DOI:
10.1039/D2MA00170E
(Paper)
Mater. Adv., 2022,
3, 3554-3561
A new method of synthesis of Sb2Se3/rGO as a high-rate and low-temperature anode for sodium–ion batteries†
Received
15th February 2022
, Accepted 2nd March 2022
First published on 2nd March 2022
Abstract
Sodium–ion batteries (SIBs) are expected to replace lithium–ion batteries with low cost and high safety to become a new generation of energy storage equipment. Here, a novel precipitation transformation method is used to synthesize Sb2Se3 wrapped by reduced graphene oxide (rGO) as an anode for SIBs. These Sb2Se3 nanoparticles with a large surface area and multiple active sites can accelerate sodium ion diffusion and storage, resulting in high capacity and excellent rate performance. At the same time, rGO can alleviate the particle agglomeration of Sb2Se3 caused by volume expansion and increase the conductivity of the compound to improve charge transport. This synthesis method enables nanoscale and tight integration with rGO, synchronously satisfying the fast transport of ions and electrons, which provides conditions for the development of low-temperature performance. More importantly, Sb2Se3/rGO exhibits a capacity of 260 mA h g−1 at a current density of 20 A g−1 at room temperature. Even at −15 °C, the capacity retention remains at 38% at a current density of 2 A g−1. Therefore, this precipitation transformation method can provide new ideas for synthesizing other compounds.
Introduction
Lithium-ion batteries as clean energy storage equipment have received extensive attention.1–3 However, the lack of lithium resources limits the application of lithium-ion batteries in large-scale energy storage equipment. Sodium–ion batteries (SIBs) are promising energy cells because of the similarity of sodium ions to lithium ions and the advantages of rich and inexpensive sodium resources. The application of SIBs is still limited by low energy density/power density, poor low-temperature performance and so on.4–7 Due to geographical location and climate, the temperature varies widely among regions. A battery may need to work in extreme environments, so exploring low-temperature battery materials is essential.8,9 For the anode materials, the kinetics of sodium ions insertion is sluggish at low temperature (LT). There is a problem of asymmetry in charging and discharging, and the electrode material is heavily polarized at a high current density.10,11 Therefore, it is urgent to improve the electrochemical performance at LT.
Compared with the low-capacity de-intercalation mechanism of carbon materials and the alloy-mechanism materials with large volume expansion, transformation mechanism materials can exhibit high capacity with relatively small volume expansion.12,13 Common anode materials include oxides and sulfur (selenides). Sulfur (selenides) has higher reaction reversibilities and higher Coulombic efficiencies compared with oxides. Sb2Se3 can also work as an anode material with a theoretical specific capacity of 670 mA h g−1, which is unmatched by carbon materials.14–17 Moreover, both the conversion and alloy reactions are involved in the sodiation/desodiation process of Sb2Se3.18,19 The product of the conversion reaction can be used as a buffer material for the alloy reaction to reduce the stress during particle pulverization. LT batteries require higher ionic and electronic conductivity of electrode materials. On the one hand, a composite with carbon material can improve the conductivity of the material and realize the rapid conduction of electrons.20,21 On the other hand, nanoscale materials can shorten the ion transport path and achieve fast conduction of sodium ions.15,22–24
However, based on the crystal growth law of antimony selenide, it is easy to grow large rods and difficult to synthesize nanoparticles.24,25 Ou et al. synthesized a reduced graphene oxide (rGO)-overcoated Sb2Se3 nanorod anode for Na+ battery, with a linear length of ≈600 nm and a diameter of ≈50 nm. After combining with rGO, the cycling performance of Sb2Se3/rGO is greatly improved, but due to the large Sb2Se3 nanorods, the rate performance is not satisfactory. At a current density of 2 A g−1, the capacity retention was only 34% compared to that at 0.1 A g−1.15 Then, Fang et al. developed an ion-exchange method to synthesize Sb2Se3 microclips based on a microbelts template. Sb2Se3@PPy microclips exhibited improved cycling and rate performance. However, this anisotropic hollow structure is easily collapsed during cycling and agglomerates, thus hindering ionic conduction, and does not exhibit good electrochemical performance.22 Therefore, it is important to use a new method to synthesize carbon–Sb2Se3 composite nanoparticles, to achieve fast conduction of ions and electrons simultaneously at LT.26–30
Here, a new precipitation transformation method is used to synthesize Sb2Se3 wrapped by rGO with controllable shape and size. The size of Sb2Se3 is only about 10 nm; such small nanoparticles with a large surface area can accelerate the reaction kinetics of sodium ion diffusion and storage, leading to high capacity and good rate performance. The addition of graphene can increase the conductivity and alleviate the particle agglomeration caused by volume expansion of Sb2Se3 in cycling. This structure design can realize fast conduction of electrons and ions, which is conducive to electrochemical performance.
