A fast, single column extraction chromatography method for the isolation of neodymium from iron-rich materials prior to radiogenic isotope ratio measurements†
Received
29th July 2021
, Accepted 9th December 2021
First published on 9th December 2021
Abstract
A novel separation method is described for the separation of Nd from Fe-rich, silicate samples in view of isotopic analyses. The procedure is based on the synergistic enhancement of the extraction of the REE from HCl media by the diglycolamide ligand in the presence of large amounts of Fe(III). Following sample decomposition with a mixture of HF and HCl, the resulting, ca. 4 M HCl solution is immediately loaded on a column of DGA resin, without evaporation of HF and volatilization of silicon. Both Fe(III) and the REE are extracted by the resin while most other elements pass through the column. Then, iron is stripped from the column with 3 M HNO3. Finally, the LREE are eluted sequentially by means of HCl of decreasing strength, thereby providing a Nd fraction suitable for isotope ratio measurements by MC-ICP-MS. Because it does not require evaporation steps, this method offers a robust, straightforward alternative to more complicated schemes when samples unusually rich in iron are to be analysed. The potential of this protocol is demonstrated by several replicate analyses of two Fe-rich international SRMs, namely the banded iron IF-G (56 wt% Fe2O3) and the bauxite BX-N (23% Fe203).
Introduction
The overall composition of primary magmatic silicate rocks constitutive of the continental and oceanic crusts and of the underlying upper mantle involves some ten, so-called major elements (Si, Al, Na, K, Ca, Mg, Fe, Ti, Mn), whose relative proportions span a relatively narrow range reflecting the rather limited fractionation of these elements during igneous processes.
In contrast with these lithologies formed at high temperatures, some sedimentary rocks offer much more extreme compositions, characterized by the overwhelming proportion of a few elements only.
This is the case of two peculiar classes of sedimentary rocks that are highly enriched in iron, namely banded iron ores, formed via selective chemical precipitation of silica and iron hydroxides in the oceans, on the one hand, and laterites, left as residues of severe continental alteration processes, on the other.
(i) Banded iron ores, or banded iron formations (BIF), are chemical deposits generally formed in relatively deep-water settings, consisting of alternating layers of chert (amorphous silica) and iron oxides (magnetite and hematite).1 These peculiar sediments, mostly restricted to the end of Archaean times (2800–2400 Ma), form the main deposits of iron worldwide. Besides their economic interest, they receive much academic attention because they are interpreted to reflect the precipitation of Fe(III), insoluble at near neutral pH value in the presence of even traces of dissolved oxygen, from Fe(II) dissolved in the ocean waters2 (and refs therein). Accordingly, they provide an important piece of information on the redox state of the ocean and atmosphere, as suggested by the observation that the peak of their deposition shortly preceded the first rise in atmospheric oxygen, the so-called « Great Oxidation Event », ca. 2.4 Ga ago.3
(ii) Laterites are brick-like rocks, passively enriched in those major elements forming insoluble hydroxides, Fe and Al, through severe leaching of all accompanying, soluble constituents, under hot and wet tropical climates (e.g.,4 and references therein). Laterites can be the products of advanced chemical weathering of a wide range of parent rocks, and these residual lithologies occupy about one third of the surface of the continents.5 Their Al2O3-rich end-member, bauxites, are of great economic importance as the major source of aluminum. Likewise, some 70% of land-based nickel resources are contained in laterites developed from ultramafic source rocks.6
Just as Fe(III) and Al, the trivalent lanthanide form insoluble hydroxides in natural waters under most circumstances, and they can be used to study the petrogenetic processes involved in the formation of iron-rich sediments. Among them, Nd is of special importance because its radiogenic isotope composition can be used as a proxy for tracing the provenance of Fe and, for example in the case of BIFs, discriminating models advocating a continental source against those favouring an origin at hydrothermal vents of mid-ocean ridges. It is thus interesting to develop straightforward separation schemes enabling the analyst to recover the REE from such chemically distinct matrices. In this scope, it was decided to develop a method exploiting a unique synergistic effect discovered by Horwitz and coworkers,7 namely, the strong enhancement in the uptake of trivalent REE from chloride media by a diglycolamide-based extraction chromatography resin in the presence of high levels of ferric ion.
The aim of this paper is to describe a new protocol based on this effect, and document its potential for the Nd isotope analyses of this peculiar class of geological samples.
Experimental
Chemicals, labware, extraction chromatographic materials, and instrumentation
All chemical handling was carried out under class 10 vertical laminar flow hoods in a laboratory supplied with an overpressure of filtered air, at a temperature of 20 °C.
