DOI:
10.1039/D1FO02171K
(Paper)
Food Funct., 2022,
13, 52-63
Enteromorpha prolifera polysaccharide–zinc complex modulates the immune response and alleviates LPS-induced intestinal inflammation via inhibiting the TLR4/NF-κB signaling pathway
Received
8th July 2021
, Accepted 1st October 2021
First published on 27th October 2021
Abstract
Enteromorpha prolifera polysaccharide–zinc (EP–Zn), a kind of polysaccharide–zinc complex, has been shown to improve the immune response and reduce the inflammatory factors in weaned piglets. Yet, the molecular mechanism remains unclear. The present study was conducted to investigate the immunomodulating activity and anti-inflammatory mechanism of EP–Zn in mice. Different doses (350 mg kg−1, 700 mg kg−1, 1050 mg kg−1 and 1400 mg kg−1) of EP–Zn were administered to C57BL/6J mice for 28 days. The results showed that under physiological conditions, 350 mg kg−1 EP–Zn stimulated cytokine (TNF-α, IL-1β, IL-6 and IL-10) secrection, regulated the intestinal microbiota, and reduced the levels of short-chain fatty acids (SCFAs) (acetic acid and propionic acid). In addition, in the LPS-induced inflammation model, EP–Zn pretreatment effectively alleviated LPS-induced shortening of colonic length and increased MPO and DAO contents, improved intestinal physical barrier function by modulating mucosal structure, and attenuated intestinal inflammation via inhibiting the TLR4/NF-κB signaling pathway. These findings suggested that EP–Zn exerted immunomodulatory and anti-inflammatory activities under physiological and inflammatory conditions, respectively.
1. Introduction
Inflammation is an innate immune defense mechanism used by the body to resist injuries, infection, and stress. Although appropriate inflammatory stimulation is beneficial for the host, excessive inflammation can cause tissue damage.1 As a major digestive and absorptive organ, the intestine provides an important defensive barrier.2,3 It has been documented that immunological challenges would lead to the destruction of intestinal tight junction barriers, and cause gut inflammation and disorders.4 Thus, a healthy intestinal function is significant for the growth and development of the host.
In the last decades, Enteromorpha prolifera has gained extensive attention and was widely applied in the functional foods and medical fields.5 According to the previous reports, Enteromorpha prolifera mainly contains carbohydrates,6,7 and is composed of rhamnose, glucuronic acid, and xylose.7Enteromorpha prolifera polysaccharide (EP) is a water-soluble sulfated polysaccharide extracted from Enteromorpha prolifera, mainly consisted of D-GlcUAp-α-(1 → 4)-3-sulfate-L-Rhap-β-(1 → 4)-D-Xylp-β-(1 → 4)-3-sulfate-L-Rha p units.7 Sulfated polysaccharide is the main component of EP, which has been reported to exhibit better anti-oxidant and immunomodulatory activities than the un-sulfated one.8,9 Besides, sulfated polysaccharides have a strong chelating ability that enables them to precisely combine with Fe, Zn, Cu, and other metal ions to reduce the generation of hydroxyl radicals, thereby improving the antioxidant and immune capacity.6,10,11 Recent studies have focused more on the immune-stimulating activities of EP,12,13 but less on the function of the EP–trace element complex. Since zinc (Zn) is the second most abundant trace element in the body, numerous studies have been conducted to examine its effects on innate and adaptive immunity.14 It has been reported that polysaccharide–zinc improved the water solubility, absorption rate, antioxidant capacity, and anti-inflammatory activity.15,16
Enteromorpha prolifera polysaccharide–zinc (EP–Zn) is a kind of seaweed polysaccharide organic trace element complex. Our recent study indicated that the replacement of antibiotics with EP–Zn in feed can improve the immune response, reduce the expression of nuclear factor-kappa B (NF-κB) and the release of downstream inflammatory factors, maintain the integrity of the intestinal barrier, and reduce the rate of diarrhea in weaned piglets.17 However, the molecular mechanism of how EP–Zn attenuates the inflammatory response and protects the intestine from bacterial and virus infections remains unclear. Therefore, the objective of the present study is to explore the immunomodulatory activities and anti-inflammatory mechanisms of EP–Zn in mice.
2. Materials and methods
2.1 Ethics statement
All the procedures and experimental design involved in this study were performed according to the Guidelines for Laboratory Animal Ethics Committee of the Chinese Academy of Sciences, and the experiments were approved by the Animal Care and Use Committee of Institute of Subtropical Agriculture, Chinese Academy of Sciences (No. ISA-2020-18).
2.2 Extraction, purification and structural features of EP
EP and EP–Zn were produced and provided by Qingdao Haida Biotechnology Co., Ltd (Qingdao, China). In brief, Enteromorpha prolifera was collected from the coast near Qingdao, China, in 2019. After being washed with tap water, it was air-dried, crushed, and kept at room temperature. Then, the Enteromorpha prolifera powdered sample was stirred and extracted with deionized water (1
:
10, w/v) and bioenzymes for 1.5 h. After being filtered and concentrated, the solution was precipitated with 80% ethanol (1
:
3, v/v). Finally, the precipitate part was washed with 80% ethanol and dried to obtain EP. EP was further purified using a Sephacryl S-100 column (2.6 × 60 cm) to obtain purified EP. The polysaccharide content is 71.35%, and the weight-average molecular weight is 4431 Da.
The monosaccharide composition of EP was determined by high performance liquid chromatography (HPLC) using rhamnose (Rha), arabinose (Ara), xylose (Xyl), mannose (Man), galactose (Gal), glucose (Glu), galacturonic (GalA) and glucuronic acid (GlcA) as monosaccharide standards.7 EP was identified as sulfated polysaccharides composed of rhamnose, glucuronic acid, xylose, galactose, and mannose at ratios of 1
:
1.25
:
0.06
:
0.02
:
0.01, respectively.
2.3 Preparation of the EP–Zn complex
The EP–Zn complex was prepared by dropping zinc chloride into EP solution at a ratio of 1
:
3 (w/w), and the pH was adjusted to 5.0–5.5 using NaOH (1 mol L−1) by heating at 50 °C for 2.5 h, then concentrated and alcohol precipitated to get EP–Zn. The contents of polysaccharide and zinc were 71.35% and 10.13%, respectively. The degree of polymerization was more than 85%, and the purity was 51%.
2.4 Fourier transform infrared (FT-IR) spectrum analysis of EP and EP–Zn
The infrared spectra of EP and EP–Zn were recorded using a Fourier transform infrared spectrometer (Thermo Electron Co., Waltham, MA, USA). The freeze-dried samples were ground with potassium bromide (KBr) and pressed into pellets for FT-IR measurement in the frequency range of 4000–400 cm−1 on the FT-IR spectrometer.
2.5 Animals and experimental design
Eight-week-old C57BL/6J male mice were purchased from Hunan Slack Jingda Experimental Animal Co., Ltd (Hunan, China) [certificate no. SCXK (Xiang) 2019-0004]. All the mice were housed at 22 ± 2 °C with a relative humidity of 50 ± 5%, 12 h light/12 h dark cycle, and free access to feed and water.