Experimental section
Material synthesis
Sb2Se3/rGO was fabricated by a precipitation transformation method. Firstly, SnSe nanosheets were synthesized at room temperature. 79 mg Se and 20 g NaOH were dissolved in 50 ml deionized water. Secondly, 0.45 g SnCl2·2H2O and 10 g citric acid were dissolved in another 50 ml deionized water. The latter solution was added dropwise into the former under stirring until the solution turned black. SnSe nanosheets were collected by centrifugation, washing, and drying at 60 °C in a vacuum overnight. Next, graphene was obtained from graphite (Sinochem) using a modified Hummers’ method,31 and then 30 mg graphene and 0.1 g SnSe nanosheets were dissolved in 30 ml deionized water. 0.114 g SbCl3 was dissolved in 10 ml alcohol and then added to the former solution under stirring. The solution was transferred into a Teflon-lined stainless autoclave and heated at 120 °C for 12 h. After centrifuging, washing, and drying, the powders were annealed at 400 °C for two hours in Ar/H2 (95
:
5).
Structural characterization
X-Ray diffraction (XRD) patterns were acquired with an X-ray diffractometer (Bruker D8 Adv, Germany). X-ray photoelectron spectroscopy (XPS) data were obtained with an X-ray photoelectron spectrometer (ES CALAB250, USA). Transmission electron microscopy (TEM), high-resolution TEM (HRTEM), energy-dispersive X-ray spectrometry (EDS) as well as elemental mapping were conducted with transmission electron microscopes (JEOL GEM1011 or JEOL GEM-2100F, Japan). Scanning electron microscopy (SEM) images were obtained with a field-emission scanning electron microscope (Zeiss SUPRA55, Germany). Thermogravimetric analysis (TGA) was conducted with a Mettler Toledo TGA/SDTA851 thermal analyzer from 40 °C to 700 °C in air. N2 sorption isotherms were performed with a Builder ASAP 2020 HD88 physisorption analyzer at 77 K.
Electrochemical measurements
The sodium-storage performances of the samples were tested with CR2032 coin cells. The working electrode was made of 70 wt% of the active materials, 20 wt% acetylene black, and 10 wt% of sodium alginate. The blend was milled for 30 min with droplets of deionized water to get a slurry. The slurry was spread on a clean copper foil and dried overnight in a vacuum at 60 °C. The loading mass of the active material was about 1 mg cm−2. Coin cells were assembled using an Ar-filled glovebox (Mikrouna, Super 122017501900). 1.0 M NaClO4 in ethylene carbonate and dimethyl carbonate (volume ratio of 1
:
1) containing 5% fluoroethylene carbonate was the electrolyte, Whatman GF/F glass microfibers were the separator, and Na foil was the counter and reference electrodes. Cyclic voltammetry (CV) curves were obtained using an electrochemical workstation (LK200A, China) at room-temperature. Galvanostatic discharge/charge profiles were measured on Land battery cyclers (Land CT2001A, China) between 0.01 and 2 V for SnSe nanosheets and 0.01 and 2.5 V for Sb2Se3/rGO at different temperatures. Electrochemical impedance spectra (EIS) were collected with an electrochemical workstation (Autolab PGSTAT 302N) in the frequency range of 100 kHz to 0.01 Hz.
Results and discussion
Sb2Se3/rGO was synthesized via a precipitation transformation method, as illustrated in Scheme 1. First, pure SnSe nanosheets (Fig. S1 and S2, ESI†) were synthesized in a simple room-temperature method.32 The SnSe nanosheets showed a size distribution with diameters of ∼70 nm (Fig. S3, ESI†). Then Sb2Se3/rGO was made by adding SbCl3 and graphene via a solvothermal reaction at 120 °C. The conversion mechanism is that the concentration of SbCl3 is very large, and it reacts with Se2− dissociated from SnSe. With the consumption of Se2−, the reaction to form Sb2Se3 is promoted. As long as the content of Sn in the final component is not high, less than the Ksp of SnSe,15 it exists in the form of ions and can be removed after washing steps. Finally, the composite was calcined at 400 °C in Ar/H2, leading to the reduction of graphene to rGO.
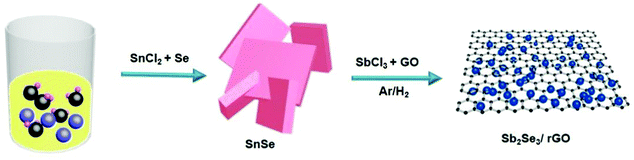 |
| Scheme 1 The preparation process of Sb2Se3/rGO. | |
The physical and chemical properties of the material are shown in Fig. 1. Fig. 1a shows the XRD pattern of Sb2Se3/rGO. It is noted that all the reflections come from the orthorhombic Sb2Se3 phase (JCPDS card no. 72-1184).15 The peak at 26° originated from rGO, indicating the presence of rGO in the composite.33,34 Further evidence comes from XPS spectra of Sb2Se3/rGO. Fig. 1b shows two peaks at 55.18 and 56.2 eV, which can be attributed to Se 3d3/2 and Se 3d5/2, respectively, indicating the presence of Se2− in Sb2Se3.35 As shown in Fig. 1c, the two peaks at 530.9 and 540.2 eV are assigned to Sb 3d3/2 and Sb 3d5/2 of Sb3+ in Sb2Se3. Another peak at 533.2 eV corresponds to O 1s, which can be attributed to the lesser oxidation of Sb2Se3 nanoparticles.23 There are four peaks located at 284.6, 285.2, 286.6, and 289.2 eV from the high-resolution XPS spectrum of C 1s, owing to SP2− bonded C–C, SP3- bonded C–C, C
O and O
C–O, respectively (Fig. 1d).28,36 The content of Sb2Se3 in the composite was examined by TGA. Fig. 1e shows the weight gain before 400 °C, assigned to the oxidation of Sb2Se3 to Sb2O4 and SeO2. Next, weight loss is associated with the sublimation of SeO2 and the oxidation of rGO (Fig. S4, ESI†). Through calculation, the content of Sb2Se3 in the composite is about 81 wt%. This result is in good agreement with the EDS spectrum (Fig. S5, ESI†). As shown in Fig. 1f, the specific surface area of Sb2Se3/rGO is measured by nitrogen adsorption–desorption isotherm, with a specific surface area of 58.3 m g−2 and an average pore size of ∼25 nm, created by particle stacking. Although the large specific surface area reduces the initial Coulomb efficiency, it dramatically increases the migration speed of sodium ions.
