DOI:
10.1039/D1MA00866H
(Paper)
Mater. Adv., 2022,
3, 224-231
Room temperature synthesis of a luminescent crystalline Cu–BTC coordination polymer and metal–organic framework†
Received
20th September 2021
, Accepted 15th November 2021
First published on 22nd November 2021
Abstract
Synthesis of crystalline materials is elemental in the field of coordination chemistry towards optical applications. In the present work, coordination between copper and benzene-1,3,5-tricarboxylic acid (BTC) is controlled by adjusting the pH scale of the reaction mixture at room temperature to synthesize two crystalline structures: metal–organic framework HKUST-1 and coordination polymer Cu(BTC)·3H2O. The post-synthesis transformation of HKUST-1 into Cu(BTC)·3H2O is further demonstrated. Single crystals of both structures are studied by multi-laser Raman and luminescence spectroscopy. It is found that both crystals exhibit photoluminescence in the range of 700–900 cm−1 within the optical gap of the bulk materials, which can be associated with crystallographic defects. This work gives impetus for the synthesis of large metal–organic crystals based on which optical properties can be studied in depth.
1 Introduction
Organic coordination compounds consist of organic ligands (Lewis base) bound to a central metal ion (Lewis acid).1–4 The unique and diverse properties of coordination compounds have been proved to be beneficial in the field of electronics, sensors, magnetism and medical research. Coordination polymers are solid-state structures consisting of repeating coordination units to form 1D extended chains, 2D sheets or 3D frameworks.5–8 Coordination polymers having a porous structure are known as metal–organic frameworks (MOFs).9–16 Synthesizing large and quality crystals of MOFs benefits in a wide range of applications such as separation, heterogeneous catalysis,17–19 gas-sorption,20,21 chromatography,22,23 optoelectronics, magnetism and sensing applications.24,25
Controlling the process of crystallization by pH adjustment is a challenging, yet fascinating process in understanding the chemistry of materials.26–28 The pH modulating technique was previously utilized to synthesize MOFs.29–34
In the present work, the reaction between copper and benzene-1,3,5-tricarboxylic (BTC) acid is controlled by adjusting the pH of the reaction mixture to obtain crystals of two different structures: Cu3(BTC)2(H2O)3 (also known as HKUST-135 or MOF-199) and Cu(BTC)·3H2O (see Fig. 1).
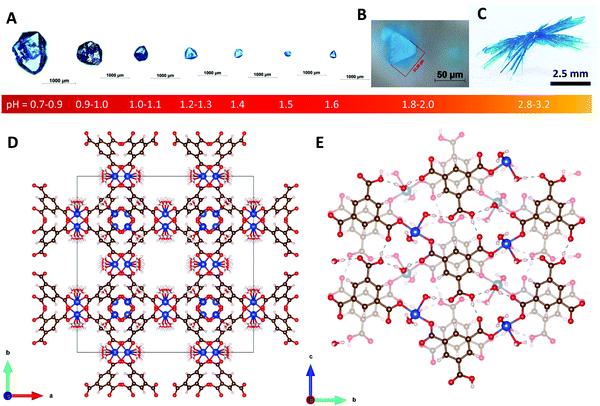 |
| Fig. 1 (A) Seven HKUST-1 crystals synthesized with HNO3 in the pH range 0.7–1.6. (B) HKUST-1 crystal synthesized without any pH adjustment. (C) Cu(BTC)·3H2O crystals synthesized in the pH range 2.8–3.2. (D) Crystallographic representation of HKUST-1. (E) Crystallographic representation of the Cu(BTC)·3H2O coordination polymer viewed along the [100] crystallographic axis. | |
HKUST-1 is typically synthesized using a hydrothermal/solvothermal method. The high-temperature synthesis yields larger crystals, but the problem is that a significant amount of Cu2O is obtained as a by-product. This by-product remains in the pores of the framework and reduces the specific surface area thereby causing hinderance in gas absorption.36 Thus, room-temperature synthesis is beneficial. Our pH-controlled synthesis procedure will help the community to synthesize quality and large crystals of HKUST-1 at room temperature.
In turn, copper(II) based coordination polymers are some of the well studied compounds in the field of natural science and medical research. Owing to the antibacterial activity, low toxicity and potential as biomaterials, these compounds are gaining interest among researchers.37 In the present work, large crystals of Cu(BTC)·3H2O are synthesized at room temperature under weak acid conditions i.e. pH greater than 2.1. Single crystal X-ray diffraction (SXRD) reveals that the structure is composed of 1D zig-zag chains interconnected by hydrogen bonding which makes the structure stable and generates a layered coordination network.38
The knowledge acquired through the pH-controlled synthesis helps us transform the porous HKUST-1 framework into Cu(BTC)·3H2O. This process is particularly useful as MOFs sustain the porosity capable of absorbing harmful gases which can be a threat to the environment when the MOFs decompose. Our technique to use water is a fast, efficient and economical method of transforming the framework into non-porous coordination solids for safe disposal.
Finally, luminescent properties of both the HKUST-1 framework and Cu(BTC)·3H2O are examined on their single crystals with multiple visible lasers. It is found that both crystals luminesce in the range 700–900 cm−1 with a red excitation laser, that can be attributed to crystallographic defects.
2 Results and discussions
All synthesis demonstrated in this work is done at room temperature. It is found that crystals of HKUST-1 can be synthesized by adjusting the pH of the reaction mixture in the pH range 0.7–1.6 as shown in Fig. 1A, whereas crystals of Cu(BTC)·3H2O can be synthesized in the pH range 2.2–3.2 as shown in Fig. 1C and 2B–D.