For the EP–Zn intervention study, 60 male C57BL/6J mice aged eight weeks were allowed to be acclimatized with a control chow diet for one week and then randomly divided into five groups, with twelve mice per group: (I) control group (NC) received a normal chow diet, and groups II to V received a normal chow diet supplemented with 350 mg kg−1, 700 mg kg−1, 1050 mg kg−1 and 1400 mg EP–Zn per kg diet, respectively. Weight and feed intake were recorded weekly. The intervention study was conducted for 28 days. Blood and colon contents were collected at the end of the experiment.
For the LPS injection study, 8-week-old mice were acclimatized with a control chow diet for one week and then randomly assigned into two treatments and fed with either a basal diet (n = 24) or a basal diet supplemented with 350 mg kg−1 EP–Zn (n = 12) for 28 days. At 08:00 am on day 29, mice supplemented with EP–Zn and half of the mice fed with a basal diet were intraperitoneally injected with LPS (0.5 mg kg−1, Escherichia coli serotype 055: B5; Sigma Chemical, Inc., St Louis, MO, USA) dissolved in a sterile 0.9% NaCl solution, and the rest of the mice were injected with the same volume of sterile 0.9% NaCl solution. Body weight changes were recorded before and after the LPS injection. At 24 h after treatment with LPS or saline, mice were euthanized and blood samples were collected. Thereafter, the lengths of the colon (from the colo-cecal junction to the anus) were measured and spleen weights were recorded. Ileum and colon tissue were immediately fixed in 4% paraformaldehyde solution for morphological observation. In addition, ileum samples and colon contents were collected and snap-frozen in liquid nitrogen and then stored at −80 °C until analysis.
2.6 Biochemical assays
The serum TNF-α, IL-1β, IL-6, IL-10 (Jiangsu Yutong Biological Technology Co., Ltd Meimian, China), myeloperoxidase (MPO), and diamine oxidase (DAO) (ZCIBIO Technology Co., Ltd, China) were measured using ELISA kits following the manufacturer's instructions.
2.7 Microbiota profiling
Genomic DNA was extracted from the colon content samples using an E.Z.N.A.® DNA Kit (Omega BioTek, Norcross, GA, USA) according to the manufacturer's recommendations. The V3–V4 region of the bacterial 16S rRNA gene was amplified with primers (F: 5′-ACTCCTACGGGAGGCAGCAG-3′ and R: 5′-GGACTACHVGGGTWTCTAAT-3′) by PCR. The PCR amplification was performed as follows: heating to 95 °C for 3 min, 35 cycles of denaturation at 95 °C for 30 s, annealing at 55 °C for 30 s and extension at 72 °C for 90 s, and single extension at 72 °C for 5 min, and finally at 4 °C. The PCR product was excised from 2% agarose gel and purified using the AxyPrep DNA Gel Extraction Kit (Axygen Biosciences, Union City, CA, USA) and quantified using a Quantus™ Fluorometer (Promega, USA). The sequencing and data analysis were performed on an Illumina MiSeq platform (Illumina, San Diego, USA) by Majorbio Bio-Pharm Technology Co. Ltd (Shanghai, China). Paired-end reads from the original DNA fragments were merged by FLASH. The resulting labels were assigned to the Operational Taxonomic Units (OTUs) with a threshold value of 97%. The complexity of species diversity (α-diversity) was analyzed by calculating Chao, Shannon, ACE, and Simpson indices. Variation in the community composition was measured by calculating the principal component analysis (PCA). In addition, the relative abundance of dominant bacteria at the genus levels was also analyzed.
2.8 Short-chain fatty acids
Colonic digesta samples were collected and the concentrations of short-chain fatty acids (SCFAs; acetic acid and propionic acid) were determined using an Agilent 6890 gas chromatograph (Agilent Technologies, Inc., Palo Alto, CA) according to the previous study.18 The Spearman correlation heatmap between the gut microbiota and SCFAs was constructed using R packages (V3.5.2).
2.9 Hematoxylin-eosin (HE) staining
Ileum and colon samples were fixed with 4% formaldehyde and were embedded in paraffin. Sections of 5 μm thickness were blocked and deparaffinized in xylene, and then stained with HE.
2.10 Quantitative reverse transcription PCR (RT-PCR) analysis
Total RNA was isolated from ileum tissue using TRIzol reagent (Invitrogen, Carlsbad, CA, USA). Then, each RNA sample was reverse-transcribed into cDNA using a Reverse Transcription Kit (Takara, Dalian, China). The qPCR was performed on a Roche LightCycler® 480II system using the primers listed in Table 1. Briefly, the reaction was carried out in a 10 μL solution containing 2 μL cDNA template, 5 μL of SYBR Green mix (Takara), 0.4 μL each of forward and reverse primers, and 2.2 μL of deionized H2O. The values of the target genes were normalized to β-actin and the relative expression level was analyzed by the 2−ΔΔCt method.
Table 1 Primers used in RT-qPCR
Gene |
Accession no. |
5′–3′ Primer sequence |
Product size (bp) |
TLR-4 |
NM_021297 |
F: CGCTGCCACCAGTTACAGAT |
263 |
R: CTTCAAGGGGTTGAAGCTCAG |
NF-κB |
NM_008689.2 |
F: GACACGACAGAATCCTCAGCATCC |
138 |
R: CCACCAGCAGCAGCAGACATG |
IL-1β |
NM_008361.4 |
F: ATGAAAGACGGCACACCCAC |
175 |
R: GCTTGTGCTCTGCTTGTGAG |
IL-6 |
NM_001314054.1 |
F: GCCTTCTTGGGACTGATGCT |
448 |
R: TGTGACTCCAGCTTATCTCTTGG |
IL-17 |
NM_010552.3 |
F: TACCTCAACCGTTCCACGTC |
119 |
R: TTTCCCTCCGCATTGACAC |
iNOS |
NM_001313922.1 |
F: CTGCAGCACTTGGATCAGGAACCTG |
311 |
R: GGGAGTAGCCTGTGTGCACCTGGAA |
β-Actin |
NM_007393.5 |
F: TGTCCACCTTCCAGCAGATGT |
101 |
R: AGCTCAGTAACAGTCCGCCTAGA |
2.11 Protein qualification using a Wes Simple Western system
Proteins were extracted from ileum samples with a radioimmunoprecipitation assay (RIPA) buffer (Beyotime Biotechnology, China) containing 1% protease inhibitor cocktail. After being centrifuged at 12
000g for 15 min, the total protein concentration was determined using the bicinchoninic acid (BCA) assay (Beyotime Biotechnology, China) according to the manufacturer's instructions. For protein qualification, a Wes Simple Western system (ProteinSimple, San Jose, CA, USA) was used. The samples were denatured by incubating them with 0.1× sample buffer (ProteinSimple). The samples were incubated for 1 h with the primary antibodies, including antibodies against GAPDH (Proteintech, Rosemont, IL, USA), TLR4, NF-κB, p-NF-κB, and IL-6 (Cell Signaling Technology, Beverly, MA, USA). Total protein expression was normalized to GAPDH.