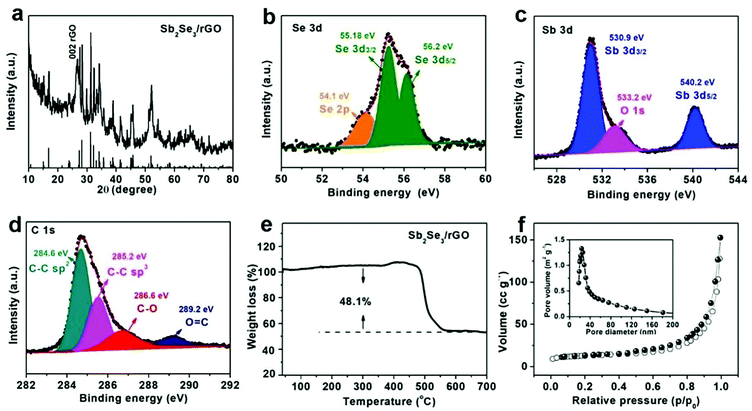 |
| Fig. 1 Structural characterization. (a) XRD pattern. XPS spectra of (b) Se-3d; (c) Sb-3d; (d) C-1s of Sb2Se3/rGO. (e) TGA curve. (f) N2 sorption isotherms and pore size distribution of Sb2Se3/rGO. | |
The morphology characterization is shown in Fig. 2. Fig. 2a and b show SEM images of nanoparticles that are uniformly distributed on the surface of rGO. As shown in Fig. 2c and d, the size of Sb2Se3 nanoparticles is ∼10 nm, and the tiny size could lead to large surface area, which is well matched with the better electrochemical performance. HRTEM image (Fig. 2e) shows clear lattice fringes with a spacing ∼0.316 nm that could be attributed to (211) planes of Sb2Se3, which is in agreement with the XRD results in Fig. 1a. EDS element mapping can further reveal that both Sb and Se are uniformly distributed throughout the rGO, as illustrated in Fig. 2f. The size of Sb2Se3 is controllable through the adjustment of concentration, reaction time and temperature. The different size of Sb2Se3 particles means that the reaction kinetics is inconsistent, which in turn affects the electrochemical performance of the material. This will be described in the electrochemical performance section.
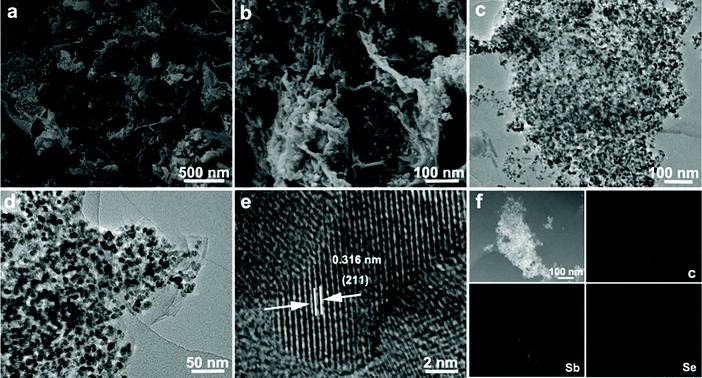 |
| Fig. 2 Morphology characterization. (a and b) SEM images; (c and d) TEM images; (e) HRTEM image; (f) elemental mapping of Sb2Se3/rGO. | |
The reaction mechanism plays a decisive role in electrochemical performance. Hence, it is vital to explore the reaction mechanism. Sb2Se3/rGO is evaluated as an anode material in a half-cell of SIB. Fig. 3a shows CV curves of Sb2Se3/rGO during the first three cycles, where the broad cathodic peak centered at 0.98 V is attributed to the intercalation of Na ions into Sb2Se3 and the formation of Sb along with Na2Se.23 The peak over 0.2–0.7 V is correlated with the alloying reaction between Sb and Na, and the decomposition of electrolyte to form a solid-state interphase (SEI) film.37–42 The anodic peaks located at 0.75 V and 1.25 V are attributed to the dealloying reaction of Na3Sb to form Sb, followed by the conversion reaction between Sb and Na2Se.43,44 The broad peak around 1.8 V could correspond to the Na ions extracted from NaxSb2Se3 to form Sb2Se3.45,46 The following two cycles are matched well, indicating the excellent reversibility of Sb2Se3/rGO during alloying and conversion reactions. To clarify the reactions during sodiation/desodiation processes, ex situ HRTEM images of Sb2Se3/rGO were obtained. The ex situ HRTEM images were collected at different discharge/charge voltages in the first cycle. Fig. 3b shows an image of Sb2Se3/rGO discharged to 0.7 V. In this image, the lattice fringes of 0.311 nm and 0.241 nm can be attributed to (012) and (220) planes of Sb and Na2Se, respectively. With the discharge voltage decreased to 0.01 V, the lattice fringes of Na3Sb appeared (Fig. 3c), indicating the alloying reaction between Na ions and Sb. When fully charged to 2.5 V, the lattice fringes of the sample shown in Fig. 3d suggest that Sb2Se3 has formed, indicating the reversibility of the Sb2Se3/rGO anode for Na ion storage. All the results agree well with the CV curves in Fig. 3a.
