DOI:
10.1039/D1NJ04662D
(Perspective)
New J. Chem., 2022,
46, 15-38
The journey of C–S bond formation from metal catalysis to electrocatalysis
Received
29th September 2021
, Accepted 4th November 2021
First published on 5th November 2021
Abstract
Due to widespread applications of sulfur-containing frameworks, the construction of the C–S bond has emerged as a useful tool for synthetic organic and medicinal chemists in the recent years. Initially, the C–S bond formations were carried out under transition metal catalysis. The last 10–15 years have been a glorified era for the C–S bond constructions during which various sustainable procedures have been developed. This perspective describes the journey of C–S bond construction starting from transition metal catalysis through oxidant catalysis, photocatalysis and the very recently employed electrocatalysis using various sulfur surrogates.
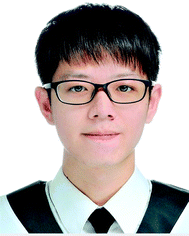
Ze-Wei Chen
| Ze-Wei Chen was born in Kaohsiung, in 1998. He received his BS degree from the Department of Applied Science at National Taitung University (Taiwan) in 2020. He is currently in the second year of his Master's studies under Professor Chin-Fa Lee at the National Chung Hsing University. |
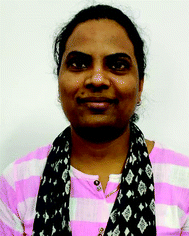
Rekha Bai
| Rekha Bai was born in 1988 in the village Jonaicha Khurd of Alawar District, Rajasthan, India. She received her MSc degree in 2012 and the PhD degree in 2020 (under Dr Satpal Singh Badsara) from the University of Rajasthan, Jaipur, India. After working as a research associate in Dr Badsara's group, very recently, she joined Prof. Chin-Fa Lee's group as a postdoctoral researcher. Her research interests include electro-organic synthesis, and cross-coupling reactions. |
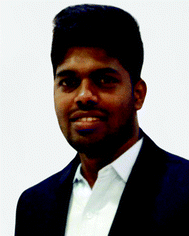
Pratheepkumar Annamalai
| Pratheepkumar Annamalai was born in 1990 at Katchamangalam, a village of Thanjavur, Tamilnadu, India and received his MSc degree (2012) from the Department of Applied Chemistry from the National Institute of Technology, Tiruchirappalli, Tamilnadu, India, and his PhD (2019) from the Department of Applied Chemistry from the National Yang-Ming Chiao Tung University (NYCU) Hsinchu, Taiwan. He is currently undertaking postdoctoral research under Professor Chin-Fa Lee's guidance at the Department of Chemistry, of National Chung Hsing University. His research interests are in the areas of sustainable catalysis and optoelectronic materials. |
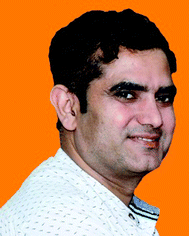
Satpal Singh Badsara
| Satpal Singh Badsara was born in 1983 and obtained his PhD degree from the University of Hyderabad, India in 2013 (under Professor Deevi Basavaiah). During 2013–14, he was a Postdoctoral Fellow under Prof Chin-Fa Lee at the National Chung Hsing University, Taiwan. Currently, he is working as an Assistant Professor at the University of Rajasthan, Jaipur India. He was the recipient of the ISCB Young Scientist Award 2019 in Chemical Science. His research interests include electro-organic synthesis, C–H functionalization, cross-coupling reactions and Baylis–Hillman chemistry. |
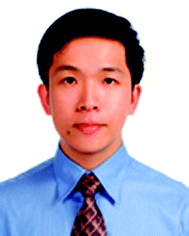
Chin-Fa Lee
| Chin-Fa Lee was born in 1975 and obtained his PhD degree from National Taiwan University in 2002 (under Professor T.-Y. Luh). After spending one postdoctoral year each under Professor T.-Y. Luh at NTU and under Professor J. F. Hartwig at Yale University (2003–2004), he was a Postdoctoral Associate under Professor D. A. Leigh at Edinburgh University from 2005 to 2007. He is currently a Chairman of the Chemistry Department and a Lifetime Distinguished Professor at National Chung Hsing University. His research interests include homogeneous catalysis, optoelectronic materials, and self-assembly chemistry. |