2.12 Statistical analysis
All statistical analyses were performed by the t-test or one-way ANOVA using SPSS 18.0 (SPSS Inc., Chicago, IL, USA). The data were presented as least squares means ± SEM. Differences were considered to be statistically significant when P < 0.05.
3. Results
3.1 FT-IR spectrum analysis
As shown in Fig. 1A, the characteristic absorption peaks of EP are derived from the stretching vibration of the O–H peak at 3432 cm−1, and the weak bands at 2931 cm−1 indicated the C–H stretching vibrations.19 The absorption peaks at 1634 cm−1 and 1429 cm−1 correspond to the carbonyl C
O vibrations and C–O stretching vibrations, respectively.5 The band at 1049 cm−1 corresponds to the C–O stretching vibrations. The typical peaks at around 1261 cm−1 and 850 cm−1 are the sulphate base, corresponding to the S
O asymmetric stretching vibrations and C–O–S symmetrical stretching vibrations, respectively.20 The spectrum of EP–Zn (Fig. 1B) is similar to that of EP, which means that the carbon chain skeleton is essentially the same. The EP–Zn complex showed a broader O–H stretching vibration at 3431 cm−1 compared with EP, inferring tentatively that the hydroxyl group underwent a coordination reaction with zinc. The C–O stretching vibration absorption peak shifted towards the higher band, inferring EP complexes with zinc as C–O–Zn.
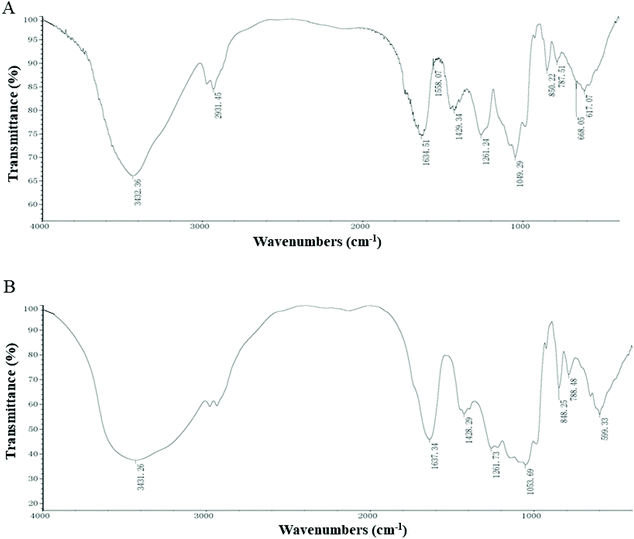 |
| Fig. 1 FT-IR spectra of (A) EP and (B) EP–Zn. | |
3.2 Effects of EP–Zn on the body weight, immune organ index, and serum cytokines
The results showed that EP–Zn treatment had no significant effect on the body weight and average daily food intake of mice (Fig. 2A and B). After 4 weeks of EP–Zn treatment, the spleen index of all mice was slightly lower relative to NC mice (Fig. 2D). In addition, the mice in the EP–Zn group exhibited significantly higher (P < 0.05) serum TNF-α, IL-1β, and IL-6 levels, and significantly lower (P < 0.05) IL-10 levels compared with the NC group (Fig. 2E–H). Notably, this effect decreased with an increase of the EP–Zn dose. Thus, the dosage of 350 mg kg−1 EP–Zn was selected for the subsequent experiments according to the immune response of mice in the intervention study.
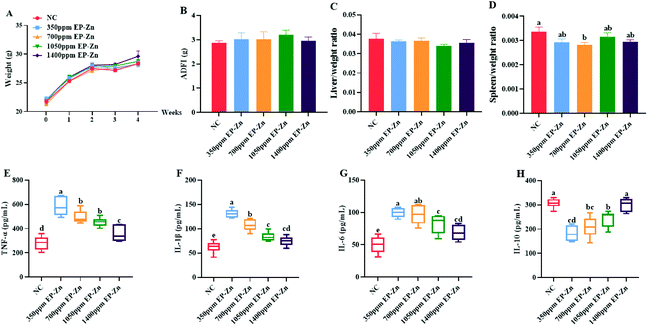 |
| Fig. 2 Effects of EP–Zn on the immune index. (A) Body weight change after 4 weeks of EP–Zn treatment (n = 12), (B) average daily food intake (n = 12), (C) liver index (n = 12), (D) spleen index (n = 12), (E) TNF-α in serum (n = 8), (F) IL-1β in serum (n = 8), (G) IL-6 in serum (n = 8), and (H) IL-10 in serum (n = 8). Values are presented as mean ± SEM. Differences were assessed by one-way ANOVA and different letters indicate significant differences. | |
3.3 Effects of EP–Zn on the gut microbiota
To assess the richness and diversity changes of the gut microbiota in response to EP–Zn, 16S rRNA high-throughput sequencing was performed on colon contents. A total of 369 OTUs were obtained as shown by Venn diagrams (Fig. 3A). The NC group had 47 special OTUs and the EP–Zn group had a larger number of unique OTUs (76). The diversity and richness of the microbial communities were measured by the Shannon, Simpson, Ace, and Chao indices (Fig. 3B). Consistent with the results of the OTUs, the diversity of the gut microbiota as exhibited by the Shannon and Simpson index in the EP–Zn group was significantly higher (P < 0.05) than that in the NC group. To evaluate the overall structural differences of the gut microbiota, principal component analysis (PCA) was performed. EP–Zn significantly changed the structure of the gut microbiota as presented by a drastic change in the microbial profile (Fig. 3C). Then, statistical differences in the microbial abundance from the taxonomic phylum to the genus were evaluated using LEfSe analysis (Fig. 3D), and the effect of species abundance on the differences between groups was estimated using LEfSe linear discriminant analysis (LDA) (Fig. 3E). Twenty-nine phylotypes from the phylum to genus were discovered as high-dimensional biomarkers. These microbes mainly belonged to the phylum Actinobacteria and Firmicutes. Remarkably, the genera Coriobacteriaceae _UCG-002 was a biomarker in the NC group. The genera Enteractinococcus, Oscillibacter, Rikenella, Atopostipes, and unclassified_f__Ruminococcaceae were predominant in the EP–Zn group. To further understand the composition of each bacterium, the bacterial compositions of the top 30 gut microbiota at the genus level were analyzed using Student's t-test. The results showed that Coriobacteriaceae_UCG-002, Brevibacterium, Brachybacterium, Dietzia, unclassified_f__Microbacteriaceae, and Parasutterella in the EP–Zn group were significantly attenuated (Fig. 3F, P < 0.05), while unclassified_f__Lachnospiraceae, Atopostipes, and unclassified_f__ Ruminococcaceae were significantly increased (Fig. 3F, P < 0.05).