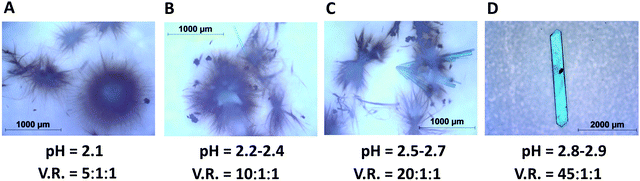 |
| Fig. 2 Crystals and whiskers of Cu(BTC)·3H2O synthesized at different pH scales. V.R. stands for the volume ratio of three solvents (deionised water, DMF, and ethanol). | |
2.1 Synthesis of HKUST-1
First, we present room temperature synthesis of HKUST-1 (crystal size 50–55 micrometers) without additional pH adjustment. Copper nitrate trihydrate CuNO3·3H2O (1 mmol) and BTC (1 mmol) is dissolved in a mixture of three solvents (deionised water 4 ml, DMF 4 ml and ethanol 4 ml) i.e. in a volume ratio (V.R.) of 1
:
1
:
1 (pH = 1.8–2.0). After 16–20 hours, crystals of HKUST-1 are formed at the bottom of the vial (pH = 2.1). A clear depiction of the morphology of the as-synthesized HKUST-1 crystal is shown in Fig. 1B. The crystal size is approximately 50 μm across. The homogeneity range is shown in Fig. S1 in the ESI.† An SXRD analysis confirms the well-known structure of HKUST-1 MOF as depicted in Fig. 1D. See the ESI† for more details.
Much larger crystals can be obtained in more acidic conditions prepared by adding 0.1 ml of nitric acid (HNO3) in the aforementioned 12 ml reaction mixture of V.R. = 1
:
1
:
1. The resulting pH scale is in the range 0.7–0.9. After 8–10 days, crystals of HKUST-1 can get as large as ∼1–1.2 mm, as shown in Fig. 1A. More examples of such crystals are shown in Fig. S2 in the ESI.† The size can be controlled by adjusting the pH of the reaction mixture in the range from 0.7 to 2.0, as shown in Fig. 1A. Similarly, large crystals of HKUST-1 in the size range ∼0.9–1.0 mm can also be obtained by using 37% hydrochloric acid (HCl) instead of HNO3 and copper(II) chloride (CuCl2) instead of CuNO3·3H2O. Their crystal size and morphology are shown in Fig. S3 in the ESI.†
It has been reported previously that large crystals of MOFs can be synthesized via slow reactions.22,39 Specifically, large crystals of HKUST-1 could be synthesized in acidic conditions.23 Deprotonation of BTC is a prerequisite for HKUST-1 growth. In our pH dependent synthesis, HKUST-1 crystals grow larger when a strong acid, HCl or HNO3, is added in the reaction mixture. The increased amount of H+ in the mixture inhibits the deprotonation of the BTC ligand. A reduced amount of BTC3− anion leads to slowing down the nucleation and further growth of fewer crystals may be possible.
2.2 Synthesis of Cu(BTC)·3H2O
When the pH is adjusted to exceed 2.1 by adding excess water in the reaction mixture to get a volume ratio of V.R. = 5
:
1
:
1, another type of crystal is formed. Fig. 1C shows this large crystal obtained 2–3 weeks after mixing the precursors. SXRD analysis reveals the structure of Cu(BTC)·3H2O as shown in Fig. 1E. At pH = 2.1, tiny whiskers appear in 3–4 hours as shown in Fig. 2A. This indicates the point of nucleation. When the pH is increased to 2.2–2.4 (V.R. = 10
:
1
:
1), both tiny whiskers and bar-shaped crystals grow in 12–16 hours as shown in Fig. 2B. A further increase in pH to 2.5–2.7 (V.R. = 20
:
1
:
1) accelerates the crystal growth, resulting in more bar-shaped crystals observed in 16–20 hours as shown in Fig. 2C. At pH = 2.8–2.9 (V.R. = 45
:
1
:
1), the crystal growth is optimized for the formation of only large bar-shaped crystals as shown in the micrograph taken after 18–22 hours in Fig. 2D.
HKUST-1 is not stable in this condition to be demonstrated as the transformation of HKUST-1 to Cu(BTC)·3H2O in Section 2.4. SXRD evidences that two of the three carboxyl groups of the BTC ligand are coordinated to the Cu2+ ion and form zig-zag chains that are linked via hydrogen bonding facilitated by the third uncoordinated/protonated carboxyl group and coordinated water molecules (see Fig. 3). The stability of the structure relies on hydrogen bonding of the copper-coordinated water molecules and the pi stacking of the BTC ligands.38
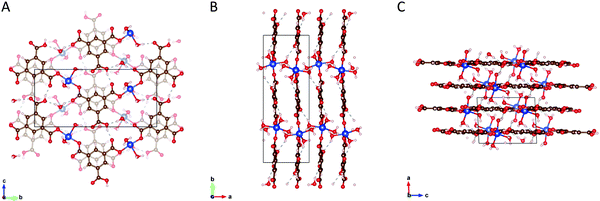 |
| Fig. 3 Panels (A, B and C) shows the structures of the Cu(BTC)·3H2O coordination polymer along the [100], [001] and [010] crystallographic planes, respectively. | |
2.3 Structure of Cu(BTC)·3H2O
The crystallographic representations of the Cu(BTC)·3H2O packing structure along the [100], [001] and [010] crystallographic axes are shown in Fig. 3A–C, respectively. For further and detailed information regarding the structure of HKUST-1 and Cu(BTC)·3H2O, see Section S2 in the ESI.†
The crystal structure is monoclinic with space group P21/n and lattice parameters a = 6.7654, b = 18.8135, c = 8.5144; α = γ = 90°, β = 92.439°. The empirical formula of the structure is C9H10CuO9. This structure was previously reported.37,38,40–42Fig. 3A shows the 2D layer of Cu–BTC. Each copper is coordinated to two BTC and three water molecules in the distorted square pyramidal or pentacoordinate geometry.38,42 BTC consists of three carboxyl groups attached to the benzene ring. Two of the three carboxyl groups of the ligand are coordinated to the metal ion via one oxygen to form the zig-zag Cu–BTC polymeric chain. The other oxygen atoms contribute to the hydrogen bonding (dotted line) between the Cu–BTC chains. The Cu–BTC layers are held together by a combination of hydrogen bonding (dotted line) and π–π stacking of the BTC ligand (see Fig. 3B and C). The distance between the adjacent planes is approximately 3.4 Å.