Water was deionized with conventional ion-exchange resins and further purified to a resistivity of 18.20 MOhm cm with an Aqua System Distribution (Bondoufle, France) device. Reagent grade hydrofluoric, hydrochloric, and nitric acids (Fluka, Seelze, Germany) were purified by subboiling distillation in PFA DST-100 systems (Savillex, Eden Prairie, MN, USA). Ammonium thiocyanate (Honeywell Fluka) was used as a colorimetric agent to monitor the behaviour of iron during the separation and check that it was reduced to negligible level before starting the elution of the lanthanides.
Screw cap PFA vessels with a rounded bottom (15 ml capacity, Savillex, Minnetonka, USA) were used to decompose the samples on a hot plate.
The DGA resin was obtained from Triskem International (Bruz, France). A quadrupole-base ICP-MS (Model 7500, Agilent Technologies, Massy, France) was used in the semi-quantitative mode to set up the separation protocol, and for measuring the concentrations of the Rare Earth Elements in the studied samples, while a Neptune Plus multi-collector ICP-MS (Thermo Instruments, Bremen, Germany) was used for the measurements of Nd isotope ratios.
Chromatographic columns
Aliquots of 500 mg of DGA resin were slurried in 0.05 M HNO3 and poured into ca. 7.5 mm i.d. polystyrene columns fitted with a 15–45 μm polyethylene filter at the bottom (5-inch chromatography columns, Caplugs Evergreen, Buffalo, N. Y., U.S.A), giving a ca. 31 mm high resin bed. An additional frit was placed on top of the resin to ensure a flat upper surface of the resin bed.
Chemical procedure
Sample digestion (for 100–200 mg).
About 100 mg of iron-rich, BIF or lateritic sample is weighted on a piece of weighting paper, and gently poured into a 15 ml screw cap PFA vessel containing 4 ml of 6 M HCl. The mixture is thoroughly mixed 15 minutes in an ultrasonic bath. Then, 2 ml of 48% HF is added, and the closed vessel placed on a hot plate at ∼110 °C overnight. The next day, the still warm sample solution is transferred to a centrifuge tube with an additional fraction of 1 ml 4 M HCl to rinse the PFA dissolution vessel, and centrifuged 5 minutes at 4000 rpm. Because such samples do not contain appreciable amount of Ca and Mg, a clear HF–HCl solution should be obtained at this stage, without precipitate of sparingly soluble fluorides of alkali earth elements. In case a solid residue of refractory minerals (e.g. zircon) is observed, this should be isolated, transferred to a steel jacketed, Teflon high pressure vessel for being decomposed with HF at elevated temperature, and the resulting liquid added to the main sample solution.
Separation chemistry.
The column of DGA resin is first preconditioned with one column reservoir (ca. 6 ml) of 4 M HCl. Then, the centrifuged HF–HCl sample solution, roughly 4 M in HCl, is loaded onto the resin, which takes a brown colour and is rather soon saturated in Fe(III), as indicated by the bright yellow colour of the effluent. The REE are extracted at that stage. Following loading, the column is rinsed with 2 × 1 ml of 4 M HCl to get rid of the interstitial sample solution. Then, the eluant is switched to nitric acid in order to strip iron while leaving the REE strongly held by the column. The decrease of Fe(III) to a negligible level is verified using an ammonium thiocyanate colorimetric test carried out on a few μl of each successive fraction, until the dark red colour gives way to a very pale pink. Four fractions of 1 ml of 3 M HNO3 are usually sufficient to get rid of iron, but a twice larger volume can be passed through the column without causing any breakthrough of the light lanthanides. Then, the eluant is switched back to the hydrochloric medium to start the sequential elution of the REE. After 1 ml of 3 M HCl to effect the acid transition, a pre-Nd fraction containing essentially all La and Ce, and a large part of Pr is eluted with 5 ml of 2 M HCl. Then, a Nd cut suitable for MC-ICP-MS isotope ratio analyses is obtained with 4 ml of 1.25 M HCl. If required, Sm can be recovered in a further fraction of 5 ml of 0.70 M HCl. Next, the heavier lanthanides (from Eu to Lu) are stripped as a group with one column reservoir (∼6 ml) of very dilute, 0.05 M HCl. Finally, the column is washed with one reservoir of 0.1 M HCl-0.29 M HF followed by one further reservoir of 0.05 M HCl. This protocol is outlined in Table 1 and displayed in Fig. 1. The total duration of the separation process is about 5 hours.
