙OH quantum yields were calculated. Quantum yields were calculated for both sensitizer/wavelength systems: benzoic acid with 254 nm light and benzene with 320 nm light. Apparent quantum yields were calculated as described by eqn (2) | 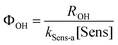 | (2) |
where R˙OH is the rate of formation of hydroxylating species in M s−1, ksens-a the specific rate of light absorption by sensitizers in s−1, and [Sens] is the sensitizer's concentration in M. The specific rate of light absorption of sensitizers was calculated using eqn (3). |  | (3) |
In eqn (3),
is the photon irradiance of the Rayonet photoreactor in Einstein cm−2 s−1, ρλ the relative spectral photon irradiance, εsens(λ) the molar absorption coefficient at the wavelength λ of the sensitizers in M−1 cm−1, εPC(λ) the molar absorption coefficient at the wavelength λ of the probe compound in M−1 cm−1, [PC] the concentration of the probe compound in M, and l the optical pathlength in cm. Additionally, R˙OH is defined as follows,
|  | (4) |
where
RPC-OH is the rate of formation of the hydroxylated probe compound and
Y˙OH the yield for the reaction of ˙OH with the probe compound.
The yield for the reaction of other hydroxylating species with the probe compound, Y˙OH-like, is unknown, so for these calculations, Y˙OH was used for all sensitizers. A reasonable hypothesis about whether Y˙OH-like is less than or greater than Y˙OH cannot be made without more information about the identity of the ˙OH-like intermediate and the mechanism by which it reacts with probe compounds. Additionally, this difference is difficult to quantitatively constrain due to the unknown quantum yield for the production of other hydroxylating species from sensitizers (apart from the use of probe compounds). Therefore, the quantum yields calculated in this study simply use Y˙OH as a benchmark for the expected value of Y˙OH-like. Even for ˙OH, a wide variety of yields exist in the literature for different arenes (e.g., ∼0.3–0.9 for Y˙OH of phenol from benzene), which may also be sensitive to solution conditions.53–57Y˙OH for the reaction of ˙OH with benzene to produce phenol, two more recent studies, Sun et al. and McKay and Rosario-Ortiz, determined this yield to be 0.693 ± 0.022 and 0.63 ± 0.07, respectively.20,43 These values are in good agreement with each other and fall within the aforementioned range for this parameter. For this study the value determined by McKay and Rosario-Ortiz, which utilized similar experimental conditions was used. Considering the formation salicylic acid from benzoic acid hydroxylation, a Y˙OH of 0.155 was used.58
Methanol quenching model
Methanol quenching was modeled by eqn (5), where f is the fraction of ˙OH reacting with the probe compound. |  | (5) |
where kPC,˙OH, kMeOH,˙OH, and ksens,˙OH are the second-order rate constants for respectively the probe compound, sensitizers, and methanol (MeOH) with ˙OH in M−1 s−1, respectively. [PC], [Sens], and [MeOH] are the concentrations of respectively the probe compound, sensitizers, and MeOH in M.
Results
Production of hydroxylating species from model sensitizers
The formation of hydroxylating species (˙OH and ˙OH-like species) from sensitizers was assessed using two different probe compounds with two irradiation wavelengths, benzoic acid with 254 nm irradiation and benzene with 320 nm irradiation. Fig. 2 shows the results for the experiments performed using benzoic acid with 254 nm irradiation. Fig. 3 shows results for the experiments using benzene with 320 nm irradiation. Formation rates for the data in these figures can be found in the ESI, Table S3.† All samples were interpreted as linear with respect to the formation of the hydroxylated probe compound with one exception: A2S with 320 nm irradiation using benzene as the probe compound because the production of phenol is clearly nonlinear (Fig. 3). Direct photolysis controls revealed that benzoic acid photolysis produces salicylic acid with a formation rate equal to 1.4 ± 0.08 nM s−1. Of the examined sensitizers, irradiation of quinones and hydroxybenzoic acids produced greater rates of salicylic acid formation (>3.0 nM s−1) than direct photolysis of benzoic acid, indicating that these sensitizers have a hydroxylating capacity. Irradiation of quinones produced salicylic acid at rates between 5.9 and 26 nM s−1, whereas irradiation of hydroxybenzoic acids produced salicylic acid at rates between 3.0 and 3.4 nM s−1. Conversely, the formation of salicylic acid in samples containing aromatic ketones and other triplet forming species was within the range of the direct photolysis control, suggesting that these sensitizers had a minimal hydroxylating capacity, if any.
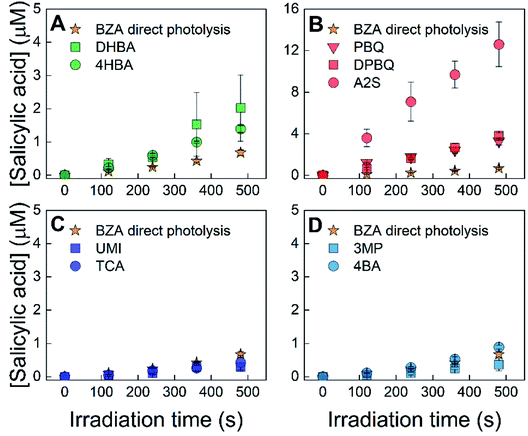 |
| Fig. 2 Production of salicylic acid with respect to time from the photolysis of sensitizers in the presence of 1 mM benzoic acid, irradiated with 254 nm lamps for 8 minutes. All samples were adjusted to pH 7.0 ± 0.1. The concentration of sensitizers was adjusted to be optically matched at an initial optical density of 0.3. Each subplot displays benzoic acid (BZA) direct photolysis data. (A) 2,4-Dihydroxybenzoic acid (DHBA), 4-hydroxybenzoic acid (4HBA), (B) p-benzoquinone (PBQ), 2,6-dimethoxy-p-benzoquinone (DPBQ), anthraquinone-2-sulfonate (A2S), (C) umbelliferone (UMI), trans-cinnamic acid (TCA), and (D) 3-methoxyacetophenone (3MP), 4-benzoylbenzoic acid (4BA). | |
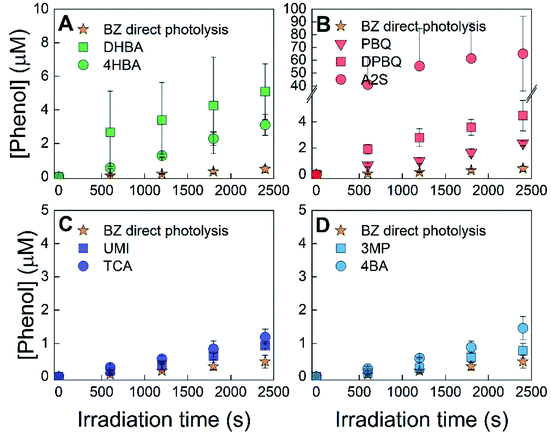 |
| Fig. 3 Production of phenol with respect to time from the photolysis of 20 μM sensitizers in the presence of 3 mM benzene, irradiated with 320 nm lamps for 40 minutes. All samples were adjusted to pH 7.0 ± 0.1. Each subplot displays benzene (BZ) direct photolysis data. (A) 2,4-Dihydroxybenzoic acid (DHBA), 4-hydroxybenzoic acid (4HBA), (B) p-benzoquinone (PBQ), 2,6-dimethoxy-p-benzoquinone (DPBQ), anthraquinone-2-sulfonate (A2S), (C) umbelliferone (UMI), trans-cinnamic acid (TCA), and (D) 3-methoxyacetophenone (3MP), 4-benzoylbenzoic acid (4BA). | |