1. Introduction
In recent years, due to the versatile applications of organosulfur compounds, the synthesis of these molecules via C–S bond formation has emerged as a useful tool for synthetic organic and medicinal chemists.1 The organosulfur frameworks show ubiquitous importance in organic synthesis,1 materials science,2a–c natural products,2d,e chemical biology,2f pharmaceutics,2g–ketc. Initially, the organosulfur frameworks were synthesized via transition metal-catalyzed C–S cross-coupling reactions between aryl halides and thiols/thiolates.3 Many transition metals, such as palladium, copper, nickel, iron, indium, cobalt, gold, manganese, silver, etc., and their salts or complexes have shown catalytic efficiency in the synthesis of thioethers and thioesters.1,4 The past decade has witnessed the development of sustainable approaches for the synthesis of organosulfur compounds using a variety of substrates and sulfur surrogates under metal-free conditions via C–H thiolation and cross-coupling reactions.5 Many alternative catalytic systems, such as oxidants, photocatalysis, electro-catalysis, etc., have also emerged as sustainable alternatives to traditional transition metal catalysis.6 Our research group has been actively working in the area of C–S bond formation and has contributed significantly both to metal catalysis as well as sustainable catalysis. In this perspective, we provide a comprehensive discussion about our contributions along with related works to explain the journey of C–S bond formation, starting from transition metal catalysis to sustainable catalysis using a variety of substrates and sulfur surrogates, that is separated into different sections.
2. Transition metal-catalysed C–S bond construction
2.1. Palladium catalysis in C–S bond construction
In 1978, Migita et al.3 employed Pd(PPh3)4 as a catalyst for the first C–S cross-coupling between aryl halides and thiols using NaOtBu as a base in DMSO to provide the corresponding diaryl sulfides or aryl alkyl sulfides in good yields (Scheme 1). As shown in Scheme 2, this reaction proceeds via the oxidative addition of aryl halide on Pd(0) to form intermediate A that reacts with thiolate salts via transmetallation to form the thiolate–Pd complex B. Reductive elimination of B produces diaryl sulfides. In 2004, Itoh and Mase7 developed the palladium-catalyzed C–S bond formation between aryl halide/triflates and thiols using Xantphos (1) as a ligand as shown in Path A of Scheme 3. The resulting thioethers were obtained in 70–92% yields. Similarly, palladium-catalyzed syntheses of thioethers were also developed by Murata and Buchwald8 using the Pd(OAc)2/DiPPF (2) catalytic system, as shown in Path B of Scheme 3.
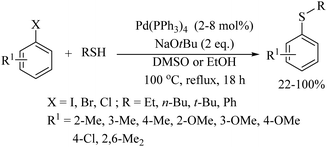 |
| Scheme 1 First C–S cross-coupling between aryl halides and thiols by Migita et al. | |
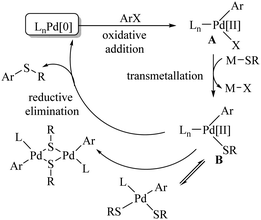 |
| Scheme 2 Mechanistic pathway for palladium-catalysed C–S bond formation. | |
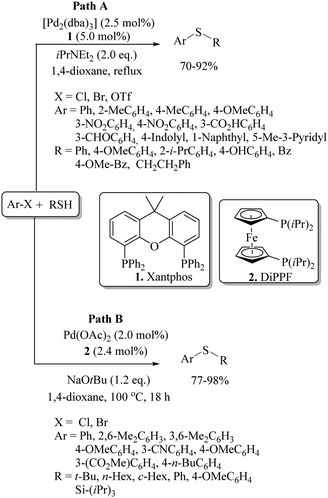 |
| Scheme 3 Palladium-catalysed C–S bond formation between aryl halide/triflates and thiols. | |
In 2006, Hartwig's research group9 contributed to the palladium-catalyzed synthesis of thioethers using aryl chlorides and thiols as coupling partners under the influence of CyPF-t-Bu (3) as a powerful ligand, as shown in Scheme 4. In 2011, Beller and co-workers10 reported the palladium-catalyzed ligand-free C–H thiolation of arenes using aryl sulfonyl cyanide as a sulfur source. The coupling partners possessing different functional groups were well tolerated under the reaction conditions employed to provide the corresponding diaryl sulfides in good to excellent yield, as shown in Scheme 5.