Table 1 Outline of the separation scheme enabling the isolation of purified Nd from iron-rich samples by single column extraction chromatography
|
Reagents |
Volume (mL) |
DGA (500 mg) |
Pre-conditioning |
4 M HCl |
6 |
Sample loading |
4 M HCl |
2 × 3.5 |
Column rinse |
4 M HCl |
2 × 1 |
Iron stripping |
3 M HNO3 |
4 × 1 |
Elution of LREE |
3 M HCl |
1 |
2 M HCl |
5 × 1 |
Elution of Nd |
1.25 M HCl |
4 × 1 |
(Elution of Sm, optional) |
0.70 M HCl |
5 × 1 |
Elution of HREE |
0.05 M HCl |
5 × 1 |
Cleaning before storage |
0.1 M HCl – 0.29 M HF |
6 |
0.05 M HCl |
6 |
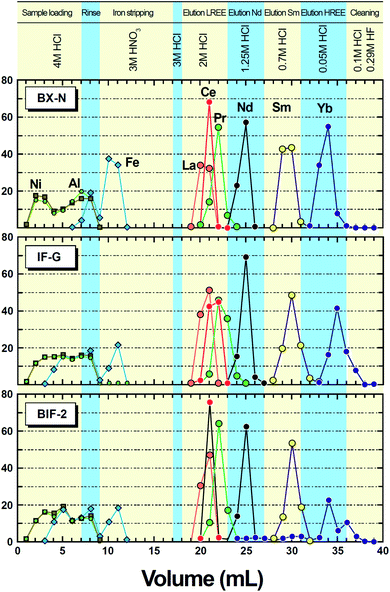 |
| Fig. 1 Elution curves on DGA single column performed for three natural samples. The first two are international standard reference materials of bauxite (BX-N) and Banded Iron Formation (IF-G). The third is a BIF sample from Eastern Indian Craton (BIF-2). Despite the chemically distinct matrices between these samples, the recovery of REE is reproducible within the given step. | |
Mass spectrometry.
After evaporation to dryness to get rid of HCl, the Nd fraction was dissolved in an appropriate volume of 0.05 M HNO3 prior to isotope ratios analysis by MC-ICP-MS. The operating parameters of the Neptune Plus instrument are detailed in Table 2.
Table 2 Instrument settings and data acquisition parameters for isotope ratio measurements of Nd by MC-ICP-MS (Neptune Plus, Thermo Fisher Scientific)
Analysis |
Nd isotopes |
Laboratory |
Magmas et Volcans |
MC-ICP-MS model |
Thermo Neptune Plus |
Plasma condition |
Wet (cyclonic spray chamber) |
RF power |
1200 W |
Resistors |
1011 Ohms |
Resolution |
Low (M/ΔM ∼ 400) |
Cool gas flow (Ar) |
15 l min−1 |
Auxiliary gas (Ar) |
0.7–0.8 l min−1 (optimised daily) |
Sample gas (Ar) |
0.9–1.1 l min−1 (optimised daily) |
Sample uptake |
100 μL min−1 |
Sample cone |
Standard |
Skimmer cone |
H |
Scanning mode |
Static multi-collection |
Integration time |
8.4 s |
Number of cycles |
60 |
Number of blocks |
1 |
Cup configuration |
L2 |
L1 |
C |
H1 |
H2 |
H3 |
H4 |
Mass collected |
143Nd |
144Nd |
145Nd |
146Nd |
147Sm |
148Nd |
150Nd |
Results
Two international SRMs, representative of the iron-rich matrices for which the separation procedure was developed, have been used to demonstrate its applicability, namely, IF-G, an Archaean (ca. 3.8 Ga) banded iron from Isua (Greenland), and BX-N, a Cretaceous (ca. 100 Ma) bauxite (Al-rich laterite) from Brignoles (France). Both geostandards were prepared by, and are available from SARM-CRPG (Nancy, France). Their recommended major element composition and Nd concentration are given in Table 3, along with abundances measured in this work. Interestingly, these two SRMs have highly contrasting REE abundances, with Nd contents differing by two orders of magnitude, viz. 1.7 μg g−1 in the chemical precipitate IF-G against 163 μg g−1 in the Al-rich laterite (leaching residue) BX-N. This contrast provides a good means to assess the potential of our separation method on a wide range of Nd/matrix ratios.