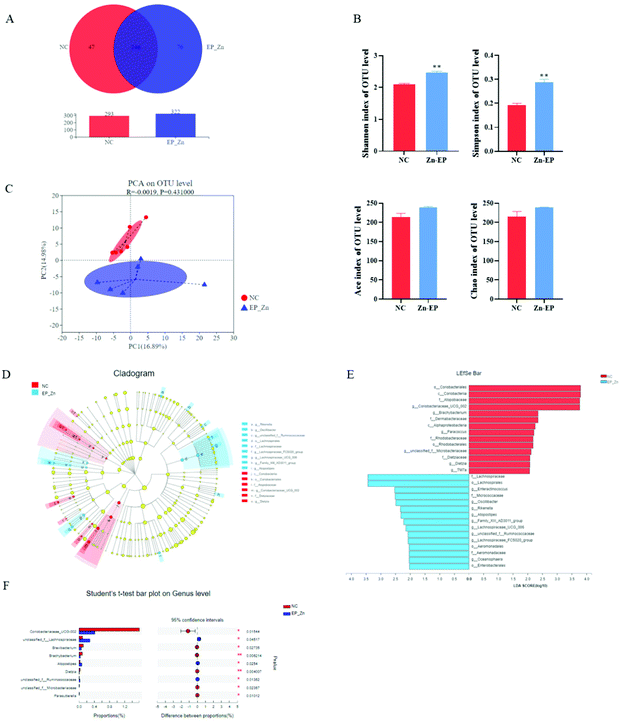 |
| Fig. 3 Effects of EP–Zn on the gut microbiota (n = 6). (A) Venn diagram of OTUs, (B) Shannon, Simpson, ACE and Chao indices (C) PCA of the OTU level, (D) cladogram generated from LEfSe analysis, (E) LDA score; LDA scores greater than 2 were considered as important differential abundances between groups, (F) Student's t-test bar plot at the genus level; values are presented as the mean ± SEM. Differences were assessed using Student's t-test and denoted as follows: *P < 0.05; **P < 0.01. | |
3.4 Effects of EP–Zn on the SCFAs and correlation analyses between SCFAs and the gut microbiota
To determine the effects of EP–Zn on SCFAs, the concentrations of acetic acid and propionic acid of colon digesta were measured, and it was found that EP–Zn supplementation reduced (P < 0.05) the fecal levels of acetic and propionic acids (Fig. 4A).
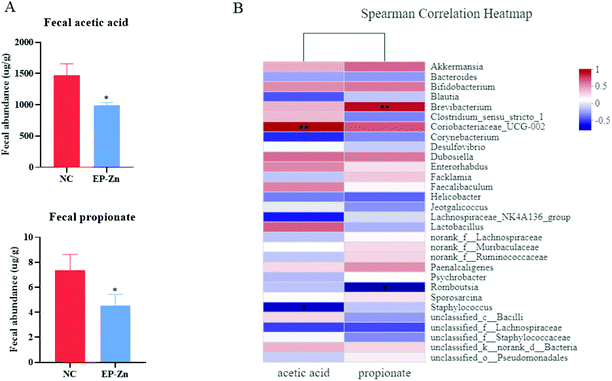 |
| Fig. 4 Effects of EP–Zn on SCFAs and the Spearman correlation heatmap between SCFAs and bacterial genera. (A) Fecal acetic acid and propionate (n = 8), (B) Spearman correlation heatmap between the bacterial genera and SCFAs (n = 6); values are presented as mean ± SEM. Differences were assessed using Student's t-test and denoted as follows: *P < 0.05; **P < 0.01. | |
To further investigate the relationship between the gut microbiota and SCFAs, the Spearman correlation analyses were carried out. According to the Spearman correlation heatmap analysis at the genus level, Coriobacteriaceae_UCG-002 showed a highly positive correlation with acetic acid and Brevibacterium was positively correlated with propionate (Fig. 4B, P < 0.01).
3.5 Effects of the EP–Zn supplement on intestinal inflammation and barrier damage in LPS-induced mice
To determine the effects of EP–Zn on intestinal inflammation and barrier damage, a mouse model induced by LPS was used. The results revealed that LPS treatment significantly decreased (P < 0.001) the body weight and increased (P < 0.001) the spleen weight after 24 hours (Fig. 5A and B). The colon length of the mice treated with LPS significantly shortened (P < 0.05) relative to the NC mice (Fig. 5C), while EP–Zn pre-treatment alleviated (P < 0.05) the decrease of the colon length in mice. Moreover, the LPS-treated mice showed significantly enhanced (P < 0.05) MPO and DAO activities in serum compared with the NC mice (Fig. 5D and E), while EP–Zn pre-treatment prevented (P < 0.01) these changes. Meanwhile, the shape of the ileal villi in the LPS-treated mice appeared to be partially lost and sloughed as observed in the HE staining results (Fig. 5F), in contrast, the ileal villi in the NC mice and EP–Zn pretreated mice did not exhibit these changes. The colon of the mice treated with LPS showed a massive reduction in goblet cells and colonic epithelial cells, a large inflammatory infiltrate of mononuclear cells in the mucosa and submucosa, and mucosal edema in the intestinal mucosa and muscularis (Fig. 5F). The intestinal pathological damage was significantly alleviated in the mice pretreated with EP–Zn.
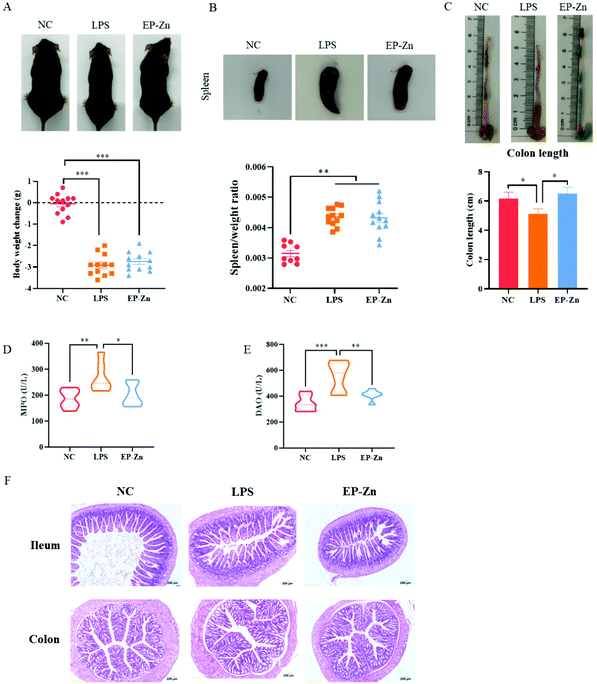 |
| Fig. 5 Effects of EP–Zn on intestinal inflammation and barrier damage induced by LPS. (A) Body weight changes before and after LPS injection (n = 12), (B) images of spleen and spleen/weight ratio (n = 9–12), (C) images and lengths of colon (n = 8), (D) serum MPO (n = 8), (E) serum DAO (n = 8), (F) H&E staining of the ileum and colon (×200; n = 8); values are presented as the mean ± SEM. Differences were assessed using one-way ANOVA and denoted as follows: *P < 0.05, **P < 0.01, and ***P < 0.001. | |
3.6 Effects of the EP–Zn supplement on the NF-κB pathway in LPS-induced mice
To clarify whether EP–Zn prevents LPS-induced intestinal inflammation via the TLR4/NF-κB signaling pathway, the related protein and mRNA expressions were measured. The result showed that the protein levels of TLR4, phosphorylated NF-κB and IL-16 were markedly enhanced (P < 0.05) in the LPS-treated mice, while EP–Zn pre-treatment prevented these changes (Fig. 6A). Moreover, the mRNA expressions of related genes (TLR4, NF-κB, IL-1β, IL-6, and IL-17) were significantly increased (P < 0.05) in the LPS mice, whereas these increments were prevented by EP–Zn pre-treatment (Fig. 6B).