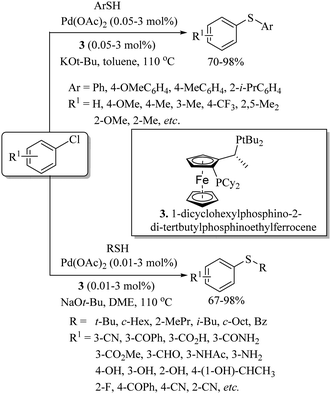 |
| Scheme 4 Palladium-catalysed synthesis of thioethers by Hartwig's research group. | |
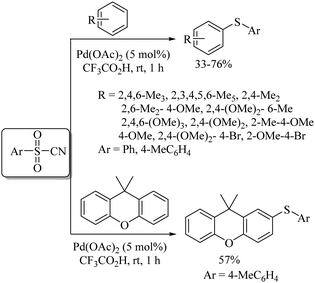 |
| Scheme 5 Palladium-catalysed ligand-free C–H thiolation of arenes using aryl sulfonyl cyanide as a sulfur source. | |
In 2014, Nishihara and co-workers11 reported an interesting palladium-catalysed C–H thiolation process on arenes using disulfides as thiolating agents in the presence of copper as a co-catalyst and phosphines as a ligand, as shown in Scheme 6. This method showed ortho selectivity that was attributed to various directing groups.
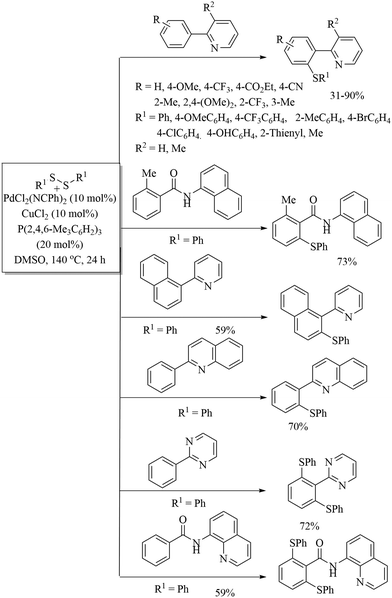 |
| Scheme 6 Palladium-catalysed C–H thiolation process on arenes using disulfides as thiolating agents. | |
In 2014, Jiang and co-workers12 developed a novel palladium-catalyzed synthesis of thioethers using aryl halides/alkyl halides as the aryl/alkyl source, and Na2S2O3·5H2O as a sulfur source, as shown in Scheme 7. In 2015, Messaoudi, Alami, and co-workers13 reported an interesting palladium-catalyzed C–S bond formation between methyl 2-iodobenzoate and peracetylated glycosyl thiols 4 using Xantphos (1) as a ligand to provide the corresponding thioglycosylated products 5 in 31–100% yields. These thioglycosylated products 5 were then further converted into fused thioglycosylated benzoxathiepinones 6via a K2CO3-mediated deprotection process (Scheme 8). In 2018, Wang et al.14 employed KSAc as a source of sulfur for the palladium-catalyzed synthesis of alkyl aryl thioethers via the reaction between aryl/heteroaryl halides and various alkylating reagents such as DMC (dimethyl carbonate), DEC, (diethyl carbonate), DPC (diphenyl carbonate), etc. Representative examples are shown in Scheme 9. The developed strategy was then extended for the synthesis of precursors for various pesticides and for the synthesis of firocoxib (7), a non-steroid anti-inflammatory drug (Scheme 10).