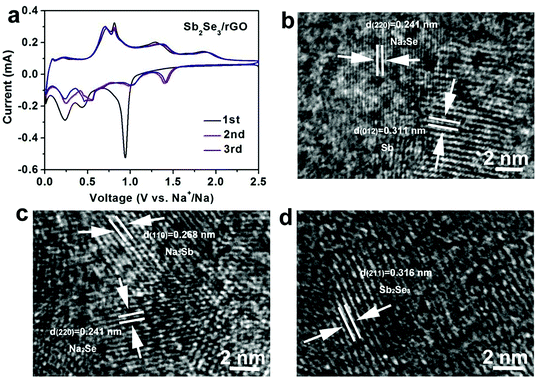 |
| Fig. 3 Electrochemical mechanism analysis. (a) Cyclic voltammograms of Sb2Se3/rGO. HRTEM images of Sb2Se3/rGO: (b) discharged to 0.7 V; (c) fully discharged to 0.01 V; (d) fully charged to 2.5 V. | |
The electrochemical properties of precursor (SnSe) and product (Sb2Se3/rGO) are shown in Fig. 4. SnSe nanosheets are a precursor material, and the cycle characteristics (Fig. S6, ESI†) and rate performances (Fig. S7, ESI†) were tested. SnSe nanosheets exhibit a capacity of 334 mA h g−1 at a current density of 0.5 A g−1 after 100 cycles. As a material with a nanosheet structure, the rate performance is good because of the fast reaction kinetics. The replacement of Sn by Sb is accompanied by the formation of nanoparticles, the capacity and kinetics being further improved. At the same time, rGO materials are introduced to increase conductivity and cycling stability. Through the structure design, high-rate and excellent low-temperature characteristics can be guaranteed. In order to obtain better electrochemical properties, many conditions have been tested, such as the amount of SbCl3 added during the reaction (Fig. S8, ESI†), the temperature and time of the hydrothermal reaction (Fig. S9 and S10, ESI†), the calcination temperature in Ar/H2 (Fig. S11, ESI†), and the cut-off voltage during charge/discharge (Fig. S12, ESI†). After optimization, Sb2Se3/rGO with the best electrochemical performance is selected. All of the following examinations are based on optimal conditions.
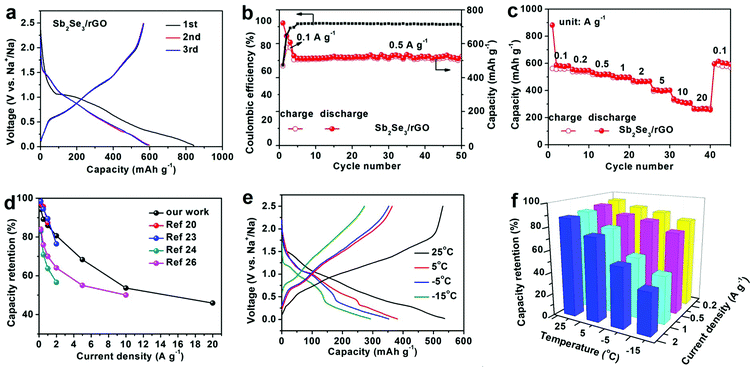 |
| Fig. 4 Electrochemical performances. (a) Discharge/charge curves of Sb2Se3/rGO at a current density of 0.1 A g−1. (b) Cycling performance and (c) rate performance of Sb2Se3/rGO. (d) Comparison of results of reported works with ours in terms of capacity retention. (e) Discharge/charge curves of Sb2Se3/rGO at different temperatures. (f) The capacity retentions of Sb2Se3/rGO along with temperature variations between 25 and −15 °C at a various current densities of 0.2, 0.5, 1, and 2 A g−1. | |
Fig. 4a shows the galvanostatic charge/discharge curves for the first three cycles. The cathodic/anodic peaks in CV curves match well with the charge/discharge plateaus. As we can see from the first-cycle charge/discharge curve, the initial Coulombic efficiency is ∼67.3%. The capacity loss comes from the decomposition of electrolytes, the formation of SEI film, and so on.47,48 In the following cycles, the capacity is almost unchanged, indicating the good reversibility of the compound. Fig. 4b shows the cycling performance of Sb2Se3/rGO within 0.01–2.5 V at a current density of 0.5 A g−1. It indicates that the composite exhibits a reversible capacity at 511 mAh g−1 after 50 cycles. As shown in Fig. S13 (ESI†), the capacity of rGO is about 75 mA h g−1 at a current density of 0.5 A g−1. The theoretical specific capacity of Sb2Se3/rGO composite is 75 mA h g−1*19% + 670 mAh g−1 × 81% = 557 mA h g−1, which is consistent with the results in Fig. 4b. The excellent rate performance is shown in Fig. 4c. The capacity of Sb2Se3/rGO is 580.6 mA h g−1 at 0.1 A g−1, 547 mA h g−1 at 0.2 A g−1, 517.5 mA h g−1 at 0.5 A g−1, 498.8 mA h g−1 at 1 A g−1, 467.9 mA h g−1 at 2 A g−1, 396.8 mA h g−1 at 5 A g−1, and 311 mA h g−1 at 10 A g−1. Even at a high current density of 20 A g−1, it still has a capacity of 266.5 mA h g−1 with a capacity retention of 46% related to the discharge capacity at 0.1 A g−1. This is in good agreement with the large surface area and multiple active sites of small Sb2Se3 nanoparticles, which accelerate Na ion diffusion kinetics.49 It is found that the rate performance of Sb2Se3/rGO is superior to that of previously reported Sb2Se3 in SIBs (Fig. 4d), including polypyrrole-coated Sb2Se3 microclips (486 mA h g−1 at 2 A g−1),20 Sb2Se3 nanowires (247 mA h g−1 at 2 A g−1),23 capsular carbon shell-encapsulated Sb2Se3 (398.7 mA h g−1 at 2 A g−1),24 and carbon nanofibers with embedded Sb2Se3 nanoparticles (250 mA h g−1 at 10 A g−1).26 To understand the excellent electrochemical performances of Sb2Se3/rGO in SIBs, EIS was measured at open circuit potential (OCP) and 2.5 V after five cycles (Fig. S13, ESI†). It