2.4 Transformation of HKUST-1 to Cu(BTC)·3H2O
One of the major concerns in MOF research is that the porous framework can absorb harmful gases. These harmful gases trapped in the pores of the framework can cause threats to the environment. Our results strongly suggest that post-synthesis transformations between HKUST-1 and Cu(BTC)·3H2O can be possible by adjusting the pH of the reaction mixture. Indeed, when crystals of HKUST-1 are immersed in deionised water to get the pH range 2.8–3.2 suitable for obtaining Cu(BTC)·3H2O (see Fig. 2D), the HKUST-1 crystals transform into Cu(BTC)·3H2O in 18–22 hours. The reason behind this conversion is that the water molecule coordinates the metal site of the framework which results in increased coordination around the metal site. As a result, hydrolysis takes place and the organic ligand (BTC) is displaced away from the framework. Ultimately, the metal hydroxide remains along with the protonated linker.42
2.5 Raman spectroscopy
Fig. 4 shows the Raman spectra of HKUST-1 and Cu(BTC)·3H2O measured with a green laser (wavelength λ = 514.5 nm) focused on the single crystals in the insets. For HKUST-1, the bands appearing in the low frequency region i.e. below 600 cm−1 is due to the copper ions in the framework. The doublet at 177.54 cm−1 and 197.63 cm−1 indicates that the MOF is hydrated and correspondingly Cu–Cu stretching modes appear.43–45 The band at 275.49 cm−1 is indicative of the Cu–Ow stretching vibration, where Ow represents the oxygen of the water molecule adsorbed on Cu2+ ions.
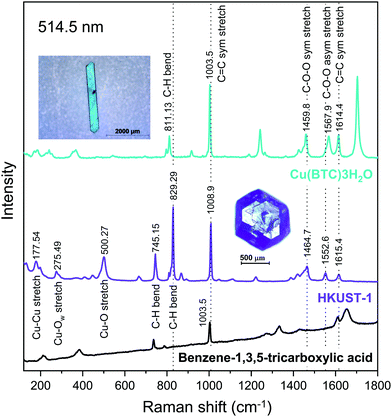 |
| Fig. 4 Raman spectra of HKUST-1 and Cu(BTC)·3H2O measured at λ = 514.5 nm. The insets show the measured single crystals of HKUST-1 and Cu(BTC)·3H2O. | |
The bands in the range 700–1800 cm−1 are mostly due to vibrational modes of the BTC ligand. The C
C stretching modes of the benzene ring are found at 1008.9 and 1615.4 cm−1 for HKUST-1,43,44,46–48 and at 1003.5 and 1614.4 cm−1 for Cu(BTC)·3H2O. The peaks at 745.15 and 829.29 cm−1 for HKUST-1 can be attributed to the C–H bending modes.43,44 The corresponding peak for Cu(BTC)·3H2O is located at 811.13 cm−1. The peaks assigned to the C–O–O symmetric and C–O–O asymmetric stretching modes are centered at 1464.7 and 1552.6 cm−1, respectively, for HKUST-1,48,49 and at 1459.8 and 1567.9 cm−1 for Cu(BTC)·3H2O.
2.6 Photoluminescence spectroscopy
Luminescence is a key property of MOFs that can lead to their potential applications in optoelectronic devices.50–52 The luminescence from HKUST-1 and Cu(BTC)·3H2O single crystals has been examined using seven different excitation lasers. The photoluminescence spectra of HKUST-1 and Cu(BTC)·3H2O crystals measured at laser wavelengths λ = 458, 488, 514.5, 531, 568, 633 and 647 nm are shown in Fig. 5. All spectra are normalized by the highest Raman peak. The luminescence peak of HKUST-1 appears in the range 675–1000 nm in the spectra measured at λ = 633 and 647 nm (Fig. 5A). Similarly, the spectra of Cu(BTC)·3H2O recorded at λ = 633 and 647 nm exhibit the luminescence peak in the same range 675–1000 nm (Fig. 5B). In contrast to HKUST-1, the other luminescence peak is observed in the range 458–600 nm at λ = 458 nm, that can be associated with the optical band gap of the Cu(BTC)·3H2O (see the UV-Vis spectra in Fig. 6). In both cases, the luminescence intensity at λ = 647 nm increases linearly with the laser power, and no saturation has been observed up to a half milliwatts as shown in the insets of Fig. 5 (see the corresponding power-dependent spectra in Fig. S12A and B in the ESI†). The highly consistent luminescence spectra of HKUST-1 and Cu(BTC)·3H2O at λ = 647 nm suggest similar types of defects present in both crystals.