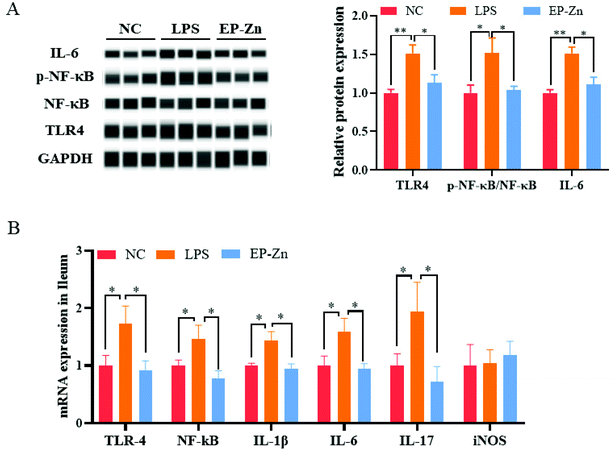 |
| Fig. 6 Effects of EP–Zn on the TLR4/NF-κB signalling pathway. (A) Relative mRNA expression in the ileum (n = 8), (B) relative protein expression in the ileum (n = 3); values are presented as the mean ± SEM. Differences were assessed using one-way ANOVA and denoted as follows: *P < 0.05, **P < 0.01, and ***P < 0.001. | |
4. Discussion
The immunomodulatory effects of sulfated polysaccharides and their complexes have been highlighted in recent years.11,21–23It has been reported that sulfated EP could stimulate the secretion of IL-2 and IFN-γ,8,22 and the polysaccharide–iron complex can restore serum cytokine levels including TNF-α, IFN-γ and IL-10.11 Our previous study showed that the EP–Zn complex promotes the development of the small intestine and enhances the antioxidant/inflammatory capacities of weaned piglets under physiological conditions.17
Based on the above information, herein, we first supplemented the mice with different levels of EP–Zn to identify the best dose. Then, the best dose was used for further study to explore the immunomodulatory activities and anti-inflammatory mechanisms of EP–Zn in the LPS-induced inflammation mice model. In the first study, the mice that received EP–Zn at different concentrations exhibited an increase in serum proinflammatory cytokines, including TNF-α, IL-1β, and IL-6, which is similar to the effect of EP.24 However, TNF-α, IL-1β, and IL-6 levels diminished and IL-10 increased with increasing doses. Thus, 350 mg kg−1 of EP–Zn was chosen as the effective dose for further study. IL-10, secreted by Th2 cells, can regulate the function of macrophages and inhibit the production of inflammatory cytokines such as TNF-α, IL-6, and IL-1β.25,26 We found that IL-10 showed an opposite trend to TNF-α, IL-6 and IL-1β after EP–Zn treatment. These results indicate that EP–Zn has immunomodulatory functions similar to EP, regulating the ability of Th1/Th2 cytokine secretion to enhance immunity. These results confirmed the immunomodulatory effects of the EP–Zn complex.
The gut microbiota is closely related to the immune reguation of the host and plays an important role in the digestion and absorption of polysaccharides via producing short-chain fatty acids.27–30 Accumulating evidence indicates that polysaccharides regulate the composition and metabolism of the gut microbiota.1,31–33After selecting 350 mg kg−1 EP–Zn as an effective dose based on the immune response results, we sequenced the 16S-rRNA from the colonic digesta of the 350 mg kg−1 EP–Zn group and control group mice to evaluate its effect on the gut microbiota dynamics. In the present study, we found that EP–Zn increased the diversity of the gut microbiota. As reported, dietary EP mainly promotes the growth of Akkermansia muciniphila, Bifidobacterium spp. and Lactobacillus spp. in female C57BL/6J mice.34 Our experimental analyses of the gut microbiota at the genus level were different from the results of the EP mice. The relative abundances of unclassified_f__Lachnospiraceae, Atopostipes, and unclassified_f__Ruminococcaceae genera were enhanced in the EP–Zn treated mice. Atopostipes are relatively new and may possess immunomodulatory activity.35Unclassified_f__Lachnospiraceae belonging to the Firmicutes phylum, which at low abundance levels, has been disclosed to increase the intestinal sensitivity to inflammation.36Unclassified_f__Ruminococcaceae levels were enhanced in high-fat diet-induced inflammatory mice.37 These significantly increased strains showed the immunomodulatory effect of EP–Zn. In addition, EP–Zn decreased the abundance of Coriobacteriaceae_UCG-002, Brevibacterium, Brachybacterium, Dietzia, unclassified_f__Microbacteriaceae, and Parasutterella genera. Coriobacteriaceae_UCG-002 and Brachybacterium, belonging to the Actinobacteria phylum,38 are potentially associated with inflammatory responses.39,40Brevibacterium is an uncommon but important agent which could cause opportunistic infections in immunocompetent individuals.41Parasutterella may be associated with chronic intestinal inflammation in irritable bowel syndrome (IBS) patients.42 These results provide supporting evidence that EP–Zn exhibited an immunomodulatory effect on gut microbiota dysbiosis.
Previous studies demonstrated that non-starch polysaccharides cannot be digested and absorbed directly by the small intestine until being fermented to short-chain fatty acids (SCFAs).1 As the main end product of the gut microbial fermentation of polysaccharides, SCFAs play crucial roles in modulating immune responses.31Aralia elata root barks20 and Crataegus pinnatifida43 polysaccharides were found to inhibit colitis via modulation of the gut microbiota and SCFA generation. In contrast, our results showed that EP–Zn treatment reduced the level of acetic and propionic acids in the feces. This might be because of the absorption of most of the SCFAs into the systemic circulation.44 We further performed the Spearman correlation analysis to identify the relationship between the detected SCFAs and microbiota genera. The results showed that Coriobacteriaceae_UCG-002 had a positive correlation with acetic acid, and Brevibacterium was positively correlated with propionate, suggesting that Coriobacteriaceae_UCG-002 and Brevibacterium may have a significant contribution to the generation of acetate and propionate. These results further confirmed that the changed intestinal microbiota and SCFAs observed in this study might play an important role in immunomodulation.