Table 3 Major and some REE trace element abundances in the standard reference materials investigated during this study (IF-G and BX-N) by ICP-AES and ICP-QMS, respectively
(Wt%) |
IF-G (iron formation) |
BX-N (bauxite) |
This study |
Number |
Ref. 8 |
Number |
This study |
Number |
Ref. 9 |
Number |
SiO2 |
40.15 ± 0.05 |
3 |
41.20 ± 0.67 |
81 |
7.11 ± 0.06 |
3 |
7.40 ± 0.46 |
37 |
Al2O3 |
0.34 ± 0.03 |
3 |
0.15 ± 0.09 |
76 |
53.38 ± 0.02 |
3 |
54.21 ± 1.16 |
34 |
Fe2O3T |
54.98 ± 0.17 |
3 |
55.85 ± 0.99 |
79 |
23.22 ± 0.08 |
3 |
23.2 ± 1.0 |
11 |
MgO |
2.23 ± 0.02 |
3 |
1.89 ± 0.16 |
84 |
0.28 ± 0.01 |
3 |
0.11 ± 0.08 |
29 |
CaO |
1.47 ± 0.03 |
3 |
1.55 ± 0.15 |
83 |
0.04 ± 0.02 |
3 |
0.17 ± 0.14 |
34 |
![[thin space (1/6-em)]](https://www.rsc.org/images/entities/char_2009.gif) |
|
(ppm) |
|
|
|
|
|
|
|
|
La |
2.66 ± 0.86 |
5 |
2.8 ± 0.6 |
24 |
353 ± 2 |
5 |
355 ± 76 |
12 |
Ce |
3.9 ± 1.1 |
5 |
4.0 ± 0.8 |
16 |
544 ± 12 |
5 |
520 ± 43 |
13 |
Nd |
1.7 ± 0.2 |
5 |
1.8 ± 0.2 |
15 |
163 ± 2 |
5 |
163 ± 31 |
7 |
Sm |
0.38 ± 0.01 |
5 |
0.4 ± 0.1 |
19 |
22.1 ± 0.2 |
5 |
22.0 ± 3.4 |
8 |
Yb |
0.58 ± 0.01 |
5 |
0.6 ± 0.3 |
22 |
11.0 ± 0.1 |
5 |
11.6 ± 2.1 |
8 |
143Nd/144Nd and 145Nd/144Nd isotope ratios
The results of multiple replicate analyses of Nd separated from the two Fe-rich SRMs are listed in Table S1,† together with measurements of the Nd isotope standard JNdi-1.14 The raw 143Nd/144Nd and 145Nd/144Nd ratios were corrected for the isobaric interference of 144Sm on 144Nd and also for instrumental mass bias by normalization to 146Nd/144Nd = 0.7219 by using an exponential function. It can be seen that the within-run (or internal) precisions of the 143Nd/144Nd ratios measured for the two rock samples (6 × 10−6 < 2SE < 10−5 for IF-G; 4 × 10−6 < 2SE < 7 × 10−6 for BX-N) do not differ significantly from those achieved for the chemically pure JNdi-1 standard (5 × 10−6< 2SE < 10−5), thereby demonstrating the efficiency of our single column separation procedure. Likewise, the uncertainty of the grand mean of the replicate analyses is only marginally larger than those of individual measurements, and not much different from that obtained on the pure Nd standard. The efficiency of the separation protocol and the robustness of the correction for the effect of the 144Sm interference were further checked by preparing several aliquots of the JNdi-1 isotopic standard spiked with variable amounts of Sm in order to encompass the range of Sm/Nd ratio found in natural samples. Half of these aliquots were processed through the DGA column in order to separate Nd from Sm prior to MC-ICP-MS measurements, while the rest of the Sm–Nd mixtures were analysed without further treatment. The 143Nd/144Nd were compared with repeat analyses of the Sm-free JNdi-1 solution as reference. The results listed in Table S2† and depicted in Fig. S1† show that, whatever the Sm/Nd ratios of the mixtures, the Sm abundance in the Nd separate is reduced by at least a factor of 103. Also, it can be seen that, within analytical precision, the 143Nd/144Nd ratios measured on the separated mixtures (0.512089, 2SD = 5 × 10−6, N = 5) do not differ from those measured on the pure JNdi-1 solution (0.512087, 2SD = 7 × 10−6, N = 6) thereby demonstrating the overall adequacy of the interference correction procedure. Finally, the results obtained on mixed Sm–Nd solutions analysed without any mutual separation show that accurate 143Nd/144Nd data still can be obtained (0.512091, 2SD = 9 × 10−6, N = 4). This further demonstrates that the interference correction of 144Sm during MC-ICP-MS analyses is extremely robust, a feature already well established, both by in situ laser ablation measurements of accessory minerals (e.g.,15,16) and by analyses of bulk LREE fractions separated from matrix elements (e.g.,17). The accuracy of our data can be assessed by the good agreement of the values of 145Nd/144Nd (constant) measured on the two SRMs and JNdi-1 and, for the radiogenic 143Nd/144Nd ratios, by a comparison with published values on IFG (Fig. 2). As far as we know, there is no Nd isotope data available for BX-N. Overall, these results highlight the good external reproducibility and accuracy of the method, thereby demonstrating its capacity to provide, in a very straightforward way, Nd fractions suitable for MC-ICP-MS isotope ratios determinations.