Similar to the results obtained with benzoic acid at 254 nm, direct photolysis of benzene leads to its photohydroxylation, forming phenol (0.19 ± 0.04 nM s−1, Table S3†). As shown in Fig. 3 and ESI Table S3,† all sensitizers irradiated at 320 nm in the presence of benzene produced phenol at rates greater than could be accounted for by direct photolysis alone (between 0.39 and 2.2 nM s−1). Quinone and hydroxybenzoic acid sensitizers had rates of phenol formation sufficiently higher than that of the direct photolysis of benzene (all greater than 0.95 nM s−1), compared to the results obtained for the aromatic ketones, umbelliferone, or trans-cinnamic acid which were a maximum of 0.41 nM s−1 (for 4-benzoyl benzoic acid) greater than the rate of formation from benzene. All samples, except those containing A2S, were interpreted as linear with respect to the formation of phenol. In the case of A2S, the production of phenol was not linear, and the formation rate was not included in ESI Table S3.† This behavior can be attributed to the reaction of triplet A2S with phenol, as it has been previously established that phenol is oxidized by A2S.23 DPBQ may be able to oxidize phenol, but the reaction does not appear to occur significantly on the timeframe of the experiments. Leresche et al. observed a small (10%) degradation of salicylic acid in irradiation experiments of DOM, which contains quinone moieties, on a relatively longer timeframe (4 hours) than the condition used in the present study.48
Fig. 3B shows that the yield of phenol from A2S photolysis is greater than the original concentration of A2S in solution. There are a few possible explanations for this behavior. One study found that the photodecomposition of anthraquinones is fully reversible in the presence of oxygen while this is not true in the case of benzoquinones.59,60 This catalytic process could be contributing to increased yields of hydroxylating species in the case of anthraquinones. Additionally, some studies have observed an increase in the production of superoxide and hydrogen peroxide from anthraquinone-2,6-disulfonate and 9,10-anthraquinone derivatives in the presence of an electron donor.50,61 It is possible that benzene (which has an oxidation potential of ∼2.2 V)62 facilitates the catalytic production of hydrogen peroxide by continuously cycling A2S between reduced (A2S˙−) and oxidized (A2S) states without forming other stable quinone photoproducts. In the presence of an electron donor, if a high concentration of hydrogen peroxide is formed, an appreciable amount of ˙OH could then be formed from hydrogen peroxide photolysis. Ultimately, a combination of pathways that produce hydroxylating species are likely contributing to the photochemical behavior of A2S that is observed in Fig. 3B. Note that phenol, the product of the reaction of benzene with hydroxylating species is itself an electron donating species. A catalytical reaction of phenol with A2S would produces an exponential formation of phenol vs. time. As the opposite is observed (i.e., the formation rate of phenol is decreasing with time), if the reaction of phenol with A2S is producing hydroxylating species its overall yield should be ≤1.
Overall, the data presented in Fig. 2 and 3 indicate that quinones and hydroxybenzoic acids have a hydroxylating capacity. However, in contrast to the data from experiments using benzoic acid with 254 nm irradiation (Fig. 2), experiments using benzene with 320 nm irradiation (Fig. 3) show that the formation of phenol in systems containing aromatic ketones and other triplet forming species may not entirely be attributed to the direct photolysis of benzene. For the purposes of this study, aromatic ketones and other triplet forming species were not investigated further because their formation rates were barely outside of the uncertainties for the direct photolysis of benzene. Even if these sensitizers do participate in the production of hydroxylating species, it is to a much lesser extent than quinones and hydroxybenzoic acids.
Estimation of quantum yields for model sensitizers
Quantum yields were calculated for sensitizers (Table 1), with values at 320 nm irradiation being considered apparent due to the lamp's polychromatic nature. There are two complicating factors for calculating the quantum yields for the sensitizers. First, because the yields of hydroxylated probe compound from the reaction of hydroxylating species other than ˙OH are unknown, the yield for the reaction of the probe compound with ˙OH (Y˙OH) was used. It is expected that the reaction rate constants of these ˙OH-like species are different than that of ˙OH, which reacts with benzene and benzoic acid at near diffusion-controlled rates.7 For this reason, the data in Table 1 can be treated as estimates of the true quantum yield for these systems. Even given the uncertainty in yield, there is a benefit to presenting the data in terms of a quantum yield because this normalizes for differences in light absorption by the sensitizers. Additionally, calculating quantum yields for sensitizers also allows for the comparison to quantum yields measured for the formation of hydroxylating species from DOM.
Table 1 Quantum yields estimates for the production of hydroxylating species using benzoic acid and benzene as probe compound and irradiating at 254 and 320 nm, respectively. Values represent average of at least triplicate measurements with error representing one standard deviation. Values assume a Y˙OH value of 0.63 and 0.15, equal to that of ˙OH, for benzene and benzoic acid, respectively. ND = no quantum yield data for sensitizer because direct photodegradation of sensitizer occurred on a timescale too short for accurate quantum yield computation. Direct photodegradation data can be found in the ESI, Fig. S5 and S6
Model sensitizer |
Benzoic acid (254 nm irradiation) (×10−3) |
Benzene (320 nm irradiation) (×10−3) |
p-Benzoquinone (PBQ) |
ND |
ND |
Anthraquinone-2-sulfonate (A2S) |
47 ± 1.2 |
ND |
2,6-Dimethoxy-p-benzoquinone (DPBQ) |
17 ± 0.55 |
ND |
2,4-Dihydroxybenzoic acid (DHBA) |
6.3 ± 0.28 |
ND |
4-Hydroxybenzoic acid (4HBA) |
5.4 ± 0.13 |
11 ± 0.91 |
Second, there is the issue of the concentration of sensitizers changing with irradiation time. To evaluate this second issue, solutions of only sensitizers were irradiated under the same conditions described above and the absorbance spectra of those solutions were measured at different irradiation times. Changes in concentration of sensitizers were observed for quinones and hydroxybenzoic acids, which are the sensitizers that have been identified to have a hydroxylating capacity, at both irradiation wavelengths (ESI Fig. S5 and S6†). Regarding quinones, PBQ rapidly photodegrades under both wavelength conditions and A2S and DPBQ undergo rapid photodegradation with 320 nm irradiation. For hydroxybenzoic acids, Fig. S6† shows rapid photodegradation of DHBA at 320 nm. Quantum yields for these sensitizers at these wavelengths were not calculated.