 |
| Fig. 5 (A) Photoluminescence spectra (Raman spectra plotted as a function of wavelength) of a HKUST-1 crystal measured on the same spot using seven different lasers λ = 458, 488, 514.5, 531, 568, 633 and 647 nm with laser powers of 0.109, 3.78, 0.617, 0.0712, 1.10, 1.72 and 0.617 mW, respectively. All spectra were normalized by the highest Raman peak. (B) Photoluminescence spectra of a Cu(BTC)·3H2O crystal measured on the same spot using seven different lasers λ = 458, 488, 514.5, 531, 568, 633 and 647 nm with laser powders of 0.105, 1.37, 0.565, 0.077, 0.708, 1.20, and 0.815 mW, respectively. All spectra were normalized by the highest Raman peak. (insets) The photoluminescence intensity plotted against the laser power at λ = 647 nm. | |
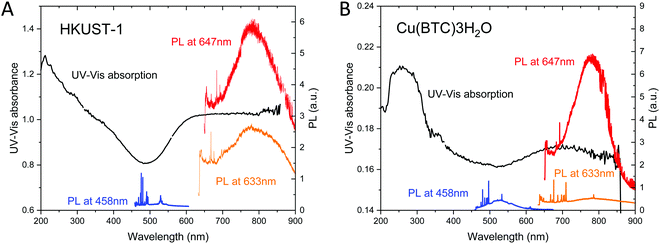 |
| Fig. 6 (A) UV-Vis absorption spectrum of HKUST-1 (black curve). (B) UV-Vis absorption spectrum of Cu(BTC)·3H2O (black curve). Their photoluminescence (PL) spectra measured at λ = 458, 633, and 647 nm are also plotted in blue, orange and red, respectively. | |
Crystallographic defects play vital roles as far as the visible-range optical properties of wide-bandgap MOFs are concerned. Types of defects in HKUST-1 reported thus far include plane dislocations with free COOH groups,53,54 dislocation growth spirals,55,56 fractures propagating in the crystal interior,53 monovalent copper,54,57,58 metal vacancy,59 linker vacancy,60 defective linkers,61–63 and temporary defects as Brønsted sites.64 Some defects can be caused post synthesis by exposure to moisture.42,65 Methods to avoid defects and reconstruct structural defects were reported.42,66
Defects can modify the electronic structure of HKUST-1. The reported primary optical band gap of hydrated HKUST-1 is in the range 3–4 eV (approximately 300–400 nm),57,58,67–70 which can be blue-shifted in defect-engineered HKUST-157 or by dehydration.70
Fig. 6A shows the UV-Vis spectrum of HKUST-1 crystals. It exhibits the absorption at wavelengths below 450 nm and above 550 nm. The former corresponds to the reported band gap of hydrated HKUST-1, while the latter can be associated with defects. This weak low-frequency visible absorption can be associated with d–d transitions at defective copper pairs leading to the blue colour of defective HKUST-1.58 This indicates the presence of defects either in the bulk or on the surface of HKUST-1. The luminescence in the wavelength range 675–1000 nm is excited at laser wavelengths of 633 nm (1.96 eV) and 647 nm (1.92 eV) that is in resonance with the optical band gap of defects.58,70
Fig. 6B shows the UV-Vis spectrum of Cu(BTC)·3H2O crystals. The photoluminescence spectra of Cu(BTC)·3H2O measured at λ = 458, 633 and 647 nm are superposed onto the UV-Vis absorption spectrum. To the best of our knowledge, there have been no reports of UV-Vis absorption and luminescence spectroscopy on Cu(BTC)·3H2O. The UV-Vis spectrum shows the strong UV absorption at wavelengths below 400 nm and the broad absorption above 600 nm. The long-wavelength tail of the UV absorption coincides with the luminescence excited at λ = 458 nm. The broad band above 600 nm leads to the luminescence observed at λ = 647 nm. The much weaker luminescence at 633 nm as compared with HKUST-1 can be attributed to the lower-wavelength absorption onset.
3 Conclusions
The present work has demonstrated that the reaction between copper and benzene-1,3,5-tricarboxylic (BTC) acid can be controlled by adjusting the pH of the precursor solution to obtain millimeter-sized crystals of two different coordination compounds, namely, metal–organic framework HKUST-1 and coordination polymer Cu(BTC)·3H2O. Crystals of HKUST-1 can be synthesized in the pH range 0.7–0.9, whereas Cu(BTC)·3H2O in the pH range 2.8–3.2. The post-synthesis transformation from HKUST-1 to Cu(BTC)·3H2O is possible, which can be useful as means for environmentally-friendly disposal of the MOF. Both species are found to be luminescent. HKUST-1 exhibits luminescence in the range 675–1000 nm that can be associated with crystallographic defects. Cu(BTC)·3H2O in turn is luminescent in the ranges 458–600 nm and 675–1000 nm, that are in-line with the UV-Vis absorption spectrum. The present work demonstrates band-gap engineering by the coordination chemistry and defect-induced luminescence in bulky crystals, that in unison will pave the way for optical applications of coordination polymers and MOFs.
Conflicts of interest
There are no conflicts to declare.
Acknowledgements
This work was financially supported by the Austrian Science Fund (FWF), project no. P30431-N36, the Czech Science Foundation (GACR), project no. 19-15217S, the Austrian Federal Ministry of Education, Science and Research (BMBWF) and OeAD-GmbH through Scientific & Technological Cooperation (WTZ) program, project no. CZ 18/2019, and the Ministry of Education, Youth and Sports of the Czech Republic (MEYS) through V4-Japan joint research program, project no. 8F21010. We thank S. Loyer and A. Stangl for technical assistance.