Plant polysaccharides have potential anti-inflammatory activity, inhibiting the LPS-induced secretion of inflammatory cytokines from macrophages.32 To further explore the role of EP–Zn in inflammation, an LPS-induced low-grade inflammatory mouse model was used in the present study. LPS stimulation can cause many pathological changes, including destroying the integrity of intestinal mucosa, increasing mucosal permeability, and intestinal inflammation.3,45 Decreased colon length and enlarged spleen were notable signs of colonic colitis.46 EP–Zn pre-treatment reversed the effects on colon length shortening induced by LPS, suggesting that pre-treatment with EP–Zn had a relieving effect on LPS-induced colitis. The gut barrier permeability and function were evaluated by the levels of MPO and DAO in serum. Both molecules are released into the bloodstream when the intestinal mucosa is destroyed.3,47 MPO is an enzyme associated with tissue damage under inflammatory conditions.48 DAO is located in the upper villi of the small intestinal mucosa, reflecting the structure and function of the intestine.49 In the current study, the serum MPO and DAO concentrations were increased in LPS-injected mice, whereas pre-treatment with EP–Zn prevented an LPS-induced increase in serum MPO and DAO levels, suggesting a protective role of EP–Zn in LPS-induced disruption of the barrier function and intestinal mucosal injury.
It has been reported that LPS can bind to TLR4 and activate the NF-κB pathway, causing an inflammatory response.50,51 Excessive release of inflammatory cytokines in LPS-treated mice may be responsible for chronic inflammation.3 Based on our previous study, we speculated that EP–Zn might play a role in reducing inflammation by inhibiting the TLR4/NF-κB signaling pathway. Cytokines such as interleukin (IL)-1β, IL-6, IL-10,52 and IL-1753 play an important role in the inflammatory process, which can reflect the generation of inflammation. We selected IL-1β and IL-6 genes to study the effects of EP–Zn on LPS-induced inflammation. A significant decrease in the expression of related proteins and mRNA expression in EP–Zn pre-treated mice was observed in the present study. The results indicated the ameliorative effect of EP–Zn on the LPS-induced inflammatory response via modulation of the TLR4/NF-κB signaling pathway.
5. Conclusion
In conclusion, the present study demonstrated that EP–Zn exerted its immunomodulatory and anti-inflammatory activities under physiological and inflammatory conditions, respectively. EP–Zn modulates the immune response, reduces SCFA levels and alters the composition of the gut microbiota under physiological conditions. Furthermore, pretreatment with EP–Zn prevented the inflammatory response and attenuated the intestinal barrier impairment by inhibiting the TLR4/NF-κB signaling pathway in LPS-treated mice. However, how the role of EP–Zn is different under physiological and inflammatory conditions needs further study. This work could provide theoretical support for the application of EP in the food industry and has great practical implications in the development of novel functional foods.
Author contributions
Yumei Zhang: conceptualization, methodology, investigation, formal analysis, data curation, and writing – original draft. Xinyi Duan: conceptualization and formal analysis. Teketay Wassie: writing – original draft. Hai-hua Wang: conceptualization and methodology. Tiejun Li: conceptualization and methodology. Chunyan Xie: supervision, and writing – review and editing. Xin Wu: writing – review and editing.
Conflicts of interest
The authors declare there is no conflict of interest.
Acknowledgements
This work was supported by grants from the National Natural Science Foundation of China (31902196), the Natural Science Foundation of Hunan Province (2019JJ50268), and the China Postdoctoral Science Foundation project (2019M662273).
References
- T. Wu, M. Shen, X. Guo, L. Huang, J. Yang, Q. Yu, Y. Chen and J. Xie, Cyclocarya paliurus polysaccharide alleviates liver inflammation in mice via beneficial regulation of gut microbiota and TLR4/MAPK signaling pathways, Int. J. Biol. Macromol., 2020, 160, 164–174 CrossRef CAS.
- C. Zhu, Y. Wu, Z. Jiang, C. Zheng, L. Wang, X. Yang, X. Ma, K. Gao and Y. Hu, Dietary soy isoflavone attenuated growth performance and intestinal barrier functions in weaned piglets challenged with lipopolysaccharide, Int. Immunopharmacol., 2015, 28, 288–294 CrossRef CAS.
- X. Zhou, Y. Zhang, L. He, D. Wan, G. Liu, X. Wu and Y. Yin, Serine prevents LPS-induced intestinal inflammation and barrier damage via p53-dependent glutathione synthesis and AMPK activation, J. Funct. Foods, 2017, 39, 225–232 CrossRef CAS.
- X. H. Gu, Y. Hao and X. L. Wang, Overexpression of heat shock protein 70 and its relationship to intestine under acute heat stress in broilers: 2. Intestinal oxidative stress, Poult. Sci., 2012, 91, 790–799 CrossRef CAS.
- L. Qiao, Y. Li, Y. Chi, Y. Ji, Y. Gao, H. Hwang, W. G. Aker and P. Wang, Rheological properties, gelling behavior and texture characteristics of polysaccharide from Enteromorpha prolifera, Carbohydr. Polym., 2016, 136, 1307–1314 CrossRef CAS PubMed.
- Y. Chi, Y. Li, G. Zhang, Y. Gao, H. Ye, J. Gao and P. Wang, Effect of extraction techniques on properties of polysaccharides from Enteromorpha prolifera and their applicability in iron chelation, Carbohydr. Polym., 2018, 181, 616–623 CrossRef CAS PubMed.
- Y. Yu, Y. Li, C. Du, H. Mou and P. Wang, Compositional and structural characteristics of sulfated polysaccharide from Enteromorpha prolifera, Carbohydr. Polym., 2017, 165, 221–228 CrossRef CAS PubMed.
- L. Huang, M. Shen, G. A. Morris and J. Xie, Sulfated polysaccharides: Immunomodulation and signaling mechanisms, Trends Food Sci. Technol., 2019, 92, 1–11 CrossRef CAS.
- Y. Yu, M. Shen, Z. Wang, Y. Wang, M. Xie and J. Xie, Sulfated polysaccharide from Cyclocarya paliurus enhances the immunomodulatory activity of macrophages, Carbohydr. Polym., 2017, 174, 669–676 CrossRef CAS PubMed.
- J. Cui, Y. Li, P. Yu, Q. Zhan, J. Wang, Y. Chi and P. Wang, A novel low molecular weight Enteromorpha polysaccharide-iron(III) complex and its effect on rats with iron deficiency anemia (IDA), Int. J. Biol. Macromol., 2018, 108, 412–418 CrossRef CAS PubMed.
- X. Gao, H. Qu, Z. Gao, D. Zeng, J. Wang, D. Baranenko, Y. Li and W. Lu, Protective effects of Ulva pertusa polysaccharide and polysaccharide iron(III) complex on cyclophosphamide induced immunosuppression in mice, Int. J. Biol. Macromol., 2019, 133, 911–919 CrossRef CAS PubMed.