Interestingly, although quinone degradation is significant, the formation rate of the hydroxylated probe compounds remains constant over our experimental timeframe in all cases but one (A2S), where it is expected that triplet A2S is reacting with phenol (Fig. 3B). One hypothesis is that the quinone and hydroxybenzoic acid photoproducts also have a hydroxylating capacity. For quinones, this was tested by performing experiments with hydroquinone, a quinone photoproduct,19 using 320 nm lamps with benzene as the probe compound. The 320 nm lamps emit at <300 nm, which overlaps with the absorption peak of hydroquinone (∼290 nm). These results can be found in the Fig. S7.† Production of phenol is observed in these experiments, indicating that hydroquinone has a hydroxylating capacity, with an estimated formation rate of 1.1 × 10−9 M−1 s−1, approximately an order of magnitude higher than the rate of formation of phenol from the direct photolysis of benzene (1.94 × 10−10 M−1 s−1). Based on these results, it is hypothesized that the quantum yields for quinones presented in this study are representative of the photochemistry of both quinones and quinone photoproducts, which could account for the linear rate of formation despite the fast photodegradation of quinones in these experimental conditions. The degradation of the hydroxybenzoic acids happens less rapidly than that of quinones but is still not negligible, especially in the case of DHBA with 320 nm irradiation (Fig. S6†). It is possible that the photoproducts of hydroxybenzoic acids also have a hydroxylating capacity, but the photoproducts of these compounds are not well established except for DHBA where a variety of carboxylated quinones and hydroxy-benzoic acids are known to be formed and to have a hydroxylating capacity.63 Based on the photochemical behavior observed for hydroquinone, it is hypothesized that quantum yields presented in this study are estimations for the quantum yields for the formation of hydroxylating species from a combination of sensitizers and their photoproducts.
Quinone quantum yields at 254 nm for A2S and DPBQ were (47 ± 1.2) × 10−3 and (17 ± 0.55) × 10−3, respectively. These values are approximately an order of magnitude lower than the quantum yield value reported by Gan et al., which is 0.37 ± 0.04.19 This difference may be due the use of different probe compounds, benzoic acid used in this study and DMSO used by Gan et al. Notably, a number have studies have concluded that DMSO is not a suitable probe for detecting hydroxylating species produced from quinone photolysis.39,40 One study did not detect a hydroxylating species from p-benzoquinone photolysis when using DMSO as a probe, while another did but attributed this to the direct reaction of the triplet quinone with DMSO.39,40 Taken as a whole, the existing data does not support the use of DMSO as a probe compound for the production of hydroxylating species from quinone photolysis. Consequently, the quantum yield measured by Gan et al. is questionable. Moreover, calculations performed to relate the absorbance by quinones in DOM to the quantum yields measured in this study validate the order of magnitude of the quantum yields presented here. For more information about these calculations, see the quinones and hydroxybenzoic acids contribution to DOM behavior section.
For hydroxybenzoic acids, variation in the quantum yields with wavelength was observed, (5.4 ± 0.13) × 10−3 at 254 nm and (11 ± 0.91) × 10−3 at 320 nm, for 4HBA (Table 1). There are two possible reasons for variation in quantum yields: the wavelength dependence of the photochemistry and the use of different probe compounds. In a study by Sun et al.,22 variation in quantum yield with wavelength was observed to range from (8.0–12.9) × 10−3 over 290–320 nm for 2,4-dihydroxybenzoic acid and (10.0–11.3) × 10−3 over 280–290 nm for 4-hydroxybenzoic acid when using the same probe compound, indicating that the photochemical pathway by which hydroxylating species are produced from this class of sensitizers is wavelength dependent. Additionally, the resulting quantum yield of (11 ± 0.91) × 10−3 for 4HBA using the 320 nm lamps (wavelength range 270 to 400 nm) agrees well with the results published by Sun et al., (10.0–11.3) × 10−3 over 280–290 nm, where benzene was also used as the probe compound.22
Methanol quenching
An important consideration to further understand the formation of hydroxylating species from DOM is the ability to differentiate between ˙OH and ˙OH-like species. Methanol was used as a quencher to investigate differences in the behavior of ˙OH and other hydroxylating species produced from quinones and hydroxybenzoic acids. These classes of sensitizers were selected based on data in Fig. 2 and 3, which established that both classes of sensitizers have a hydroxylating capacity. Methanol reacts with ˙OH, with a rate constant of 9.7 × 108 M−1 s−1,7 but the rate constant between methanol and other unknown hydroxylating species is unknown, although it is hypothesized to be different. Methanol quenching was modeled using the rate constant for ˙OH, represented as the fraction of ˙OH reacting with the probe compounds, f, as the concentration of methanol increases, represented by eqn (5). Experimental data were compared to the model, f, to assess similarities between the behavior of ˙OH and other hydroxylating species.
Fig. 4A shows normalized rates of hydroxylated probe compound formation for experiments for different sensitizers using benzoic acid and 254 nm irradiation at different methanol concentrations. Fig. 4B shows the same plot but for 320 nm irradiation. Methanol quenching of ˙OH produced from the photolysis of nitrate and nitrite was measured to confirm the modeled behavior of ˙OH.64 In experiments containing nitrate (Fig. 4A) and nitrite (Fig. 4B), there is good agreement between observed and calculated quenching (i.e., f calculated via eqn (5)), especially considering the range of reported values for the rate constant of the reaction between ˙OH and methanol varies by about 20%.7 Conversely, measured quenching for both quinones and hydroxybenzoic acids exhibited stark and inconsistent differences with calculated quenching in the two different probe/wavelength systems. For DHBA (a hydroxybenzoic acid hypothesized to produce free ˙OH) with benzoic acid and 254 nm irradiation, there is incomplete quenching of the production of salicylic acid with increasing concentrations of methanol (Fig. 4A, green circles). Conversely, for this same sensitizer but using benzene with 320 nm irradiation, methanol quenches the production of phenol to a greater degree than that modeled by ˙OH (Fig. 4B, green circles). Considering quinones, when using PBQ and A2S as the sensitizers, no quenching of salicylic acid production by methanol was observed in samples using benzoic acid with 254 nm irradiation. However, when using benzene with 320 nm irradiation, PBQ exhibited quenching of the formation of phenol that was similar to that of ˙OH (Fig. 4B, cyan circles).