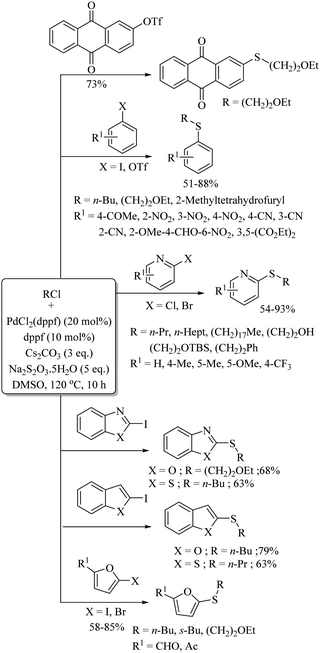 |
| Scheme 7 Palladium-catalysed synthesis of thioethers using Na2S2O3·5H2O as a sulfur source. | |
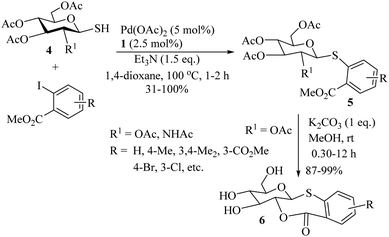 |
| Scheme 8 Palladium-catalysed synthesis of thioglycosylated products. | |
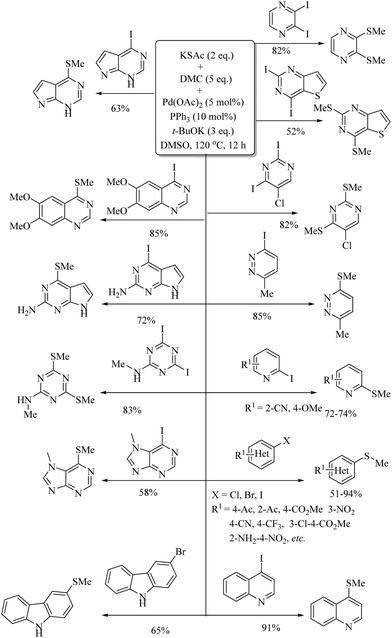 |
| Scheme 9 Palladium-catalysed synthesis of alkyl aryl thioethers using KSAc as a sulfur source. | |
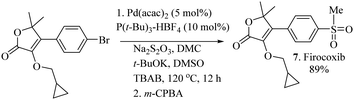 |
| Scheme 10 Synthesis of firocoxib. | |
In 2018, an interesting palladium-catalyzed intramolecular C–H thiolation of masked thiols 8 was developed by Jiang and co-workers.15 The resulting dihydrobenzothiophene 9 and thiochromans 10 were obtained in good to excellent yields, as shown in Scheme 11. The developed method was further extended to obtain a variety of semiconductor moieties such as BTT, BTBT and BBTT (Scheme 11). Sanford and co-workers16 performed a comparative catalytic study between palladium and nickel for the decarbonylation process of thioesters using different ligands, as shown in Scheme 12. When this interesting decarbonylation process was carried out using the Pd[p(o-tol)3]2/PAd2Bn catalytic system, the resulting thioethers were obtained in 27–85% yields, whereas the same reaction under the catalytic influence of Ni(cod)2/PCy3 provided the thioethers up to 99% yields.