- Z. Zhou, S. K. Pan and S. J. Wu, Modulation of the growth performance, body composition and nonspecific immunity of crucian carp Carassius auratus upon Enteromorpha prolifera polysaccharide, Int. J. Biol. Macromol., 2020, 147, 29–33 CrossRef CAS PubMed.
- T. Wassie, K. M. Niu, C. Xie, H. Wang and X. Wu, Extraction Techniques, Biological Activities and Health Benefits of Marine Algae Enteromorpha prolifera polysaccharide, Front. Nutr., 2021 DOI:10.3389/fnut.2021.747928.
- S. Hojyo and T. Fukada, Roles of Zinc Signaling in the Immune System, J. Immunol. Res., 2016, 2016, 6762343 Search PubMed.
- M. Zhang, H. Zhao, Y. Shen, Y. Wang, Z. Zhao and Y. Zhang, Preparation, characterization and antioxidant activity evaluation in vitro of Fritillaria ussuriensis polysaccharide-zinc complex, Int. J. Biol. Macromol., 2020, 146, 462–474 CrossRef CAS PubMed.
- Y. Zhang, M. Z. H. Khan, T. Yuan, Y. Zhang, X. Liu, Z. Du and Y. Zhao, Preparation and characterization of D. opposita Thunb polysaccharide-zinc inclusion complex and evaluation of anti-diabetic activities, Int. J. Biol. Macromol., 2019, 121, 1029–1036 CrossRef CAS PubMed.
- C. Xie, Y. Zhang, K. Niu, X. Liang, H. Wang, J. Shan and X. Wu, Enteromorpha polysaccharide-zinc replacing prophylactic antibiotics contributes to improving gut health of weaned piglets, Anim. Nutr., 2021, 7, 641–649 CrossRef PubMed.
- J. Yin, Y. Li, H. Han, S. Chen, J. Gao, G. Liu, X. Wu, J. Deng, Q. Yu, X. Huang, R. Fang, T. Li, R. J. Reiter, D. Zhang, C. Zhu, G. Zhu, W. Ren and Y. Yin, Melatonin reprogramming of gut microbiota improves lipid dysmetabolism in high-fat diet-fed mice, J. Pineal Res., 2018, 65, e12524 CrossRef PubMed.
- R. Huang, M. Shen, Y. Yu, X. Liu and J. Xie, Physicochemical characterization and immunomodulatory activity of sulfated Chinese yam polysaccharide, Int. J. Biol. Macromol., 2020, 165, 635–644 CrossRef CAS PubMed.
- Y. G. Xia, T. L. Wang, S. M. Yu, J. Liang and H. X. Kuang, Structural characteristics and hepatoprotective potential of Aralia elata root bark polysaccharides and their effects on SCFAs produced by intestinal flora metabolism, Carbohydr. Polym., 2019, 207, 256–265 CrossRef CAS PubMed.
- H. S. Kim, Y. J. Kim, K. L. Hong, H. S. Ryu, S. K. Ji, J. Y. Mi, J. S. Kang, T. H. Jin, Y. Kim and S. B. Han, Activation of macrophages by polysaccharide isolated from Paecilomyces cicadae through toll-like receptor 4, Food Chem. Toxicol., 2012, 50, 3190–3197 CrossRef CAS PubMed.
- J. K. Kim, M. L. Cho, S. Karnjanapratum, I. S. Shin and S. G. You, In vitro and in vivo immunomodulatory activity of sulfated polysaccharides from Enteromorpha prolifera, Int. J. Biol. Macromol., 2011, 49, 1051–1058 CrossRef CAS PubMed.
- Y. Geng, L. Xing, M. Sun and F. Su, Immunomodulatory effects of sulfated polysaccharides of pine pollen on mouse macrophages, Int. J. Biol. Macromol., 2016, 91, 846–855 CrossRef CAS PubMed.
- R. Zhong, X. Wan, D. Wang, C. Zhao, D. Liu, L. Gao, M. Wang, C. Wu, S. M. Nabavid, M. Daglia, E. Capanoglu, J. Xiao and H. Cao, Polysaccharides from Marine Enteromorpha: Structure and function, Trends Food Sci. Technol., 2020, 99, 11–20 CrossRef CAS.
- J. L. Schultze, A. Schmieder and S. Goerdt, Macrophage activation in human diseases, Semin. Immunol., 2015, 27, 249–256 CrossRef CAS PubMed.
- M. P. Motwani and D. W. Gilroy, Macrophage development and polarization in chronic inflammation, Semin. Immunol., 2015, 27, 257–266 CrossRef PubMed.
- Q. Fang, J. Hu, Q. Nie and S. Nie, Effects of polysaccharides on glycometabolism based on gut microbiota alteration, Trends Food Sci. Technol., 2019, 92, 65–70 CrossRef CAS.
- D. P. Venegas, M. Fuente, G. Landskron, M. J. Gonzalez, R. Quera, G. Dijkstra, H. Harmsen, K. N. Faber and M. A. Hermoso, Short Chain Fatty Acids (SCFAs)-Mediated Gut Epithelial and Immune Regulation and Its Relevance for Inflammatory Bowel Diseases, Front. Immunol., 2019, 10, 277 CrossRef CAS PubMed.
- D. Zheng, T. Liwinski and E. Elinav, Interaction between microbiota and immunity in health and disease, Cell Res., 2020, 30, 492–506 CrossRef PubMed.
- J. Huang, J. Huang, Y. Li, Y. Wang, F. Wang, X. Qiu, X. Liu and H. Li, Sodium Alginate Modulates Immunity, Intestinal Mucosal Barrier Function, and Gut Microbiota in Cyclophosphamide-Induced Immunosuppressed BALB/c Mice, J. Agric. Food Chem., 2021, 69, 7064–7073 CrossRef CAS.
- M. Ying, Q. Yu, B. Zheng, H. Wang, J. Wang, S. Chen, S. Nie and M. Xie, Cultured Cordyceps sinensis polysaccharides modulate intestinal mucosal immunity and gut microbiota in cyclophosphamide-treated mice, Carbohydr. Polym., 2020, 235, 115957 CrossRef CAS PubMed.
- X. Zhang, N. Zhang, J. Kan, R. Sun, S. Tang, Z. Wang, M. Chen, J. Liu and C. Jin, Anti-inflammatory activity of alkali-soluble polysaccharides from Arctium lappa L. and its effect on gut microbiota of mice with inflammation, Int. J. Biol. Macromol., 2020, 154, 773–787 CrossRef CAS PubMed.
- M. Jin, Y. Zhu, D. Shao, K. Zhao, C. Xu, Q. Li, H. Yang, Q. Huang and J. Shi, Effects of polysaccharide from mycelia of Ganoderma lucidum on intestinal barrier functions of rats, Int. J. Biol. Macromol., 2017, 94, 1–9 CrossRef CAS.
- Q. Shang, Y. Wang, L. Pan, Q. Niu, C. Li, H. Jiang, C. Cai, J. Hao, G. Li and G. Yu, Dietary Polysaccharide from Enteromorpha Clathrata Modulates Gut Microbiota and Promotes the Growth of Akkermansia muciniphila, Bifidobacterium spp. and Lactobacillus spp, Mar. Drugs, 2018, 16 DOI:10.3390/md16050167.