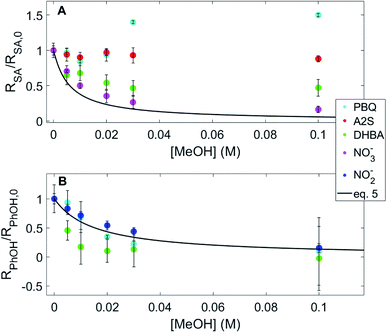 |
| Fig. 4 Effect of methanol addition (˙OH quencher) on the production of the hydroxylated probe compound. Left axis, normalized experimental rates of formation for the photolysis of 20 μM p-benzoquinone (PBQ), 20 μM anthraquinone-2-sulfonate (A2S), 20 μM 2,4-dihydroxybenzoic acid (DHBA), and 6 mM nitrate (NO3−) with a probe compound in the presence of 0, 0.005, 0.01, 0.02, 0.03, 0.1 M methanol. (A) 1 mM benzoic acid as probe compound, 254 nm lamps. (B) 3 mM benzene as probe compound, 320 nm lamps. | |
Discussion
Hydroxylating capacities of model sensitizers
Data in Fig. 2 and 3 are supportive of previous studies indicating that quinones and hydroxybenzoic acids have a hydroxylating capacity. One previous study has reported the detection of ˙OH from the photolysis of hydroxybenzoic acids.22 In this case, methane quenching experiments were supportive that the hydroxylating species responsible was free ˙OH. While several mechanisms were postulated such as photoionization,22 no definitive mechanism has been established for the production of ˙OH from hydroxybenzoic acids. The production of hydroxylating species from quinones has been studied more extensively than that of hydroxybenzoic acids. Studies have shown that the hydroxylating species produced by quinone photolysis is not ˙OH, but an ˙OH-like species proposed to be a triplet quinone–water exciplex.21 Other studies suggest that if triplet quinones do form an exciplex with water, this species proceeds directly to other photochemical degradation products of quinones, unless there is sufficient concentration of other reactive compounds.19,39,42 Additionally, previous work indicated that the quantum yield for the formation of phenol from benzene in the presence of PBQ had an activation energy near zero.20 In this case, this behavior was attributed to a multi-step process involving an exciplex.
Although data in Fig. 3C and D could be taken as support that aromatic ketones and other triplet forming species have a hydroxylating capacity, we hypothesize that this is not the case for two reasons. First, to the best of our knowledge there is no known photochemistry for aromatic ketones and the other triplet forming sensitizers that would lead to ˙OH or other hydroxylating species. Although prior studies have indicated that species having a very high triplet state one electron reduction potential (e.g., A2S) are capable of oxidizing hydroxide to form ˙OH,10,23,65 the aromatic ketones and other triplet forming species in this study (Fig. 1) have lower one electron reduction potential than A2S. Second, these data are not in agreement with the data from experiments using benzoic acid with 254 nm irradiation where salicylic acid formation was attributed solely to the direct photolysis of benzoic acid. Because the rates of formation for aromatic ketones and other triplet forming species are lower than that of the quinones and hydroxybenzoic acids (Table S3†) and the formation is thought to be dependent on the probe compound and irradiation wavelength, for the purposes of this study, they are not be considered significant contributors to the overall production of hydroxylating species from DOM. Overall, the data displayed in Fig. 2 and 3 is consistent with previous publications, confirming the presence of hydroxylating species from the photolysis of quinones and hydroxybenzoic acids.19–22,35,42
Methanol quenching: hydroxybenzoic acids
There are a few possible explanations for behavior observed in the DHBA methanol quenching data (Fig. 4). One is that the hydroxylating species produced from the photolysis of DHBA is not ˙OH (in contrast with a study by Sun et al.)22 and this ˙OH-like species has rate constants with benzene, benzoic acid, and methanol that are different than ˙OH, resulting in a trend in the methanol quenching data that differs from that of ˙OH. Interestingly, if this ˙OH-like species has a rate constant with benzene that is an order of magnitude lower than that of ˙OH, 7.80 × 108 M−1 s−1 instead of 7.80 × 109 M−1 s−1, then the experimental behavior of this hydroxylating species in the presence of benzene and methanol would match the model represented by eqn (5) which is shown in Fig. S8 in the ESI.† However, not only is this hypothesis at odds with more stringent methane quenching data reported by Sun et al.22 that indicated that the photolysis of hydroxybenzoic acids produces free ˙OH, but it also only explains the behavior of DHBA in systems using benzene with 320 nm irradiation, not for benzoic acid with 254 nm irradiation. Sun et al.22 used different quenchers and probe compound to determine whether the hydroxylating species detected was free ˙OH than those that were used in our study, including methane as a probe compound selective for ˙OH and formate as a competitor with benzene, which could be responsible for the conflicting results.22
Another possible explanation for the behavior in systems containing DHBA is that there are other photochemical pathways contributing to the formation and quenching of salicylic acid and phenol. One possible pathway that was tested, relevant to the use of benzoic acid with 254 nm irradiation, is production of salicylic acid from the direct photolysis of DHBA (Fig. S3†). If salicylic acid was being produced from the direct photolysis of DHBA as well as the reaction of the hydroxylating intermediate, that could explain the incomplete quenching of the formation of salicylic acid by methanol. However, no formation of salicylic acid was observed from the direct photolysis of DHBA (Fig. S3†), so the incomplete quenching of salicylic acid from the photolysis of DHBA is unaccounted for. Overall, the conflicting results described in Fig. 4 demonstrate that methanol cannot distinguish between ˙OH and ˙OH-like species from hydroxybenzoic acids.
Methanol quenching: quinones
Quinones (PBQ and A2S) exhibited no quenching of salicylic acid formation in experiments using benzoic acid with 254 nm irradiation upon methanol addition. Conversely, when using benzene with 320 nm irradiation, the quenching of phenol formation from quinone photolysis was similar to quenching observed for known free ˙OH sources (Fig. 4). There are a few possible explanations for this behavior. One hypothesis for the difference in the 254 nm and 320 nm quenching data for quinones is that there are different hydroxylating intermediates being produced at the two wavelengths, one of which does not react with methanol, resulting in the absence of quenching behavior. It is possible that at 254 nm the hydroxylating species produced from quinone photolysis is the ˙OH-like species and that this species is not quenched by methanol and that at 320 nm ˙OH is produced, which has a well-known rate constant with methanol.7 One study that utilized electron paramagnetic resonance (EPR) to investigate the reactivity of t-butanol with ˙OH produced from H2O2 and from PBQ photolysis observed that t-butanol was much more effective at reacting with ˙OH produced from H2O2 than ˙OH produced from PBQ photolysis, leading to the conclusion that the hydroxylating species observed in the EPR spectra is not ˙OH.39 This study supports the hypothesis that alcohols react more slowly with ˙OH-like species than with ˙OH and that differences in the methanol quenching data shown in this study could be attributed to the presence of different hydroxylating species at the two wavelengths tested.
The second hypothesis is that the lack of quenching in the system using benzoic acid with 254 nm irradiation is due to the formation of salicylic acid from one-electron oxidation of benzoic acid by the triplet quinone, with the sum of the production from the hydroxylating species and the one-electron oxidation of benzoic acid by the triplet quinone. However, the oxidation potential of benzoic acid calculated using the formula found in Jonsson et al.62 is 2.56 V (with an associated error that can be estimated to be ±0.2 V) and the reduction potential of triplet PBQ is 2.42 V,10 so if this reaction does happen it is anticipated to occur slowly. If the formation of salicylic acid in these experimental conditions is mostly due to the reaction of triplet quinones with benzoic acid, it is expected that there would still be only limited decrease in the quenching trend in Fig. 4. The decrease would be due to the quenching of hydroxylating species by methanol and to an eventual reaction of the triplet quinone with methanol.