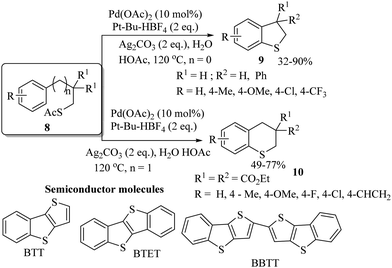 |
| Scheme 11 Synthesis of dihydrobenzothiophene and thiochromans. | |
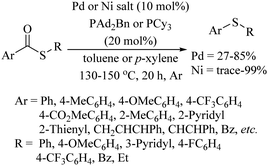 |
| Scheme 12 Decarbonylation process of thioesters. | |
2.2. Nickel catalysis in C–S bond construction
In 1981, Cristau and co-workers17 for the first time found the catalytic application of Ni complex 11 for the formation of C–S bonds between thiolates and aryl halides to afford the corresponding aryl thioethers in 67–100% yields (Path A, Scheme 13). In 1987, Takagi18 also reported a similar type of thioether synthesis via the formation of C–S bonds between various aryl halides and thiols using the NiBr2/dppf catalytic system in the presence of Zn, as shown in Path B of Scheme 13.
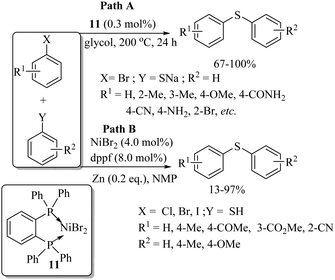 |
| Scheme 13 Nickel-catalysed C–S bond formation between thiolates and aryl halides. | |
Zhang et al.19 for the first time employed an N-heterocyclic carbene (NHC)-based Ni complex catalyst in the presence of a base for the C–S bond construction between a variety of aryl bromides/iodides and thiols to afford the corresponding thioethers in 78–99% yields (Scheme 14). In 2015, an interesting nickel-catalyzed C-sp3 C–H bond-activation process on a substrate possessing an 8-aminoquinoline as a directing group for thiolation using disulfides as thiolating agents was developed by Shi's group20 and by Zhang's group.21 Representative examples20 are presented in Scheme 15.
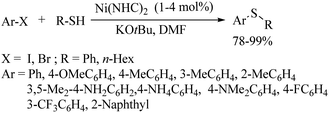 |
| Scheme 14 Ni(NHC)2-catalysed C–S bond formation. | |
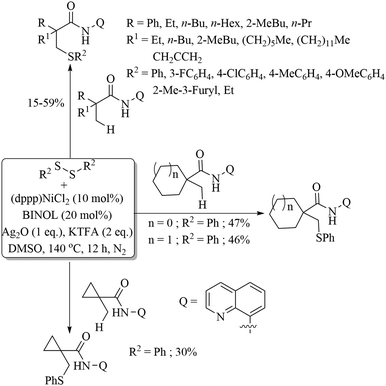 |
| Scheme 15 Nickel-catalysed C–S bond formation via sp3 C–H bond activation. | |
In 2018, Liu and Szostak22 employed the nickel salt/complex [Ni(dppp)Cl2] for the decarbonylation of thioesters in the presence of a base to obtain thioethers, as shown in Path A of Scheme 16. Almost at the same time, Yamauchi and co-workers23 reported a similar nickel-catalysed decarbonylation protocol of thioesters as shown in Path B, Scheme 16.
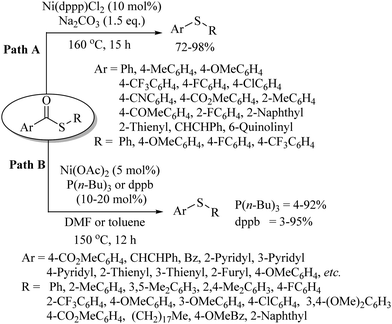 |
| Scheme 16 Nickel-catalysed decarbonylation of thioesters. | |
2.3. Copper catalysis in C–S bond construction
The first copper-catalyzed synthesis of thioethers was developed in 1984 by Yamamoto and Sekine24 using aryl thiols and aryl/alkyl halides. The authors reported two alternative copper-catalyzed procedures for the preparation of thioethers, as shown in Scheme 17. When thiophenols were reacted with metallic copper, CuSAr (12) was obtained, which on reaction with aryl bromides at 270 °C in an autoclave provided the resulting thioethers in 24–67% yields (Path A). Alternatively, the reaction between thiols and aryl iodides in the presence of Cu powder at 240–300 °C provided the resulting thioethers in 18–88% yields via Path B.