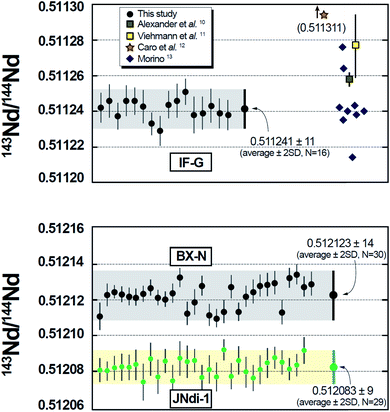 |
| Fig. 2 Nd Isotope composition for each individual digestion of the international reference material IF-G and BX-N processed through the protocol described in this study. Single measurements are plotted with their standard errors (±2S.E) while the average values are plotted with their external reproducibility (±2S.D). Literature data for IF-G standard are also reported.10–13 | |
Chemical yields
Due to the small difference of their distribution ratios, the short column used in this work does not allow to resolve the elution peaks of Pr and Nd, thus requiring making a trade-off between the chemical yield of Nd and its purity with regard to this element. However, the Fig. 1 show that about 80–90% of Nd can be recovered in a fraction containing <15% of Pr and <1% Ce. Although an overwhelming proportion of the REE were extracted by the DGA column under these conditions, the data of Table S3† show that minor amounts (in the 2–10% range) were found in the loading fractions. It is not clear at present whether these determinations are significant or have been biased to spuriously high values due to polyatomic interferences based on the relatively heavy elements Fe and Cl present in large amounts in these fractions. However, the data probably document a real leak of REE during the loading steps. In this case, the lost fraction would reflect the presence of non extractable species, likely fluorides. Further work showed that reducing the amount of HF by a factor of two while leaving the sample weight unchanged (100 mg) allows reducing the early loss of REE to less than 10% bringing a positive consequence on the Nd recovery (i.e. 90–95%).
Procedural blanks
Nd concentrations measured in the three acids used in the sample dissolution and Nd separation step are given in Table 4, along with column and total procedural blanks. It can be concluded that the contamination associated to the method is negligible with respect to the amount of Nd (typically in the 0.2–15 μg range) processed.
Table 4 Reagent, column and total procedural Nd blanks measured by ICP-QMS
|
Reagent blanks (pg mL−1) |
Used column blank (pg) |
Total procedural blank (pg) |
29 M HF |
6 M HCl |
14 M HNO3 |
H2O (18.2 MΩ cm) |
Range |
0.3–0.7 |
0.2–0.4 |
0.4–0.9 |
0.01–0.04 |
5–12 |
17–32 |
Mean ± 1SD |
0.4 ± 0.2 |
0.3 ± 0.1 |
0.6 ± 0.2 |
0.02 ± 0.02 |
9 ± 3 |
25 ± 6 |
Number |
3 |
2 |
3 |
4 |
5 |
7 |
Discussion
Sample decomposition
From an analytical point of view, a critical feature of the iron-rich, silicate sediments object of this study is the near absence of alkali earths from their bulk composition. For this reason, a major problem inherent in any dissolution with hydrofluoric acid is avoided, namely the formation of poorly soluble fluorides of Ca and Mg, and more complex species based on these elements.18 These precipitates sequester significant amounts of many trace elements of interest, including the REE, and they must be destroyed before subsequent analytical steps, by means of lengthy treatments with other acids, the most efficient of which (H2SO4 and HClO4) are not welcome.
No such problem arises with banded iron ores and laterites, essentially composed of silica, and iron oxides, +/− alumina, all soluble in HF–HCl mixtures. As a result, clear sample solutions are achieved, and they could be used without further treatment for isolating the REE from unwanted matrix elements, in so far as a separation procedure operating in HF–HCl mixture is made available.