It is important to note that studies have shown that the photoreduction of triplet quinones happens in the presence of hydrogen donors, such as alcohols.39 The reaction between triplet quinones and methanol is thought to produce a carbon centered radical, which can then react with ground state quinone to form formaldehyde.39 The formation of formaldehyde was not measured in this study. To assess the contribution of methanol to reaction pathways of triplet quinone a calculation for the fraction of triplet quinone reacting with methanol in a system containing water, benzoic acid, methanol, and oxygen was performed using eqn (6) and a figure displaying this data is presented in the ESI, Fig. S9,† using the following rate constants. The rate constant for the reaction of triplet p-benzoquinone and oxygen is reported to be 2 × 109 M−1 s−1 and the rate constant for the reaction between triplet p-benzoquinone and methanol is reported to be 4.2 × 107 M−1 s−1.39 The rate constant of triplet methyl-p-benzoquinone with water has been estimated to be 7.3 × 104 M−1 s−1.19 The rate constant for the reaction between benzoic acid and triplet quinones has not been quantified. For this reason, eqn (6) was calculated using values for kBA ranging from 104 to 109 M−1 s−1 to assess the potential effect of this reaction.
|  | (6) |
According to Fig. S9,† at methanol concentrations lower than 0.03 M approximately 20% or less of triplet quinone reacts with methanol. At the highest concentration of methanol, 0.1 M, no more than 50% of the triplet quinone reacts with methanol, depending on the rate constant for the reaction of benzoic acid and the triplet quinone. It is possible that complex chemistry involving methanol reactivity with triplet quinones in the presence of benzoic acid is contributing to a fraction of the lack of quenching observed in Fig. 4A. Ultimately, the conflicting quenching trends for quinones, the use of different probes at the two wavelengths used in this study, and the possibility of the reaction of methanol with triplet quinones leave the question of the chemistry of the hydroxylating intermediate produced from quinone photolysis in question.
Quinones and hydroxybenzoic acids contribution to DOM behavior
To assess the role of quinones as sensitizers for production of ˙OH and ˙OH-like species, the contribution of quinones and hydroxybenzoic acids to the fraction of light absorbed by DOM was calculated, using electrochemical data for Suwannee River fulvic acid (SRFA).66 Similar calculations were done in a study by Ma et al.67 Electron accepting capacities (EAC, μmole− gHS−1) were used to calculate the concentration of quinones (EAC × μmolquinone/2 μmole−) and electron donating capacities (EDC, μmole− gHS−1) were used to calculate the concentration of hydroxybenzoic acids (EDC × μmolhydroxybenzoicacid/2 μmole−). EDC and EAC measurements are dependent on solutions conditions, which might not be identical to solution conditions of DOM photochemistry experiments. The absorbance due to quinones and hydroxybenzoic acids was calculated by multiplying the above calculated concentrations by the molar absorption coefficient for these compounds measured in our study. The results of these calculations are shown in Fig. 5 and in Table S4† (for detailed calculations, see Text S3, ESI†). For the hydroxybenzoic acid sensitizers, 4HBA and DHBA, the fraction of light absorbed at 300 nm ranged from 0.2% by 4HBA to 47.9% by DHBA, whereas at 254 nm the fraction of light absorbed by 4HBA is 86.0% and the fraction of light absorbed by DHBA is 47.9%. Fig. 5A shows that the fraction of light absorbed by quinones depends on the specific compound and wavelength. As a surrogate for the variety of quinone structures in a given DOM sample eight quinones were used to approximate the total quinone absorbance, shown in Fig. 5B. In this approximation (Fig. 5B) quinone absorbance falls in the 1.5–22% range. For two quinone sensitizers in this study (PBQ and DPBQ), values ranged between nearly 31% (by PBQ at 246 nm) and <1% (by PBQ at 424 nm). For quinones whose absorption spectrum extends into the far UV and visible, the fraction of light absorbed at longer wavelengths increases (e.g., 15.2% by 1,4-napthoquinone at 342 nm). For DPBQ, the product of the observed quantum yield at 254 nm as an estimate for the quinone quantum yield (17 × 10−3, Table 1) and the fraction of light absorbed by the mixture of quinones at within the range of wavelengths shown in Fig. 5B (1.5–22%), at 254 nm the quantum yield would be ∼4.8 × 10−3. A study by Lester et al. showed that quantum yields for the formation of hydroxylating species from DOM photolysis at 254 nm is generally an order of magnitude higher than the quantum yield values at wavelengths greater than 300 nm, with the quantum yield for the formation of hydroxylating species from SRFA being 0.047.68
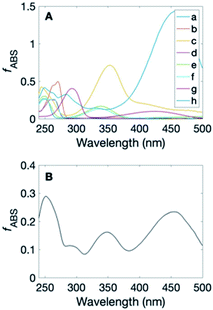 |
| Fig. 5 Fraction of light absorbed (fABS) by quinones in DOM. (A) Fraction of light absorbance by (a) p-benzoquinone, (b) 2,3,5,6-tetramethyl-1,4-benzoquinone, (c) 2,5-diphenyl-1,4-benzoquinone, (d) 2,5-di-tert-butyl-1,4-benzoquinone, (e) 1,4-naphthoquinone, (f) 2-methyl-1,4-naphthoquinone, (g) 2,6-dimethoxy-p-benzoquinone, and (h) alizarin, calculated individually, as though each account for entirety of quinone content in SRFA. (B) Fraction of absorbance by quinones (a–h) from above to account for quinone content of DOM in equal parts (12.5% each). | |
A recent study by Leresche et al. measuring the hydroxylating capacity of DOM before and after ozonation attributed the increase in quantum yields for hydroxylating species to the increase in quinone content of DOM post-oxidation.48 Our results support the hypothesis that 3DOM*, including quinones, are precursors of ˙OH and ˙OH-like species from DOM photolysis. However, another recent study suggested that 3DOM* is not a major precursor to ˙OH or ˙OH-like species based on correlations between RI quantum yields and the abundance of various formulas from ultra-high-resolution mass spectrometry.69 Instead, it was suggested that 1DOM*, charge-transfer states, or exciplexes involving photochemically excited DOM may be involved.69 This hypothesis is consistent with the role of hydroxybenzoic acids as sensitizers, but not with quinones. We contend that triplet quinones are likely responsible for some of the hydroxylation reactions observed for aromatic probe compounds given the known presence of these moieties in DOM. Additionally, our study demonstrates quinones do not necessarily need to be photostable in order to contribute significantly to the ˙OH or ˙OH-like production. For example, we observed similar rates of hydroxylation reactions from hydroquinone, a phenol photoproduct.