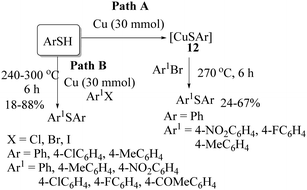 |
| Scheme 17 First copper-catalysed synthesis of thioethers. | |
Later on, in 2002 Kwong and Buchwald25 used CuI as the catalyst for C–S bond formation between aryl iodide and thiols in the presence of ethyl glycol as a ligand and K2CO3 as a base to provide the corresponding thioethers in 71–95% yields (Scheme 18).
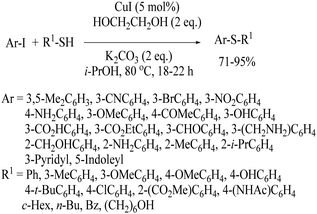 |
| Scheme 18 CuI-catalysed C–S bond formation between aryl iodide and thiols. | |
Similarly, Naus and co-workers26 also used CuI for the arylation of sugar thiols 13 with o-haloaryl triazenes 14 in the presence of K2CO3/pyridine, as shown in Path A of Scheme 19. The authors also extended the developed procedure to the diarylation of sugar thiols, i.e., 1-thioglycopyranoses 13 with a variety of 2,6-dihaloaryl triazenes 15 and the results are shown in Path B, Scheme 19.
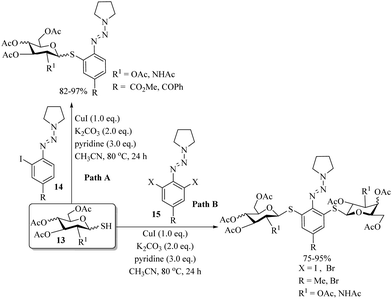 |
| Scheme 19 CuI-catalysed arylation of sugar thiols. | |
In 2002, Liebeskind and co-workers27 developed an alternative synthesis of thioethers using N-thioimides 16 as a thiolating agent for the copper-catalyzed C–S bond formation with boronic acids, as shown in Path A of Scheme 20. A variety of aryl and alkyl boronic acids underwent CuMeSal-catalyzed C–S bond formation with aromatic and heteroaromatic thiols to provide the corresponding thioethers in 51–79% yields. Later on, in 2014, Jiang and co-workers28 performed a copper-catalyzed Chan–Lam reaction of S8 with boronic acids to afford the aryl thioethers shown in Path B, Scheme 20. Similarly, in 2015, Tian and co-workers29 developed a copper-catalyzed synthesis of thioethers via the C–S bond formation between boronic acids and sulfonyl hydrazides as shown in Path C, Scheme 20.
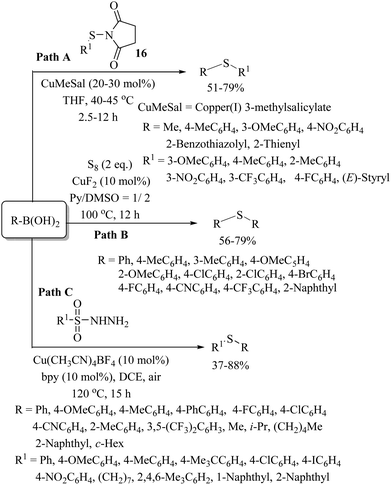 |
| Scheme 20 Copper-catalysed synthesis of thioethers from boronic acids. | |
In 2006, Yu and co-workers30 for the first time reported an interesting Cu(II)-catalyzed direct C–H thiolation of phenyl pyridine (17) with thiols or disulfides, as shown in Scheme 21. The plausible mechanistic pathway for this protocol is believed to proceed via Cu(II)-mediated single-electron transfer (SET), and ortho selectivity was achieved through intramolecular anion transfer via a nitrogen-bound Cu(I) ate complex.