is fitted by an equivalent circuit (Table S1, ESI†). Sb2Se3/rGO presents a smaller charge-transfer resistance (Rct) after five cycles. The result is associated with the faster interface kinetics, resulting in excellent rate performance.50 However, Rct after 100 cycles is greater than after 5 cycles, because of the continuous thickening of the SEI film and the agglomeration caused by the structural destruction of Sb2Se3, causing a slowed ion conduction. In addition to the excellent rate performance, the composite also displays outstanding LT performance. Fig. 4e shows the discharge curves tested under various temperatures from 25 °C to −15 °C at a current density of 0.1 A g−1. With a drop in temperature, the capacity gradually decreases. This can be associated with the difficult sodiation and serious polarization of the electrode at LT.51 The discharge curves also show an increase in polarization voltage. The main reason is the slow desolvation of Na+ ions and the slow transport of Na+ ions in the electrode material. The LT performance of SnSe was also tested (Fig. S14, ESI†). Fig. 4f shows the capacity retentions at different discharge current densities (0.1, 0.2, 0.5, 1, and 2 A g−1) and testing temperatures (from 25 °C to −15 °C). The capacity retention at −15 °C compared to that at 25 °C is ∼77.5% at 0.2 A g−1 and ∼43% at 2 A g−1. This result is better than that of SnSe tested at the same condition (Fig. S15, ESI†), proving the importance of our structural design.
Conclusions
In summary, Sb2Se3/rGO is synthesized by the precipitation transformation method with controllable shape and size. This composite exhibits an outstanding rate performance owing to small Sb2Se3 nanoparticles with an increase of specific surface area and increase of active sites. The diffusion and storage of sodium ions become more rapid, resulting in good rate performance. What is more, rGO can alleviate the particle agglomeration caused by volume expansion of Sb2Se3 and increase the conductivity of the compound. This synthesis strategy can tightly bind Sb2Se3 nanoparticles to rGO, and achieve fast conduction of ions and electrons simultaneously. As for the rate capability, the composite exhibits a reversible capacity of 266 mAh g−1 at 20 A g−1. Even at −15 °C, the capacity retention remains at 38% at a current density of 2 A g−1. All those data indicate that this composite structure has promising potential in low-temperature SIBs. In the future, the precipitation transformation conversion method could be widely used in the synthesis of other composite nanomaterials.
Author contributions
Lulu Hu: methodology, formal analysis, investigation, writing – original draft, resources. Jun Pan: conceptualization, investigation, data curation, writing – original draft, writing – review & editing, supervision. Pei Zhao: draft curation, supervision. Gejun Shi: data curation, supervision. Baofeng Wang: investigation, funding acquisition, resources, writing – review & editing. Fuqiang Huang: methodology, writing – review & editing, supervision, project administration, funding acquisition.
Conflicts of interest
The authors declare that they have no known competing financial interests or personal relationships that could have appeared to influence the work reported in this paper.
Acknowledgements
This work is financially supported by the National Natural Science Foundation of China (No. 22075173), the Science and Technology Commission of Shanghai Municipality (No. 19DZ2271100 and 21010501100), the Key Research Program of Frontier Science of the Chinese Academy of Sciences (Grant No. QYZDJ-SSW-JSC013), and the Super Post-Doctoral Fellow Program of Shanghai (E01SCB17).
References
- J. Xie and Y. C. Lu, A retrospective on lithium-ion batteries, Nat. Commun., 2020, 11, 2499 CrossRef CAS PubMed.
- Y. J. Fan, D. Y. Luan, S. Y. Gao and X. W. Lou, Rational design and engineering of one-dimensional hollow nanostructures for efficient electrochemical energy storage, Angew. Chem., Int. Ed., 2021, 60, 20102–20118 CrossRef PubMed.
- F. X. Wu, J. Maier and Y. Yu, Guidelines and trends for next-generation rechargeable lithium and lithium-ion batteries, Chem. Soc. Rev., 2020, 49, 1569–1614 RSC.