Conclusion
The goal of this work was to further understand the formation of ˙OH and ˙OH-like species from DOM photolysis. These results build on prior work that has established that quinones and hydroxybenzoic acids have a hydroxylating capacity.19,21,22,34,39,42 Aromatic ketones and other triplet forming species were selected to account for a class of sensitizers that are responsible for a portion of reactive triplet species in DOM. Aromatic ketones and other triplet forming species were expected to be negative controls for the production of hydroxylating intermediates.
The results presented in this study have several implications for measurement of hydroxylating species formation from DOM photolysis. Testing both benzoic acid and benzene as probe compound shed light on their respective effectiveness as probe compound for hydroxyl radials and, more generally, hydroxylating species. Additionally, the quenching of phenol formation from benzene during quinone photolysis by methanol indicates that methanol reacts with both ˙OH and ˙OH-like species. Although this result has been demonstrated in the literature, methanol has not been used explicitly to assess the reactivity of ˙OH-like species derived from triplet quinones.
The probes molecules used in this study are often used to estimate ˙OH steady-state concentration ([˙OH]ss) in surface waters without distinguishing between ˙OH and other hydroxylating species, leading to a potential over estimation of [˙OH]ss. This can potentially lead to an erroneous estimation of the ˙OH influence on the fate of micropollutants, e.g., if one uses an over evaluated [˙OH]ss and the second-order rate constant between ˙OH and the micropollutant to evaluate the rate of transformation of a micropollutant due to ˙OH.
Taken as a whole, the results of this study provide further support for the role of quinones and hydroxybenzoic acids as sensitizers for the production hydroxylating species from DOM photolysis. An important finding is that photoproducts from these species, especially in the case of quinones, likely have a hydroxylating capacity as well. Methanol quenching as a test of hydroxylating species' identity was inconclusive, indicating that other quenchers are needed to assess these differences in reactivity. Additionally, the quantum yields of ˙OH and ˙OH-like species from sensitizers photolysis are comparable to values measured for DOM by taking into account the fraction of light absorbed by quinones within DOM. These results indicate that triplet state quinones play a role in the hydroxylating capacity of DOM.
Conflicts of interest
The authors declare no competing financial interest.
Acknowledgements
Funding for this study came from the US National Science Foundation (CBET #1453906 and CHE #1808126) and through the University of Colorado Discovery Learning Apprenticeship.
References
- B. A. Southworth and B. M. Voelker, Hydroxyl radical production via the photo-fenton reaction in the presence of fulvic acid, Environ. Sci. Technol., 2003, 37(6), 1130–1136 CrossRef CAS PubMed.
- P. P. Vaughan and N. V. Blough, Photochemical formation of hydroxyl radical by constituents of natural waters, Environ. Sci. Technol., 1998, 32(19), 2947–2953 CrossRef.
- D. Vione, G. Falletti, V. Maurino, C. Minero, E. Pelizzetti, M. Malandrino, R. Ajassa, R. I. Olariu and C. Arsene, Sources and sinks of hydroxyl radicals upon irradiation of natural water samples, Environ. Sci. Technol., 2006, 40(12), 3775–3781 CrossRef CAS PubMed.
- J. Wenk, U. von Gunten and S. Canonica, Effect of dissolved organic matter on the transformation of contaminants induced by excited triplet states and the hydroxyl radical, Environ. Sci. Technol., 2011, 45(4), 1334–1340 CrossRef CAS PubMed.
- H. M. Xu, W. J. Cooper, J. Jung and W. H. Song, Photosensitized degradation of amoxicillin in natural organic matter isolate solutions, Water Res., 2011, 45(2), 632–638 CrossRef CAS PubMed.
- A. L. Boreen, W. A. Arnold and K. McNeill, Photodegradation of pharmaceuticals in the aquatic environment: A review, Aquat. Sci., 2003, 65(4), 320–341 CrossRef CAS.
- G. V. Buxton, C. L. Greenstock, W. P. Helman and A. B. Ross, Critical-review of rate constants for reactions of hydrated electrons, hydrogen-atoms and hydroxyl radicals (˙OH/˙O−) in aqueous-solution, J. Phys. Chem. Ref. Data, 1988, 17(2), 513–886 CrossRef CAS.
- D. E. Latch, B. L. Stender, J. L. Packer, W. A. Arnold and K. McNeill, Photochemical fate of pharmaceuticals in the environment: Cimetidine and ranitidine, Environ. Sci. Technol., 2003, 37(15), 3342–3350 CrossRef CAS PubMed.
- M. B. McConville, S. P. Mezyk and C. K. Remucal, Indirect photodegradation of the lampricides TFM and niclosamide, Environ. Sci.: Processes Impacts, 2017, 19(8), 1028–1039 RSC.
- K. McNeill and S. Canonica, Triplet state dissolved organic matter in aquatic photochemistry: Reaction mechanisms, substrate scope, and photophysical properties, Environ. Sci.: Processes Impacts, 2016, 18(11), 1381–1399 RSC.
- J. L. Packer, J. J. Werner, D. E. Latch, K. McNeill and W. A. Arnold, Photochemical fate of pharmaceuticals in the environment: Naproxen, diclofenac, clofibric acid, and ibuprofen, Aquat. Sci., 2003, 65(4), 342–351 CrossRef CAS.
- F. E. Scully and J. Hoigné, Rate constants for reactions of singlet oxygen with phenols and other compounds in water, Chemosphere, 1987, 16(4), 681–694 CrossRef CAS.
- F. L. Rosario-Ortiz and S. Canonica, Probe compounds to assess the photochemical activity of dissolved organic matter, Environ. Sci. Technol., 2016, 50(23), 12532–12547 CrossRef CAS PubMed.
- D. Vione, M. Minella, V. Maurino and C. Minero, Indirect photochemistry in sunlit surface waters: Photoinduced production of reactive transient species, Chemistry, 2014, 20(34), 10590–10606 CrossRef CAS PubMed.
- C. J. Miller, A. L. Rose and T. D. Waite, Hydroxyl radical production by H2O2-mediated oxidation of fe(II) complexed by suwannee river fulvic acid under circumneutral freshwater conditions, Environ. Sci. Technol., 2013, 47(2), 829–835 CrossRef CAS PubMed.
- E. M. White, P. P. Vaughan and R. G. Zepp, Role of the photo-fenton reaction in the production of hydroxyl radicals and photobleaching of colored dissolved organic matter in a coastal river of the southeastern united states, Aquat. Sci., 2003, 65(4), 402–414 CrossRef CAS.
- K. Mopper and X. L. Zhou, Hydroxyl radical photoproduction in the sea and its potential impact on marine processes, Science, 1990, 250(4981), 661–664 CrossRef CAS PubMed.
- S. E. Page, W. A. Arnold and K. McNeill, Assessing the contribution of free hydroxyl radical in organic matter-sensitized photohydroxylation reactions, Environ. Sci. Technol., 2011, 45(7), 2818–2825 CrossRef CAS PubMed.