References
- T. Damhus, R. M. Hartshorn, A. T. Hutton and N. G. Connelly, Nomenclature of inorganic chemistry - iupac recommendations, Chem. Int., 2005, 27(6), 25–26 Search PubMed
.
- M.ichael O'Keeffe, M.axim A. Peskov, S.tuart J. Ramsden and O.mar M. Yaghi, . The Reticular Chemistry Structure Resource (RCSR) Database of, and Symbols for, Crystal Nets, Acc. Chem. Res., 2008, 41(Suppl. 12), 1782–1789 CrossRef PubMed
.
- S. R. Batten, N. R. Champness, X.-M. Chen, J. Garcia-Martinez, S. Kitagawa, L. Ohrstrom, M. O'Keeffe, M. Paik Suh and J. Reedijk, . Coordination polymers, metal-organic frameworks and the need for terminology guidelines, CrystEngComm, 2012, 14(9), 3001–3004 RSC
.
-
R. R. Crichton, Basic coordination chemistry for biologists, in Biological Inorganic Chemistry, ed. R. R. Crichton, Elsevier, Oxford, 2nd edn, 2012, ch. 2, pp. 21–34 Search PubMed
.
-
S. R. Batten, S. M. Neville and D. R. Turner, Coordination polymers: Design, analysis and application, Royal Soc Chemistry, Thomas Graham House, Science park, Cambridge, CB4 4WF, CAMBS, England, 2009, pp. 1–424 Search PubMed
.
- S. R. Batten, N. R. Champness, X.-M. Chen, J. Garcia-Martinez, S.usumu Kitagawa, L. Ohrstrom, M. O'Keeffe, M. Paik Suh and J. Reedijk, Terminology of metal–organic frameworks and coordination polymers (IUPAC Recommendations 2013), Pure Appl. Chem., 2013, 85(8), 1715–1724 CAS
.
- L. Ohrstrom, Let's Talk about MOFs-Topology and Terminology of Metal-Organic Frameworks and Why We Need Them, Crystals, 2015, 5(1), 154–162 CrossRef
.
- E. R. Engel and J. L. Scott, Advances in the green chemistry of coordination polymer materials, Green Chem., 2020, 22(12), 3693–3715 RSC
.
- J. L. C. Rowsell and O. M. Yaghi, Metal-organic frameworks: a new class of porous materials, Microporous Mesoporous Mater., 2004, 73(1-2), 3–14 CrossRef CAS
.
- J. R. Long and O. M. Yaghi, The pervasive chemistry of metal-organic frameworks, Chem. Soc. Rev., 2009, 38(5), 1213–1214 RSC
.
- K. Biradha, A. Ramana and J. J. Vittal, Coordination Polymers Versus Metal–Organic Frameworks, Cryst. Growth Des., 2009, 9(7), 2969–2970 CrossRef CAS
.
- H. Li, M. Eddaoudi, M. O'Keeffe and O. M. Yaghi, Design and synthesis of an exceptionally stable and highly porous metal–organic framework, Nature, 1999, 402(6759), 276–279 CrossRef CAS
.
- A. K. Cheetham and C. N. R. Rao, There's room in the middle, Science, 2007, 318(5847), 58–59 CrossRef CAS PubMed
.
- C. Janiak and J. K. Vieth, MOFs, MILs and more: concepts, properties and applications for porous coordination networks (PCNs), New J. Chem., 2010, 34(11), 2366–2388 RSC
.
- Z.-G. Gu, D.-J. Li, C. Zheng, Y. Kang, C. Woell and J. Zhang, MOF-Templated Synthesis of Ultrasmall Photoluminescent Carbon-Nanodot Arrays for Optical Applications, Angew. Chem., Int. Ed., 2017, 56(24), 6853–6858 CrossRef CAS PubMed
.
- Z.-G. Gu and J. Zhang, Epitaxial growth and applications of oriented metal-organic framework thin films, Coord. Chem. Rev., 2019, 378(SI), 513–532 CrossRef CAS
.
- A. Dhakshinamoorthy, M. Alvaro and H. Garcia, Commercial metal-organic frameworks as heterogeneous catalysts, Chem. Commun., 2012, 48(92), 11275–11288 RSC
.
- A. Corma, H. Garcia and F. X. L. I. Llabres i Xamena, Engineering Metal Organic Frameworks for Heterogeneous Catalysis, Chem. Rev., 2010, 110(8), 4606–4655 CrossRef CAS PubMed
.
- Y. Wen, J. Zhang, Q. Xu, X.-T. Wu and Q.-L. Zhu, Pore surface engineering of metal-organic frameworks for heterogeneous catalysis, Coord. Chem. Rev., 2018, 376, 248–276 CrossRef CAS
.
- R. Babarao, J. Jiang and S. I. Sandler, Molecular Simulations for Adsorptive Separation of CO2/CH4 Mixture in Metal-Exposed, Catenated, and Charged Metal–Organic Frameworks, Langmuir, 2009, 25(9), 5239–5247 CrossRef CAS PubMed
.
- O. Zybaylo, O. Shekhah, H. Wang, M. Tafipolsky, R. Schmid, D. Johannsmann and C. Woell, A novel method to measure diffusion coefficients in porous metal-organic frameworks, Phys. Chem. Chem. Phys., 2010, 12(28), 8092–8097 RSC
.