- P. K. Nayak, L. Yang, W. Brehm and P. Adelhelm, From Lithium-Ion to Sodium-Ion Batteries: Advantages, Challenges, and Surprises, Angew. Chem., Int. Ed., 2018, 57, 102–120 CrossRef CAS PubMed.
- J. Y. Hwang, S. T. Myung and Y. K. Sun, Sodium-ion batteries: present and future, Chem. Soc. Rev., 2017, 46, 3529–3614 RSC.
- D. R. Deng, X. Y. Cui, Q. H. Wu, M. S. Zheng and Q. F. Dong,
In situ synthesis TiO2 nanosheets@rGO for ultrafast sodium ion storage at both room and low temperatures, J. Alloys Compd., 2020, 835, 155413 CrossRef CAS.
- A. L. Zhao, Y. J. Fan, X. P. Ai, H. X. Yang and Y. L. Cao, Mixed polyanion cathode materials: Toward stable and high-energy sodium-ion batteries, J. Energy Chem., 2021, 60, 635–648 CrossRef.
- Y. Y. Wang, B. H. Hou, J. Z. Guo, Q. L. Ning, W. L. Pang, J. Wang, C. L. Lü and X. L. Wu, An ultralong lifespan and low-temperature workable sodium-ion full battery for stationary energy storage, Adv. Energy Mater., 2018, 8, 1703252 CrossRef.
- H. L. Zhu, F. Shen, W. Luo, S. Z. Zhu, M. H. Zhao, B. Natarajan, J. Q. Dai, L. H. Zhou, X. L. Ji, R. S. Yassar, T. Li and L. B. Hu, Low temperature carbonization of cellulose nanocrystals for high performance carbon anode of sodium-ion batteries, Nano Energy, 2017, 33, 37–44 CrossRef CAS.
- J. Z. Guo, P. F. Wang, X. L. Wu, X. H. Zhang, Q. Yan, H. Chen, J. P. Zhang and Y. G. Guo, High-energy/power and low-temperature cathode for sodium-Ion batteries: in situ XRD study and superior full-cell performance, Adv. Mater., 2017, 29, 1701968 CrossRef PubMed.
- B. H. Hou, Y. Y. Wang, D. S. Liu, Z. Y. Gu, X. Feng, H. Fan, T. Zhang, C. Lü and X. L. Wu, N-doped carbon-coated Ni1.8Co1.2Se4 nanoaggregates encapsulated in N-doped carbon nanoboxes as advanced anode with outstanding high-rate and low-temperature performance for sodium-ion half/full batteries, Adv. Funct. Mater., 2018, 28, 1805444 CrossRef.
- L. C. Wang, J. Swiatowska, S. R. Dai, M. L. Cao, Z. C. Zhong, Y. Shen and M. K. Wang, Promises and challenges of alloy-type and conversion-type anode materials for sodium-ion batteries, Mater. Today Energy, 2019, 11, 46–60 CrossRef CAS.
- Y. J. Fang, D. Y. Luan and X. W. Lou, Recent advances on mixed metal sulfides for advanced sodium-ion batteries, Adv. Mater., 2020, 32, 2002976 CrossRef CAS PubMed.
- Z. Yang, J. Y. Sun, Y. Z. Ni, Z. H. Zhao, J. M. Bao and S. Chen, Facile synthesis and in situ transmission electron microscopy investigation of a highly stable Sb2Te3/C nanocomposite for sodium–ion batteries, Energy Storage Mater., 2017, 9, 214–220 CrossRef.
- X. Ou, C. H. Yang, X. H. Xiong, F. H. Zheng, Q. C. Pan, C. Jin, M. L. Liu and K. Huang, A new rGO-overcoated Sb2Se3 nanorods anode for Na+ battery: in situ X-Ray diffraction study on a live sodiation/desodiation process, Adv. Funct. Mater., 2017, 27, 1606242 CrossRef.
- J. Ding, H. L. Wang, Z. Li, A. Kohandehghan, K. Cui, Z. W. Xu, B. Zahiri, X. H. Tan, E. M. Lotfabad, B. C. Olsen and D. Mitlin, Carbon nanosheet frameworks derived from peat moss as high performance sodium ion battery anodes, ACS Nano, 2013, 7, 11004–11015 CrossRef CAS PubMed.
- G. H. Chen, J. Zhou, J. Zuo and Q. Yang, Organometallically anisotropic growth of ultralong Sb2Se3 nanowires with highly enhanced photothermal response, ACS Appl. Mater. Interfaces, 2016, 8, 2819–2825 CrossRef CAS PubMed.
- Y. Wu, W. Luo, P. Gao, C. Y. Zhu, X. B. Hu, K. Qu, J. Chen, Y. Q. Wang, L. T. Sun, L. Q. Mai and F. Xu, Unveiling the microscopic origin of asymmetric phase transformations in (de)sodiated Sb2Se3 with in situ transmission electron microscopy, Nano Energy, 2020, 77, 105299 CrossRef CAS.
- L. Guo, L. Y. Cao, J. F. Huang, J. Y. Li and S. Y. Chen, Carbon capsule confined Sb2Se3 for fast Na+ extraction in sodium-ion batteries, Sustain, Energy Fuels, 2020, 4, 797–808 CAS.