- D. Gan, M. Jia, P. P. Vaughan, D. E. Falvey and N. V. Blough, Aqueous photochemistry of methyl-benzoquinone, J. Phys. Chem. A, 2008, 112(13), 2803–2812 CrossRef CAS PubMed.
- G. McKay and F. L. Rosario-Ortiz, Temperature dependence of the photochemical formation of hydroxyl radical from dissolved organic matter, Environ. Sci. Technol., 2015, 49(7), 4147–4154 CrossRef CAS PubMed.
- A. Pochon, P. P. Vaughan, D. Q. Gan, P. Vath, N. V. Blough and D. E. Falvey, Photochemical oxidation of water by 2-methyl-1,4-benzoquinone: Evidence against the formation of free hydroxyl radical, J. Phys. Chem. A, 2002, 106(12), 2889–2894 CrossRef CAS.
- L. N. Sun, J. G. Qian, N. V. Blough and K. Mopper, Insights into the photoproduction sites of hydroxyl radicals by dissolved organic matter in natural waters, Environ. Sci. Technol. Lett., 2015, 2(12), 352–356 CrossRef CAS.
- D. Vione, M. Ponzo, D. Bagnus, V. Maurino, C. Minero and M. E. Carlotti, Comparison of different probe molecules for the quantification of hydroxyl radicals in aqueous solution, Environ. Chem. Lett., 2010, 8(1), 95–100 CrossRef CAS.
- R. G. Zepp, G. L. Baughman and P. F. Schlotzhauer, Comparison of photochemical behavior of various humic substances in water: II. Photosensitized oxygenations, Chemosphere, 1981, 10, 119–126 CrossRef CAS.
- S. Canonica, U. Jans, K. Stemmler and J. Hoigné, Transformation kinetics of phenols in water: Photosensitization by dissolved natural organic material and aromatic ketones, Environ. Sci. Technol., 1995, 29(7), 1822–1831 CrossRef CAS PubMed.
- G. McKay, Emerging investigator series: Critical review of photophysical models for the optical and photochemical properties of dissolved organic matter, Environ. Sci.: Processes Impacts, 2020, 22(5), 1139–1165 RSC.
- C. M. Sharpless and N. V. Blough, The importance of charge-transfer interactions in determining chromophoric dissolved organic matter (CDOM) optical and photochemical properties, Environ. Sci.: Processes Impacts, 2014, 16(4), 654–671 RSC.
- E. A. Vialykh, G. McKay and F. L. Rosario-Ortiz, Computational assessment of the three-dimensional configuration of dissolved organic matter chromophores and influence on absorption spectra, Environ. Sci. Technol., 2020, 54(24), 15904–15913 CrossRef CAS PubMed.
- G. McKay, J. A. Korak, P. R. Erickson, D. E. Latch, K. McNeill and F. L. Rosario-Ortiz, The case against charge transfer interactions in dissolved organic matter photophysics, Environ. Sci. Technol., 2018, 52(2), 406–414 CrossRef CAS PubMed.
- J. M. Burns, W. J. Cooper, J. L. Ferry, D. W. King, B. P. DiMento, K. McNeill, C. J. Miller, W. L. Miller, B. M. Peake and S. A. Rusak,
et al. Methods for reactive oxygen species (ROS) detection in aqueous environments, Aquat. Sci., 2012, 74(4), 683–734 CrossRef CAS.
- M. M. Dong and F. L. Rosario-Ortiz, Photochemical formation of hydroxyl radical from effluent organic matter, Environ. Sci. Technol., 2012, 46(7), 3788–3794 CrossRef CAS PubMed.
- A. I. Ononye, A. R. McIntosh and J. R. Bolton, Mechanisms of the photochemistry of p-benzoquinone in aqueous solutions. 1. Spin trapping and flash photolysis electron paramagnetic resonance studies, J. Phys. Chem., 1986, 90(23), 6266–6270 CrossRef CAS.
- S. E. Page, W. A. Arnold and K. McNeill, Terephthalate as a probe for photochemically generated hydroxyl radical, J. Environ. Monit., 2010, 12(9), 1658–1665 RSC.
- A. E. Alegria, A. Ferrer and E. Sepulveda, Photochemistry of water-soluble quinones. Production of a water-derived spin adduct, Photochem. Photobiol., 1997, 66(4), 436–442 CrossRef CAS PubMed.
- A. E. Alegria, A. Ferrer, G. Santiago, E. Sepulveda and W. Flores, Photochemistry of water-soluble quinones. Production of the hydroxyl radical, singlet oxygen and the superoxide ion, J. Photochem. Photobiol., A, 1999, 127(1–3), 57–65 CrossRef CAS.
- A. I. Ononye and J. R. Bolton, Mechanism of the photochemistry of p-benzoquinone
in aqueous-solutions. 2. Optical flash-photolysis studies, J. Phys. Chem., 1986, 90(23), 6270–6274 CrossRef CAS.
- S. Pou, D. J. Hassett, B. E. Britigan, M. S. Cohen and G. M. Rosen, Problems associated with spin trapping oxygen-centered free-radicals in biological-systems, Anal. Biochem., 1989, 177(1), 1–6 CrossRef CAS PubMed.
- L. Eberson and O. Persson, Generation of acyloxyl spin adducts from N-tert-butyl-α-phenylnitrone (PBM) and 4,5-dihydro-5,5-dimethylpyrrole 1-oxide (DMPO) via nonconventional mechanisms, J. Chem. Soc., Perkin Trans. 2, 1997, 2(9), 1689–1696 RSC.
- J. von Sonntag, E. Mvula, K. Hildenbrand and C. von Sonntag, Photohydroxylation of 1,4-benzoquinone in aqueous solution revisited, Chem.–Eur. J., 2004, 10(2), 440–451 CrossRef CAS PubMed.
- H. Gorner, Photoreactions of p-quinones with dimethyl sulfide and dimethyl sulfoxide in aqueous acetonitrile, Photochem. Photobiol., 2006, 82(1), 71–77 CrossRef PubMed.
- S. M. Beck and L. E. Brus, Photo-oxidation of water by para-benzoquinone, J. Am. Chem. Soc., 1982, 104(4), 1103–1104 CrossRef CAS.
- H. Gorner, Photoprocesses of p-benzoquinones in aqueous solution, J. Phys. Chem. A, 2003, 107(51), 11587–11595 CrossRef.
- L. N. Sun, H. M. Chen, H. A. Abdulla and K. Mopper, Estimating hydroxyl radical photochemical formation rates in natural waters during long-term laboratory irradiation experiments, Environ. Sci.: Processes Impacts, 2014, 16(4), 757–763 RSC.
- I. Loeff, A. Treinin and H. Linschitz, Photochemistry of 9,10-anthraquinone-2-sulfonate in solution. 1. Intermediates and mechanism, J. Phys. Chem., 1983, 87(14), 2536–2544 CrossRef CAS.
- S. Jin, A. A. Mofidi and K. G. Linden, Polychromatic UV fluence measurement using chemical actinometry, biodosimetry, and mathematical techniques, J. Environ. Eng., 2006, 132(8), 831–841 CrossRef CAS.