Chemical separation
By virtue of their greatly improved selectivity, chemical separation methods based on extraction chromatography have largely superseded those using cation exchange.19 One of these methods, based on the ligand tetra-n-octyldiglycolamide (TODGA, hereafter abbreviated to DGA) is particularly attractive in the present case, for several reasons. First, and in contrast with the other widely used EXC resins TRU and RE Spec, operated in nitric media, the DGA resin is able to extract the REE in both HCl and HNO3. The LN resin, based on the classical HDEHP extractant can also be used in hydrochloric medium, but the distribution ratios of the light lanthanides are relatively small, and decrease drastically with increasing acid strength. Moreover, its selectivity against other several tri- and tetravalent elements, including iron, is relatively poor. On the contrary, the distribution ratios of the REE on the DGA resin increase as a function of increasing acid strength, and as the function of atomic number along the lanthanide series. When the resin is used in nitric acid medium, the extraction of calcium is fairly important, and it is necessary to use concentrated acid to get rid of that element.20 Although Ca is not a source of concern in the specific samples investigated in this work, available distribution data indicate that it is more weakly extracted from HCl medium.21
However, there is another, much more decisive reason to choose the DGA resin to separate the REE from iron-rich matrices. Indeed, the presence of macro amounts of Fe is not a source of concern but instead actually enhances the extraction. This synergistic effect was discovered by Horwitz et al.7 who found that the uptake of lanthanides and trivalent actinides increases by several orders of magnitude when the extractions takes place from HCl containing mg L−1 quantities of anionic chlorides, including ferric iron. Horwitz et al.7 further noticed that the synergistic effect persists, even when the amount of metal chloride exceeds the capacity of the resin. Based on these observations, it was decided to develop a separation scheme specifically adapted to iron-rich silicate rocks.
The elution behaviour of a large spectrum of unwanted major and trace elements is shown in Table S2.† It can be seen that ≥98% of the amount of most of them are found in the loading fractions plus initial 4 M HCl column rinse. This indicates that these elements (Be, V, Cr, Mn, Co, Ni, Cu, As, Rb, Sr, Zr, Cd, Sn, Cs, Ba, Hf, Pb, Bi and Th), are not extracted by the resin from the HF–HCl mixed medium. Likewise, 92 to 98% of several further trace elements (Na, Mg, Al, Ca, Sc, Ti, Zn, Ge, Nb, Mo, Sb and U) are recovered in the loading fraction, documenting an essentially passive behavior, while few elements such as Ga and Tl are significantly retained (ca. ≥70%).
The behaviour of iron is particularly noteworthy. As observed visually during the experiment, and can be checked from the results of the four successive loading fractions, iron was extracted, but the column was soon saturated, since about 80% of Fe(III) occur in the three last ml of sample loading. Nevertheless, some 90% of the REE were extracted by the resin. Therefore, the observation of Horwitz et al. that the synergistic effect persists even when the amount of iron chloride exceeds the capacity of the resin is confirmed. It was noticed that Ga and Tl, two other elements forming very stable chloride complexes, are also significantly retained. Following the mechanism proposed by Horwitz et al., this is interpreted to reflect the strong co-extraction of their tetrachloro-complexes, in replacement of the Cl− counter ion required for charge neutrality in the Ln–DGA complex. As Fe, these elements were readily released from the resin when the column was rinsed with nitric acid.
It is further observed that, even in the presence of a fairly high concentration of HF, the DGA resin is still able to extract most of the lanthanides from ca. 4 M HCl containing large amounts of Fe(III). However, it is most likely that fluoride complexing was responsible for the early loss of some 10–20% of the lanthanides during the column loading step. Whether this lost component reflects extremely fine-grained insoluble compounds (not removed by centrifugation in the absence of alkaline earth-based carriers) or soluble molecular species, not extractable by the diglycolamide ligand, or both, is not clear at present. However, additional experiments show that a twofold decrease of the amount of HF used for dissolution (that is, 1 ml for 0.1 g sample) permits to reduce the early loss of lanthanides to less than 10%. Thus, provided a too large excess of HF is not used for dissolving the samples, the important saving of time inherent in the direct loading of the column with the HCl–HF mixture has a rather limited cost in terms of chemical yield. The next fractions of nitric acid achieve a rapid stripping of the ca. 30% of iron that were initially extracted, while the REE are still strongly held by virtue of the relatively high (3 M) acid strength. At this stage, almost all matrix elements have been removed. For the subsequent steps, hydrochloric acid is used again, because it allows the sequential elution of the LREE to be achieved,22–24 as depicted in Fig. 1. The lanthanides heavier than Nd are still retained on the column, and need more and more dilute HCl to be stripped from the DGA resin. This can be made by using 0.7 M HCl for Sm, and then 0.05 M HCl in order to elute as a group the M- and HREE. Finally, washing the column with a dilute HCl–HF mixture strips the rest of the few elements still present on the column, mainly Th. The comparison of the elution profiles (Fig. 1) obtained for the two studied international SRMs, and an additional BIF sample from the Eastern Indian Craton, even richer in iron (BIF-2, 59.3 wt% Fe2O3), shows that the position of the elution peaks of the individual LREE is quite stable, thereby demonstrating the robustness of the method in the 20–60 wt% range of total iron as Fe2O3. It is further noticed that, in all cases, the Nd peak is well resolved from those of Sm and Ce, while the overlap by the tail of the Pr peak can be reduced to <10% without important loss of Nd. This is a noteworthy achievement for a single column chromatographic separation.