- S. Han, Y. Wei, C. Valente, I. Lagzi, J. J. Gassensmith, A. Coskun, J. F. Stoddart and B. A. Grzybowski, Chromatography in a Single Metal-Organic Framework (MOF) Crystal, J. Am. Chem. Soc., 2010, 132(46), 16358–16361 CrossRef CAS PubMed
.
- L. Li, F. Sun, J. Jia, T. Borjigin and G. Zhu, Growth of large single MOF crystals and effective separation of organic dyes, CrystEngComm, 2013, 15(20), 4094–4098 RSC
.
- J. W. Brown, B. L. Henderson, M. D. Kiesz, A. C. Whalley, W. Morris, S. Grunder, H. Deng, H. Furukawa, J. I. Zink, J. F. Stoddart and O. M. Yaghi., Photophysical pore control in an azobenzene-containing metal-organic framework, Chem. Sci., 2013, 4(7), 2858–2864 RSC
.
- J. A. Mason, M. Veenstra and J. R. Long, Evaluating metal-organic frameworks for natural gas storage, Chem. Sci., 2014, 5(1), 32–51 RSC
.
- N. D. Burrows, C. R. H. Hale and R. Lee Penn, Effect of pH on the Kinetics of Crystal Growth by Oriented Aggregation, Cryst. Growth Des., 2013, 13(8), 3396–3403 CrossRef CAS
.
- N. Fomina, C. A. Johnson, A. Maruniak, S. Bahrampour, C. Lang, R. W. Davis, S. Kavusi and H. Ahmad, An electrochemical platform for localized pH control on demand, Lab Chip, 2016, 16(12), 2236–2244 RSC
.
- R.-Q. Chen, Q.-D. Cheng, J.-J. Chen, D.-S. Sun, L.-B. Ao, D.-W. Li, Q.-Q. Lu and D.-C. Yin, An investigation of the effects of varying pH on protein crystallization screening, CrystEngComm, 2017, 19(5), 860–867 RSC
.
- C. Gabriel, M. Perikli, C. P. Raptopoulou, A. Terzis, V. Psycharis, C. Mateescu, T. Jakusch, T. Kiss, M. Bertmer and A. Salifoglou, pH-Specific Hydrothermal Assembly of Binary and Ternary Pb(II)-(O,N-Carboxylic Acid) Metal Organic Framework Compounds: Correlation of Aqueous Solution Speciation with Variable Dimensionality Solid-State Lattice Architecture and Spectroscopic Signatures, Inorg. Chem., 2012, 51(17), 9282–9296 CrossRef CAS PubMed
.
- I. Chi-Duran, J. Enriquez, C. Manquian, K. Wrighton-Araneda, W. Canon-Mancisidor, D. Venegas-Yazigi, F. Herrera and D. P. Singh, pH-Controlled Assembly of 3D and 2D Zinc-Based Metal-Organic Frameworks with Tetrazole Ligands, ACS Omega, 2018, 3(1), 801–807 CrossRef CAS PubMed
.
- R. Abazari, A. R. Mahjoub, A. M. Z. Slawin and C. L. Carpenter-Warren, Morphology- and size-controlled synthesis of a metal–organic framework under ultrasound irradiation: An efficient carrier for pH responsive release of anti-cancer drugs and their applicability for adsorption of amoxicillin from aqueous solution, Ultrason. Sonochem., 2018, 42, 594–608 CrossRef CAS PubMed
.
- Y. Lv, S. Wang, R. Zhang, D. Zhang, H. Yu and G. Lu, PH-modulated formation of uniform MOF-5 sheets, Inorg. Chem. Commun., 2018, 97, 30–33 CrossRef CAS
.
- R. Seetharaj, P. V. Vandana, P. Arya and S. Mathew, Dependence of solvents, pH, molar ratio and temperature in tuning metal organic framework architecture, Arabian J. Chem., 2019, 12(3), 295–315 CrossRef CAS
.
- X. Luo, Q. Zhou, S. Du, J. Li, L. Zhang, K. Lin, H. Li, B. Chen, T. Wu, D. Chen, M. Chang and Y. Liu, One-Dimensional Porous Hybrid Structure of Mo2C-CoP Encapsulated in N-Doped Carbon Derived from MOF: An Efficient Electrocatalyst for Hydrogen Evolution Reaction over the Entire pH Range, ACS Appl. Mater. Interfaces, 2018, 10(49), 42335–42347 CrossRef CAS PubMed
.
- S. S. Y. Chui, S. M. F. Lo, J. P. H. Charmant, A. G. Orpen and I. D. Williams, A chemically functionalizable nanoporous material [Cu-3(TMA)(2)(H2O)(3)](n), Science, 1999, 283(5405), 1148–1150 CrossRef CAS PubMed
.
- K.-S. Lin, A. K. Adhikari, C.-N. Ku, C.-L. Chiang and H. Kuo, Synthesis and characterization of porous HKUST-1 metal organic frameworks for hydrogen storage, Int. J. Hydrogen Energy, 2012, 37(18), 13865–13871 CrossRef CAS
.
- A. Rauf, J. Ye, S. Zhang, Y. Qi, G. Wang, Y. Che and G. Ning, Copper(II)-based coordination polymer nanofibers as a highly effective antibacterial material with a synergistic mechanism, Dalton Trans., 2019, 48, 17810–17817 RSC
.
- A. Kojtari, P. J. Carroll and H.-F. Ji, Metal organic framework (MOF) micro/nanopillars, CrystEngComm, 2014, 16(14), 2885–2888 RSC
.