- J. R. Laszakovits, S. M. Berg, B. G. Anderson, J. E. O’Brien, K. H. Wammer and C. M. Sharpless, p-Nitroanisole/pyridine and p-nitroacetophenone/pyridine actinometers revisited: Quantum yield in comparison to ferrioxalate, Environ. Sci. Technol. Lett., 2017, 4(1), 11–14 CrossRef CAS.
- X. L. Zhou and K. Mopper, Determination of photochemically produced hydroxyl radicals in seawater and freshwater, Mar. Chem., 1990, 30(1–3), 71–88 CrossRef CAS.
- F. Leresche, J. A. Torres-Ruiz, T. Kurtz, U. von Gunten and F. L. Rosario-Ortiz, Optical properties and photochemical production of hydroxyl radical and singlet oxygen after ozonation of dissolved organic matter, Environ. Sci.: Water Res. Technol., 2021, 7(2), 346–356 RSC.
- S. Garg, A. L. Rose and T. D. Waite, Photochemical production of superoxide and hydrogen peroxide from natural organic matter, Geochim. Cosmochim. Acta, 2011, 75(15), 4310–4320 CrossRef CAS.
- S. Garg, A. L. Rose and T. D. Waite, Production of reactive oxygen species on photolysis of dilute aqueous quinone solutions, Photochem. Photobiol., 2007, 83(4), 904–913 CrossRef CAS PubMed.
- S. Goldstein, D. Aschengrau, Y. Diamant and J. Rabani, Photolysis of aqueous H2O2: Quantum yield and applications for polychromatic UV actinometry in photoreactors, Environ. Sci. Technol., 2007, 41(21), 7486–7490 CrossRef CAS PubMed.
- J. Rabani, D. Klugroth and A. Henglein, Pulse radiolytic investigations of OHCH2O2 radicals, J. Phys. Chem., 1974, 78(21), 2089–2093 CrossRef CAS.
- T. Arakaki and B. C. Faust, Sources, sinks, and mechanisms of hydroxyl radical ˙OH photoproduction and consumption in authentic acidic continental cloud waters from Whiteface Mountain, New York: The role of the Fe(r) (r=II, III) photochemical cycle, J. Geophys. Res.: Atmos., 1998, 103(D3), 3487–3504 CrossRef CAS.
- I. Balakrishnan and M. P. Reddy, Effect of temperature on gamma-radiolysis of aqueous-solutions, J. Phys. Chem., 1972, 76(9), 1273–1279 CrossRef CAS.
- T. Charbouillot, M. Brigante, G. Mailhot, P. R. Maddigapu, C. Minero and D. Vione, Performance and selectivity of the terephthalic acid probe for ˙OH as a function of temperature, pH and composition of atmospherically relevant aqueous media, J. Photochem. Photobiol., A, 2011, 222(1), 70–76 CrossRef CAS.
- U. Deister, P. Warneck and C. Wurzinger, OH radicals generated by NO3− photolysis in aqueous-solution: competition kinetics and a study of the reaction OH + CH2(OH)SO3−, Ber. Bunsen-Ges., 1990, 94(5), 594–599 CrossRef CAS.
- J. Van Buren, C. Prasse, E. L. Marron, B. Skeel and D. L. Sedlak, Ring-cleavage products produced during the initial phase of oxidative treatment of alkyl-substituted aromatic compounds, Environ. Sci. Technol., 2020, 54(13), 8352–8361 CrossRef CAS PubMed.
- J. G. Qian, K. Mopper and D. J. Kieber, Photochemical production of the hydroxyl radical in antarctic waters, Deep Sea Res., Part I, 2001, 48(3), 741–759 CrossRef CAS.
- H. Gorner, Photoreduction of 9,10-anthraquinone derivatives, transient spectroscopy and effets of alcohols and amines on reactivity in solution, Photochem. Photobiol., 2003, 77(2), 171–179 CrossRef CAS PubMed.
- H. Gorner, Photoreduction of p-benzoquinones: Effects of alcohols and amines on the intermediates and reactivities in solution, Photochem. Photobiol., 2003, 78(5), 440–448 CrossRef PubMed.
- H. Gorner, Photoinduced oxygen uptake for 9,10-anthraquinone in air-saturated aqueous acetonitrile in the presence of formate, alcohols, ascorbic acid or amines, Photochem. Photobiol. Sci., 2006, 5(11), 1052–1058 CrossRef PubMed.
- M. Jonsson, J. Lind, T. Reitberger, T. E. Eriksen and G. Merenyi, Redox chemistry of substituted benzenes - the one-electron reduction potentials of methoxy-substituted benzene radical cations, J. Phys. Chem., 1993, 97(43), 11278–11282 CrossRef CAS.
- R. Tafer, M. Sleiman, A. Boulkamh and C. Richard, Photomineralization of aqueous salicylic acids. Photoproducts characterization and formation of light induced secondary OH precursors (LIS-OH), Water Res., 2016, 106, 496–506 CrossRef CAS PubMed.
- R. Zellner, M. Exner and H. Herrmann, Absolute OH quantum yields in the laser photolysis of nitrate, nitrite and dissolved H2O2 at 308 and 351 nm in the temperature range 278-353 K, J. Atmos. Chem., 1990, 10(4), 411–425 CrossRef CAS.
- B. Sur, M. Rolle, C. Minero, V. Maurino, D. Vione, M. Brigante and G. Mailhot, Formation of hydroxyl radicals by irradiated 1-nitronaphthalene (1NN): Oxidation of hydroxyl ions and water by the 1NN triplet state, Photochem. Photobiol. Sci., 2011, 10(11), 1817–1824 CrossRef CAS PubMed.
- M. Aeschbacher, M. Sander and R. P. Schwarzenbach, Novel electrochemical approach to assess the redox properties of humic substances, Environ. Sci. Technol., 2010, 44(1), 87–93 CrossRef CAS PubMed.
- J. H. Ma, R. Del Vecchio, K. S. Golanoski, E. S. Boyle and N. V. Blough, Optical properties of humic substances and CDOM: Effects of borohydride reduction, Environ. Sci. Technol., 2010, 44(14), 5395–5402 CrossRef CAS PubMed.
- Y. Lester, C. M. Sharpless, H. Mamane and K. G. Linden, Production of photooxidants by dissolved organic matter during UV water treatment, Environ. Sci. Technol., 2013, 47(20), 11726–11733 CrossRef CAS PubMed.
- S. M. Berg, Q. T. Whiting, J. A. Herrli, R. Winkels, K. H. Wammer and C. K. Remucal, The role of dissolved organic matter composition in determining photochemical reactivity at the molecular level, Environ. Sci. Technol., 2019, 53(20), 11725–11734 CrossRef CAS PubMed.
Footnote |
† Electronic supplementary information (ESI) available. See DOI: 10.1039/d1em00345c |
|
This journal is © The Royal Society of Chemistry 2022 |
Click here to see how this site uses Cookies. View our privacy policy here.