Using a single column of DGA resin for separating Nd is not new22–24 but, as far as we are aware, our protocol is the first to load the sample onto the column in hydrochloric acid medium, an approach particularly well suited for high-Fe samples. Moreover, in so far as the samples are sufficiently poor in alkaline earth elements, such as the rocks targeted by this study, the HF–HCl solution resulting from the sample decomposition can be directly loaded onto the column, without any further treatment. This feature of our protocol alleviates the need for time-consuming evaporation to dryness of the dissolved sample and repeated treatments of the residue with nitric acid, thereby making the whole procedure extremely straightforward.
Indeed, following sample weighing and the addition of HCl and HF acids (taking a few minutes per sample), dissolution is made overnight. After centrifugation of the resulting solution (some 10 minutes), the column separation procedures takes less than 5 hours only. Thus, starting from the sample powder, 6 to 12 neodymium fractions ready for MC-ICP-MS can be obtained in less than 24 hours with relatively little analyst time and effort.
Conclusion
The aim of this work was to develop a new procedure based on the unique properties of the diglycolamide-based EXC resin (DGA) in hydrochloric acid medium containing large amounts of ferric ion7 and evaluate its potential for geochemical applications, specifically, 143Nd/144Nd isotope ratio analyses. The resulting protocol enables the analyst to separate in a very straightforward way the lanthanides from iron-rich silicate materials such as banded iron ores or lateritic samples, and to isolate in a single step a Nd fraction suitable for isotope ratio measurements by MC-ICP-MS. The method is extremely rapid, by virtue of two major features: (i) the solution obtained after sample dissolution with an HF–HCl mixture is directed to the DGA EXC column for separation without any intervening step, thereby circumventing the time-consuming evaporation and conversion steps inherent in conventional methods; and (ii) the separation is performed by using a single, relatively short column allowing fast, gravity driven flow rates. Besides its obvious interest in terms of analyst labor, the method is very simple, as it involves only three most common minerals acids and does not require a fume hood or any sophisticated piece of equipment. In addition, it is believed that it might be extended rather easily to other radiogenic isotopic systems involving lanthanide elements. Indeed, La and Ce might be recovered ahead of Nd and further purified from each other by using conventional HDEHP or DGA resins, or a combination thereof. Likewise, after evaporation to dryness to remove silicon, the loading + rinse fraction containing Fe(III) and most of the accompanying elements might be used to separate other elements of radiogenic isotope interest by EXC, as described elsewhere.25 Finally, it is suggested that the procedure outlined in this paper might deserve further work to evaluate its potential for processing iron-rich meteorites (siderites, pallasites).
Author contributions
A. G. and C. P. designed the analytical procedure, carried out the early tests and isotopic measurements on the standard reference materials. K. S. prepared and analysed the trace element data. A. G. and C. P. wrote the main paper. All authors discussed the results and commented on the manuscript at all stages.
Conflicts of interest
There are no conflicts to declare.
Acknowledgements
We thank Chantal Bosq for preparing high purity acids and Jordan Wright for providing the BIF-2 sample used during the setup of our procedure. We also thank Mhammed Benbakkar for major element analyses on the ICP-AES. We thank three anonymous referees for their perceptive reviews which led to significant improvements of the manuscript and Ziva Whitelock for editorial handling. We thank the Geological Survey of Japan for providing the isotopic standard JNdi-1. This research was financed by the French Government Laboratory of Excellence Initiative n°ANR-10-LABX-0006, the Région Auvergne and the European Regional Development Fund. This is Laboratory of Excellence ClerVolc contribution N° 514.