- J. M. Garcia-Garfido, J. Enríquez, I. Chi-Durán, I. Jara, L. Vivas, F. J. Hernández, F. Herrera and D. P. Singh, Millimeter-scale zn(3-ptz)2 metal-organic framework single crystals: Self-assembly mechanism and growth kinetics, ACS Omega, 2021, 6(27), 17289–17298 CrossRef CAS PubMed
.
- R. Pech and J. Pickardt, Catena-Triaqua-Mu-[1,3,5-benzenetricarboxylato(2-)]-Copper(II), Acta Crystallogr., Sect. C: Cryst. Struct. Commun., 1988, 44(6), 992–994 CrossRef
.
- G. W. Peterson, G. W. Wagner, A. Balboa, J. Mahle, T. Sewell and C. J. Karwacki, Ammonia Vapor Removal by Cu-3(BTC)(2) and Its Characterization by MAS NMR, J. Phys. Chem. C, 2009, 113(31), 13906–13917 CrossRef CAS PubMed
.
- G. Majano, O. Martin, M. Hammes, S. Smeets, C. Baerlocher and J. Perez-Ramirez, Solvent-Mediated Reconstruction of the Metal-Organic Framework HKUST-1 (Cu-3(BTC)(2)), Adv. Funct. Mater., 2014, 24(25), 3855–3865 CrossRef CAS
.
- C. Prestipino, L. Regli, J. G. Vitillo, F. Bonino, A. Damin, C. Lamberti, A. Zecchina, P. L. Solari, K. O. Kongshaug and S. Bordiga, Local structure of framework Cu(II) in HKUST-1 metallorganic framework: Spectroscopic characterization upon activation and interaction with adsorbates, Chem. Mater., 2006, 18(5), 1337–1346 CrossRef CAS
.
- N. R. Dhumal, M. P. Singh, J. A. Anderson, J. Kiefer and H. J. Kim, Molecular Interactions of a Cu-Based Metal-Organic Framework with a Confined Imidazolium-Based Ionic Liquid: A Combined Density Functional Theory and Experimental Vibrational Spectroscopy Study, J. Phys. Chem. C, 2016, 120(6), 3295–3304 CrossRef CAS
.
- S. D. Worrall, M. A. Bissett, P. I. Hill, A. P. Rooney, S. J. Haigh, M. P. Attfield and R. A. W. Dryfe, Metal-organic framework templated electrodeposition of functional gold nanostructures, Electrochim. Acta, 2016, 222, 361–369 CrossRef CAS
.
- J. Cortes-Suarez, V. Celis-Arias, H. Beltran, I. A. Tejeda-Cruz, I. A. Ibarra, J. E. Romero-Ibarra, E. Sanchez-Gonzalez and S. Loera-Serna., Synthesis and Characterization of an SWCNT@HKUST-1 Composite: Enhancing the CO2 Adsorption Properties of HKUST-1, ACS Omega, 2019, 4(3), 5275–5282 CrossRef CAS PubMed
.
- N. Marshall, W. James, J. Fulmer, S. Crittenden, A. B. Thompson, P. A. Ward and G. T. Rowe, Polythiophene Doping of the Cu-Based Metal-Organic Framework (MOF) HKUST-1 Using Innate MOF-Initiated Oxidative Polymerization, Inorg. Chem., 2019, 58(9), 5561–5575 CrossRef CAS PubMed
.
- F. S. Gentile, M. Pannico, M. Causa, G. Mensitieri, G. D. Palma, G. Scherillo and P. Musto, Metal defects in HKUST-1 MOF revealed by vibrational spectroscopy: a combined quantum mechanical and experimental study, J. Mater. Chem., 2020, 8(21), 10796–10812 RSC
.
- C. Petit, B. Mendoza, D. O'Donnell and T. J. Bandosz, Effect of Graphite Features on the Properties of Metal-Organic Framework/Graphite Hybrid Materials Prepared Using an in Situ Process, Langmuir, 2011, 27(16), 10234–10242 CrossRef CAS PubMed
.
- M. D. Allendorf, C. A. Bauer, R. K. Bhakta and R. J. T. Houk, Luminescent metal-organic frameworks, Chem. Soc. Rev., 2009, 38(5), 1330–1352 RSC
.
- Y. Cui, Y. Yue, G. Qian and B. Chen, Luminescent Functional Metal-Organic Frameworks, Chem. Rev., 2012, 112(Supp. 2), 1126–1162 CrossRef CAS PubMed
.
- J. Heine and K. Mueller-Buschbaum, Engineering metal-based luminescence in coordination polymers and metal–organic frameworks, Chem. Soc. Rev., 2013, 42(24), 9232–9242 RSC
.
- R. Ameloot, F. Vermoortele, J. Hofkens, F. C. De Schryver, D. E. De Vos and M. B. J. Roeffaers, Three-Dimensional Visualization of Defects Formed during the Synthesis of Metal-Organic Frameworks: A Fluorescence Microscopy Study, Angew. Chem., Int. Ed., 2013, 52(1), 401–405 CrossRef CAS PubMed
.
- Z. Fang, B. Bueken, D. E. D. Vos and R. A. Fischer, Defect-Engineered Metal-Organic Frameworks, Angew. Chem., Int. Ed., 2015, 54(25), 7234–7254 CrossRef CAS PubMed
.
- M. Shoaee, M. W. Anderson and M. P. Attfield, Crystal Growth of the Nanoporous Metal-Organic Framework HKUST-1 Revealed by In Situ Atomic Force Microscopy, Angew. Chem., Int. Ed., 2008, 47(44), 8525–8528 CrossRef CAS PubMed
.