- Z. J. Cao, X. B. Ma, W. H. Dong and H. L. Wang, FeS@tubular mesoporous carbon as high capacity and long cycle life anode materials for lithium- and sodium-ions batteries, J. Alloys Compd., 2019, 786, 523–529 CrossRef CAS.
- P. Ge, X. Y. Cao, H. S. Hou, S. J. Li and X. B. Ji, Rodlike Sb2Se3 wrapped with carbon: the eploring of electrochemical properties in sodium-ion batteries, ACS Appl. Mater. Interfaces, 2017, 9, 34979–34989 CrossRef CAS PubMed.
- Y. J. Fang, X. Y. Yu and X. W. Lou, Formation of polypyrrole-coated Sb2Se3 microclips with enhanced sodium-storage properties, Angew. Chem., Int. Ed., 2018, 57, 9859–9863 CrossRef CAS PubMed.
- Q. Q. Li, P. S. Du, Y. F. Yuan, W. T. Yao, Z. T. Ma, B. K. Guo, Y. C. Lyu, P. Wang, H. T. Wang, A. M. Nie, R. Shahbazian-Yassar and J. Lu, Real-time TEM study of nanopore evolution in battery materials and their suppression for enhanced cycling performance, Nano Lett., 2019, 19, 3074–3082 CrossRef CAS PubMed.
- L. Guo, L. Y. Cao, J. F. Huang, Y. Wang, W. B. Li, H. Qi, S. Y. Chen and J. Y. Li, Design of an ultra-stable Sb2Se3 anode with excellent Na storage performance, J. Alloys Compd., 2019, 810, 151930 CrossRef CAS.
- Y. J. Fang, X. Y. Yu and X. W. Lou, Formation of polypyrrole-coated Sb2Se3 microclips with enhanced sodium-storage properties, Angew. Chem., Int. Ed., 2018, 57, 9859–9863 CrossRef CAS PubMed.
- L. S. Xia, Z. D. Yang, B. Tang, F. Li, J. P. Wei and Z. Zhou, Carbon nanofibers with embedded Sb2Se3 nanoparticles as highly reversible anodes for Na-ion batteries., Small, 2021, 17, 2006016 CrossRef CAS PubMed.
- F. E. Niu, J. Yang, N. N. Wang, D. P. Zhang, W. L. Fan, J. Yang and Y. T. Qian, MoSe2-covered N,P-doped carbon nanosheets as a long-life and high-rate anode material for sodium-ion batteries, Adv. Funct. Mater., 2017, 27, 1700522 CrossRef.
- R. F. Nie, J. J. Shi, W. C. Du, W. S. Ning, Z. Y. Hou and F. S. Xiao, A sandwich N-doped graphene/Co3O4 hybrid: an efficient catalyst for selective oxidation of olefins and alcohols, J. Mater. Chem. A, 2013, 1, 9037–9045 RSC.
- W. J. Zhao, M. J. Li, Y. B. Qi, Y. J. Tao, Z. P. Shi, Y. J. Liu and J. P. Cheng, Ultrasound sonochemical synthesis of amorphous Sb2S3-graphene composites for sodium-ion batteries, J. Colloid Interface Sci., 2021, 586, 404–411 CrossRef CAS PubMed.
- H. Q. Wang, L. Gou, W. F. Jing, D. An, Y. Li, M. Wang, N. Li, S. L. Hu and Y. B. He, Highly microporous SbPO4/BCx hybrid anodes for sodium-ion batteries, Mater. Adv., 2020, 1, 206–214 RSC.
- Y. Wen, K. He, Y. J. Zhu, F. D. Han, Y. H. Xu, I. Matsuda, Y. Ishii, J. Cumings and C. S. Wang, Expanded graphite as superior anode for sodium-ion batteries, Nat. Commun., 2014, 5, 4033 CrossRef CAS PubMed.
- S. Yuan, Y. H. Zhu, W. Li, S. Wang, D. Xu, L. Li, Y. Zhang and X. B. Zhang, Surfactant-free aqueous synthesis of pure single-crystalline SnSe nanosheet clusters as anode for high energy-and power-density sodium-ion batteries, Adv. Mater., 2017, 29, 1602469 CrossRef PubMed.
- H. T. Yuan, X. G. Liu, F. Afshinmanesh, W. Li, G. Xu, J. Sun, B. Lian, A. G. Curto, G. J. Ye, Y. Hikita, Z. X. Shen, S. C. Zhang, X. H. Chen, M. Brongersma, H. Y. Hwang and Y. Cui, Polarization-sensitive broadband photodetector using a black phosphorus vertical p–n junction, Nat. Nanotechnol., 2015, 10, 707–713 CrossRef CAS PubMed.
- M. Patel, H. Haroon, A. Kumar, J. Ahmad, G. A. Bhat, S. Lone, D. Putthusseri, K. Majid and M. Wahid, High Na+ mobility in rGO wrapped high aspect ratio 1D SbSe nano structure renders better electrochemical Na+ battery performance, ChemPhysChem, 2020, 21, 814–820 CrossRef CAS PubMed.
- S. L. Xin, Z. Q. Liu, L. Ma, Y. Sun, C. H. Xiao, F. Li and Y. P. Du, Visualization of the electrocatalytic activity of three-dimensional MoSe2@reduced graphene oxide hybrid nanostructures for oxygen reduction reaction, Nano Res., 2016, 9, 3795–3811 CrossRef CAS.