References
-
A. Trendall, in Encyclopedia of Geology, ed. R. Selley, R. Cocks and I. Plimer, Elsevier, Academic Press, 2005, pp. 37–42 Search PubMed.
-
A. Bekker, N. J. Planavsky, B. Krapez, B. Rasmussen, A. Hofmann, J. F. Slack, O. J. Rouxel, K. O. Konhauser, in Treatise on Geochemistry, ed. H. Holland and K. Turekian, Elsevier, Netherlands, 2014, vol. 9, pp. 561–628 Search PubMed.
- P. Cloud, Econ. Geol., 1973, 68, 1135–1143 CrossRef CAS.
-
G. J. J. Aleva, Laterite, Concepts, Geology, Morphology, and Chemistry,ISRIC, Netherlands, 1994 Search PubMed.
-
Y. Tardy, Petrology of Laterites and Tropical Soils, Taylor and Francis group, Netherlands, 1997 Search PubMed.
-
A. D. Dalvi, W. G. Bacon and R. C. Osborne, The Past and the Future of Nickel Laterites.PDAC International Convention, Trade Show and Investors Exchange, 2004, pp. 1–27 Search PubMed.
- E. P. Horwitz, D. R. McAlister and A. H. Thakkar, Solvent Extr. Ion Exch., 2008, 26, 12–14 CrossRef CAS.
- K. Govindaraju, Geostand. Newsl., 1995, 19, 55–96 CrossRef CAS.
- K. Govindaraju and I. Roelandts, Geostand. Newsl., 1989, 13, 5–67 CrossRef CAS.
- B. W. Alexander, M. Bau and P. Andersson, Earth Planet. Sci. Lett., 2009, 283, 144–155 CrossRef CAS.
- S. Viehmann, M. Bau, B. Bühn, E. L. Dantas, F. R. D. Andrade and D. H. G. Walde, Precambrian Res., 2016, 282, 74–96 CrossRef CAS.
- G. Caro, P. Morino, S. J. Mojzsis, N. L. Cates and W. Bleeker, Earth Planet. Sci. Lett., 2017, 457, 23–37 CrossRef CAS.
-
P. Morino, PhD thesis, University of Lorraine, 2017.
- T. Tanaka, S. Togashi, H. Kamioka, H. Amakawa and H. Kagami, Chem. Geol., 2000, 168, 279–281 CrossRef.
- C. R. M. McFarlane and M. T. McCulloch, Chem. Geol., 2007, 245, 45–60 CrossRef CAS.
- C. Fisher, C. R. M. McFarlane, J. M. Hanchar, M. D. Schmitz, P. J. Sylvester, R. Lam and H. P. Longerich, Chem. Geol., 2011, 284, 1–20 CrossRef CAS.
- M. E. Sánchez-Lorda, S. García de Madinabeitia, C. Pin and J. I. Gil Ibarguchi, Int. J. Mass Spectrom., 2013, 333, 34–43 CrossRef.
-
Z. Šulcek and P. Povondra, Methods of Decomposition in Inorganic Analysis. CRC Press, Boca Raton, Fl, USA, 1989 Search PubMed.
-
C. Pin and J. Rodriguez, in Treatise on Geochemistry, ed. H. Holland and K. Turekian, Elsevier, Netherlands, 2014, vol. 15, pp. 147–170 Search PubMed.
- A. Pourmand and N. Dauphas, Talanta, 2010, 81, 741–753 CrossRef CAS PubMed.
- E. P. Horwitz, D. R. McAlister, A. H. Bond and R. E. Barrans Jr, Solvent Extr. Ion Exch., 2005, 23, 319–344 CrossRef CAS.
- Y. Wang, X. Huang, Y. Sun, S. Zhao and Y. Yue, Anal. Methods, 2017, 9, 3531–3540 RSC.
- A. Retzmann, T. Zimmermann, D. Pröfrock, T. Prohaska and J. Irrgeher, Anal. Bioanal. Chem., 2017, 409, 5463–5480 CrossRef CAS PubMed.
- Z.-Y. Chu, M.-J. Wang, C.-F. Li, Y.-H. Yang, J.-J. Xu, W. Wang and J.-H. Guo, J. Anal. At. Spectrom., 2019, 34, 2053–2060 RSC.
- C. Pin and A. Gannoun, Anal. Chem., 2017, 89, 2411–2417 CrossRef CAS PubMed.
Footnote |
† Electronic supplementary information (ESI) available. See DOI: 10.1039/d1ja00397f |
|
This journal is © The Royal Society of Chemistry 2022 |
Click here to see how this site uses Cookies. View our privacy policy here.