- M. Shoeaee, J. R. Agger, M. W. Anderson and M. P. Attfield, Crystal form, defects and growth of the metal organic framework HKUST-1 revealed by atomic force microscopy, CrystEngComm, 2008, 10(6), 646–648 RSC
.
- Z. Fang, J. P. Duerholt, M. Kauer, W. Zhang, C. Lochenie, B. Jee, B. Albada, N. Metzler-Nolte, A. Poeppl, B. Weber, M. Muhler, Y. Wang, R. Schmid and R. A. Fischer, Structural Complexity in Metal-Organic Frameworks: Simultaneous Modification of Open Metal Sites and Hierarchical Porosity by Systematic Doping with Defective Linkers, J. Am. Chem. Soc., 2014, 136(27), 9627–9636 CrossRef CAS PubMed
.
- K. Müller, K. Fink, L. Schöttner, M. Koenig, L. Heinke and C. Wöll, Defects as color centers: The apparent color of metal-organic frameworks containing Cu2+ -based paddle-wheel units, ACS Appl. Mater. Interfaces, 2017, 9(42), 37463–37467 CrossRef PubMed
, PMID: 28976730.
- W. Zhang, M. Kauer, P. Guo, S. Kunze, S. Cwik, M. Muhler, Y. Wang, K. Epp, G. Kieslich and R. A. Fischer, Impact of synthesis parameters on the formation of defects in hkust-1, Eur. J. Inorg. Chem., 2017, 2017(5), 925–931 CrossRef CAS
.
- S. Bordiga, L. Regli, F. Bonino, E. Groppo, C. Lamberti, B. Xiao, P. S. Wheatley, R. E. Morris and A. Zecchina, Adsorption properties of hkust-1 toward hydrogen and other small molecules monitored by ir, Phys. Chem. Chem. Phys., 2007, 9(21), 2676–2685 RSC
.
- T. Steenhaut, N. Gregoire, G. Barozzino-Consiglio, Y. Filinchuk and S. Hermans, Mechanochemical defect engineering of HKUST-1 and impact of the resulting defects on carbon dioxide sorption and catalytic cyclopropanation, RSC Adv., 2002, 10(34), 19822–19831 RSC
.
- L. Guo, J. Du, C. Li, G. He and Y. Xiao, Facile synthesis of hierarchical micro-mesoporous hkust-1 by a mixed-linker defect strategy for enhanced adsorptive removal of benzothiophene from fuel, Fuel, 2021, 300, 120955 CrossRef CAS
.
- D. F. Sanchez, J. Ihli, D. Zhang, T. Rohrbach, P. Zimmermann, J. Lee, C. N. Borca, N. Böhlen, D. Grolimund and J. A. van Bokhoven,
et al., Spatio-chemical heterogeneity of defect-engineered metal-organic framework crystals revealed by full-field tomographic X-ray absorption spectroscopy, Angew. Chem., 2021, 133(18), 10120–10127 CrossRef
.
- M. Polozij, M. Rubes, J. Cejka and P. Nachtigall, Catalysis by Dynamically Formed Defects in a Metal-Organic Framework Structure: Knoevenagel Reaction Catalyzed by Copper Benzene-1,3,5-tricarboxylate, ChemCatChem, 2014, 6(10), 2821–2824 CrossRef CAS
.
- M. Todaro, G. Buscarino, L. Sciortino, A. Alessi, F. Messina, M. Taddei, M. Ranocchiari, M. Cannas and F. M. Gelardi, Decomposition process of carboxylate mof hkust-1 unveiled at the atomic scale level, J. Phys. Chem. C, 2016, 120(23), 12879–12889 CrossRef CAS
.
- K. Müller, N. Vankova, L. Schöttner, T. Heine and L. Heinke, Dissolving uptake-hindering surface defects in metal-organic frameworks, Chem. Sci., 2019, 10, 153–160 RSC
.
- F. A. Sofi, K. Majid and O. Mehraj, The visible light driven copper based metal-organic-framework heterojunction:hkust-1@ag-ag3po4 for plasmon enhanced visible light photocatalysis, J. Alloys Compd., 2018, 737, 798–808 CrossRef CAS
.
- C. Fan, H. Dong, Y. Liang, J. Yang, G. Tang, W. Zhang and Y. Cao, Sustainable synthesis of hkust-1 and its composite by biocompatible ionic liquid for enhancing visible-light photocatalytic performance, J. Cleaner Prod., 2019, 208, 353–362 CrossRef CAS
.
- Y. Qiao, Q. Han, D. Li, H. Li, B. Wei, G. Che, W. Jiang and W. Guan, Construction of novel Ag/HKUST-1/g-C3N4 towards enhanced photocatalytic activity for the degradation of pollutants under visible light, RSC Adv., 2019, 9(71), 41591–41602 RSC
.
- N. K. Kulachenkov, D. Sun, Y. A. Mezenov, A. N. Yankin, S. Rzhevskiy, V. Dyachuk, A. Nominé, G. Medjahdi, E. A. Pidko and V. A. Milichko, Photochromic free mof-based near-infrared optical switch, Angew. Chem., Int. Ed., 2020, 59(36), 15522–15526 CrossRef CAS PubMed
.
Footnote |
† Electronic supplementary information (ESI) available. CCDC 2069999 and 2070000. For ESI and crystallographic data in CIF or other electronic format see DOI: 10.1039/d1ma00866h |
|
This journal is © The Royal Society of Chemistry 2022 |
Click here to see how this site uses Cookies. View our privacy policy here.