- M. Wang, Z. Z. Yang, W. H. Li, L. Gu and Y. Yu, Superior sodium storage in 3D interconnected nitrogen and oxygen dual-doped carbon network, Small, 2016, 12, 2559–2566 CrossRef CAS PubMed.
- J. Pan, N. N. Wang, Y. L. Zhou, X. F. Yang, W. Y. Zhou, Y. T. Qian and J. Yang, Simple synthesis of a porous Sb/Sb2O3 nanocomposite for a high-capacity anode material in Na-ion batteries, Nano Res., 2017, 10, 1794–1803 CrossRef CAS.
- R. Kumar, P. Lu, X. Xiao, Z. Huang and B. W. Sheldon, Strain-induced lithium losses in the solid electrolyte interphase on silicon electrodes, ACS Appl. Mater. Interfaces, 2017, 9, 28406–28417 CrossRef CAS PubMed.
- S. Bhattacharya, A. R. Riahi and A. T. Alpas, Electrochemical cycling behaviour of lithium carbonate (Li2CO3) pre-treated graphite anodes-SEI formation and graphite damage mechanisms, Carbon, 2014, 77, 99–112 CrossRef CAS.
- L. S. Xia, Z. D. Yang, B. Tang, F. Li, J. P. Wei and Z. Zhou, Carbon nanofibers with embedded Sb2Se3 nanoparticles as highly reversible anodes for Na-ion batteries, Small, 2021, 17, 2006016 CrossRef CAS PubMed.
- S. Sarkar and S. C. Peter, An overview on Sb-based intermetallics and alloys for sodium-ion batteries: trends, challenges and future prospects from material synthesis to battery performance, J. Mater. Chem. A, 2021, 9, 5164–5196 RSC.
- X. L. Qiu, X. L. Wang, Y. X. He, J. Y. Liang, K. Liang, B. L. Tardy, J. J. Richardon, M. Hu, H. Wu, Y. Zhang, O. J. Rojas, I. Manners and J. L. Guo, Superstructured mesocrystals through multiple inherent molecular interactions for highly reversible sodium ion batteries, Sci. Adv., 2021, 7, eabh3482 CrossRef CAS PubMed.
- D. K. Yu and C. M. Park, Sb-based intermetallics and nanocomposites as stable and fast Na-ion battery anodes, Chem. Eng. J., 2021, 409, 127380 CrossRef CAS.
- J. Pan, S. L. Chen, Q. Fu, Y. W. Sun, Y. C. Zhang, N. Lin, P. Gao, J. Yang and Y. Y. Qian, Layered-structure SbPO4/reduced graphene oxide: a new advanced anode material for sodium ion batteries, ACS Nano, 2018, 12, 12869–12878 CrossRef CAS PubMed.
- Y. Y. Lu, N. Zhang, S. Jiang, Y. D. Zhang, M. Zhou, Z. L. Tao, L. A. Archer and J. Chen, High-capacity and ultrafast Na-ion storage of a self-supported 3D porous antimony persulfide-graphene foam architecture, Nano Lett., 2017, 17, 3668–3674 CrossRef CAS PubMed.
- J. Lin, L. X. Yao, C. Y. Zhang, H. R. Ding, Y. H. Wu, S. Li, J. J. Han, G. H. Yue and D. L. Peng, Construction of Sb2S3@SnS@C tubular heterostructures as high-performance anode materials for sodium-ion batteries, ACS Sustainable Chem. Eng., 2021, 9, 11280–11289 CrossRef CAS.
- M. J. Hu, Y. Z. Jiang, W. P. Sun, H. T. Wang, C. H. Jin and M. Yan, Reversible conversion-alloying of Sb2O3 as a high-capacity, high-rate, and durable anode for sodium ion batteries, ACS Appl. Mater. Interfaces, 2014, 6, 19449–19455 CrossRef CAS PubMed.
- Y. H. Du, Y. F. Zhang, L. Li, N. Wang and Y. J. Chai, Nano SnO2 and Sb2O3 combined with CNTs as a high-capacity lithium storage material, Appl. Surf. Sci., 2021, 543, 148870 CrossRef CAS.
- L. Guo, L. Y. Cao, J. F. Huang, J. Y. Li, K. Kajiyoshi, J. J. He and H. Qi, Guiding fabrication of continuous carbon-confined Sb2Se3 nanoparticle structure for durable potassium-storage performance, ACS Appl. Energy Mater., 2021, 4, 10391–10403 CrossRef CAS.
- P. Zhang, F. R. Qin, L. Zou, M. R. Wang, K. Zhang, Y. Q. Lai and J. Li, Few-layered MoS2/C with expanding d-spacing as a high-performance anode for sodium-ion batteries, Nanoscale, 2017, 9, 12189–12195 RSC.
- G. B. Liu, S. M. Li, J. Mei, L. M. Liu, Y. H. Cui and H. Liu, New insights into low temperature properties of Li-rich layered cathode materials, J. Power Sources, 2017, 353, 51–57 CrossRef CAS.
Footnote |
† Electronic supplementary information (ESI) available. See DOI: 10.1039/d2ma00170e |
|
This journal is © The Royal Society of Chemistry 2022 |
Click here to see how this site uses Cookies. View our privacy policy here.