- S. Stanisavljevic, J. Lukic, M. Momcilovic, M. Miljkovic, B. Jevtic, M. Kojic, N. Golic, M. Stojkovic and D. Miljkovic, Gut-associated lymphoid tissue, gut microbes and susceptibility to experimental autoimmune encephalomyelitis, Benefic. Microbes, 2016, 7, 363–373 CrossRef CAS PubMed.
- H. Chen, F. Zhang, R. Li, Y. Liu, X. Wang, X. Zhang, C. Xu, Y. Li, Y. Guo and Q. Yao, Berberine regulates fecal metabolites to ameliorate 5-fluorouracil induced intestinal mucositis through modulating gut microbiota, Biomed. Pharmacother., 2020, 124, 109829 CrossRef CAS PubMed.
- D. Hou, Q. Zhao, L. Yousaf, Y. Xue and Q. Shen, Beneficial effects of mung bean seed coat on the prevention of high-fat diet-induced obesity and the modulation of gut microbiota in mice, Eur. J. Nutr., 2020, 60, 2029–2045 CrossRef.
- X. Gao, L. Du, E. Randell, H. Zhang, K. Li and D. Li, Effect of different phosphatidylcholines on high fat diet-induced insulin resistance in mice, Food Funct., 2021, 12, 1516–1528 RSC.
- Z. W. Yi, Y. J. Xia, X. F. Liu, G. Q. Wang, Z. Q. Xiong and L. Z. Ai, Antrodin A from mycelium of Antrodia camphorata alleviates acute alcoholic liver injury and modulates intestinal flora dysbiosis in mice, J. Ethnopharmacol., 2020, 254 DOI:10.1016/j.jep.2020.112681.
- S. Zhao, X. Peng, Q. Y. Zhou, Y. Y. Huang, X. Rao, J. L. Tu, H. Y. Xiao and D. M. Liu, Bacillus coagulans 13002 and fructo-oligosaccharides improve the immunity of mice with immunosuppression induced by cyclophosphamide through modulating intestinal-derived and fecal microbiota, Food Res. Int., 2021, 140, 109793 CrossRef CAS.
- N. Asai, H. Suematsu, A. Yamada, H. Watanabe, N. Nishiyama, D. Sakanashi, H. Kato, A. Shiota, M. Hagihara, Y. Koizumi, Y. Yamagishi and H. Mikamo, Brevibacterium paucivorans bacteremia: Case report and review of the literature, BMC Infect. Dis., 2019, 19 DOI:10.1186/s12879-019-3962-y.
- Y. J. Chen, H. Wu, S. D. Wu, N. Lu, Y. T. Wang, H. N. Liu, L. Dong, T. T. Liu and X. Z. Shen, Parasutterella, in association with irritable bowel syndrome and intestinal chronic inflammation, J. Gastroenterol. Hepatol., 2018, 33, 1844–1852 CrossRef CAS.
- C. Guo, Y. Wang, S. Zhang, X. Zhang, Z. Du, M. Li and K. Ding, Crataegus pinnatifida polysaccharide alleviates colitis via modulation of gut microbiota and SCFAs metabolism, Int. J. Biol. Macromol., 2021, 181, 357–368 CrossRef CAS PubMed.
- R. Han, Y. Ma, J. Xiao, L. You, S. Pedisic and L. Liao, The possible mechanism of the protective effect of a sulfated polysaccharide from Gracilaria Lemaneiformis against colitis induced by dextran sulfate sodium in mice, Food Chem. Toxicol., 2021, 149, 112001 CrossRef CAS PubMed.
- H. Liu, K. M. Feye, Y. T. Nguyen, A. Rakhshandeh, C. L. Loving, J. C. M. Dekkers, N. K. Gabler and C. K. Tuggle, Acute systemic inflammatory response to lipopolysaccharide stimulation in pigs divergently selected for residual feed intake, BMC Genomics, 2019, 20, 728 CrossRef.
- Z. Xu, W. Liu, Y. Zhang, D. Zhang, B. Qiu, X. Wang, J. Liu and L. Liu, Therapeutic and Prebiotic Effects of Five Different Native Starches on Dextran Sulfate Sodium-Induced Mice Model of Colonic Colitis, Mol. Nutr. Food Res., 2021, e2000922, DOI:10.1002/mnfr.202000922.
- L. Yang, G. Liu, K. Lian, Y. Qiao, B. Zhang, X. Zhu, Y. Luo, Y. Shang and X. L. Gu, Dietary leonurine hydrochloride supplementation attenuates lipopolysaccharide challenge-induced intestinal inflammation and barrier dysfunction by inhibiting the NF-kappaB/MAPK signaling pathway in broilers, J. Anim. Sci., 2019, 97, 1679–1692 CrossRef.
- K. Gil-Cardoso, R. Comitato, I. Gines, A. Ardevol, M. Pinent, F. Virgili, X. Terra and M. Blay, Protective Effect of Proanthocyanidins in a Rat Model of Mild Intestinal Inflammation and Impaired Intestinal Permeability Induced by LPS, Mol. Nutr. Food Res., 2019, 63, e1800720 CrossRef PubMed.
- X. Y. Zhang, J. Chen, K. Yi, L. Peng, J. Xie, X. Gou, T. Peng and L. Tang, Phlorizin ameliorates obesity-associated endotoxemia and insulin resistance in high-fat diet-fed mice by targeting the gut microbiota and intestinal barrier integrity, Gut Microbes, 2020, 12, 1–18 CrossRef PubMed.
- J. F. Burgueno and M. T. Abreu, Epithelial Toll-like receptors and their role in gut homeostasis and disease, Nat. Rev. Gastroenterol. Hepatol., 2020, 17, 263–278 CrossRef CAS PubMed.
- A. E. Price, K. Shamardani, K. A. Lugo, J. Deguine, A. W. Roberts, B. L. Lee and G. M. Barton, A Map of Toll-like Receptor Expression in the Intestinal Epithelium Reveals Distinct Spatial, Cell Type-Specific, and Temporal Patterns, Immunity, 2018, 49, 560–575 CrossRef CAS PubMed.
- P. A. Hwang, S. Y. Chien, Y. L. Chan, M. K. Lu, C. H. Wu, Z. L. Kong and C. J. Wu, Inhibition of Lipopolysaccharide (LPS)-induced inflammatory responses by Sargassum hemiphyllum sulfated polysaccharide extract in RAW 264.7 macrophage cells, J. Agric. Food Chem., 2011, 59, 2062–2068 CrossRef CAS.
- A. Beringer, M. Noack and P. Miossec, IL-17 in Chronic Inflammation: From Discovery to Targeting, Trends Mol. Med., 2016, 22, 230–241 CrossRef CAS PubMed.
|
This journal is © The Royal Society of Chemistry 2022 |
Click here to see how this site uses Cookies. View our privacy policy here.