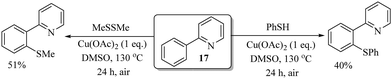 |
| Scheme 21 Cu-Catalysed direct C–H thiolation of phenyl pyridine. | |
In 2010, Lee's research group31 prepared an efficient catalyst of CuO on mesoporous silica and employed it for C–S bond formation between a variety of aryl iodides and thiols in the presence of K2CO3 as a base, which provided the resulting thioethers in 66–97% yields (Path A, Scheme 22). This catalytic system was also found to be suitable for C–S coupling between aryl iodides and heteroaromatic thiols (Path B, Scheme 22). In 2011, Lee's research group32 also employed Cu2O along with 1,10-phenanthroline as a catalytic system for C–S bond formation between a variety of vinyl halides (with aryl as well as alkyl substitution) and thiols to provide the resulting thioethers in good to excellent yields as shown in Scheme 23. It is worthy of mention here that, for the C–S coupling between vinyl iodides and thiols, only 0.5 mol% of Cu2O was required (Path A, Scheme 23), whereas for vinyl bromide and chloride, 5 mol% of Cu2O was required (Path B, Scheme 23). It was also observed that the C–S coupling between vinyl bromide and chloride with thiol can also be performed under catalyst-free conditions to afford the corresponding thioethers in excellent yields (Path C, Scheme 23).
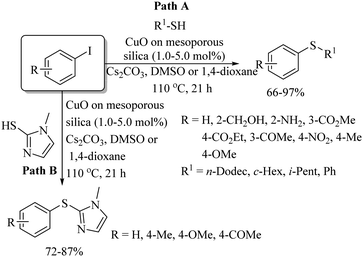 |
| Scheme 22 Synthesis of thioethers catalysed by CuO on mesoporous silica. | |
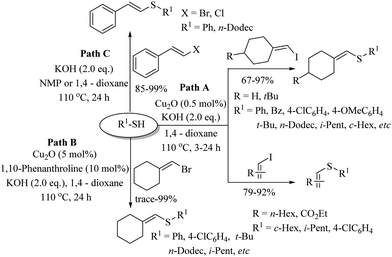 |
| Scheme 23 Cu2O-catalysed C–S bond formation between vinyl halides and thiols. | |
In 2012, an interesting iridium-catalyzed meta-C–H borylation process followed by copper-catalyzed C–S bond formation was also developed by Lee and co-workers.33 In a one-pot synthetic procedure, first aryl boronic esters were prepared in situ via an Ir[(cod)OMe]2-catalyzed meta-borylation of arenes/heteroarenes. The volatile compounds were then removed and the crude mixture upon subsequent treatment with aryl disulfides under the catalytic influence of CuCl provided thioethers in 46–88% yields, as shown in Scheme 24. In 2013, Bolm's research group34 also employed copper as a catalyst for C–S bond formation between β-diketones/esters and disulfides via a decarbonylation process to afford the corresponding α-thioaryl ketone/esters in 30–99% yields, as shown in Scheme 25.
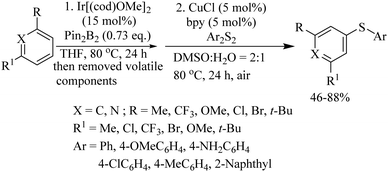 |
| Scheme 24 Iridium-catalysed meta-C–H borylation followed by copper-catalyzed C–S bond formation. | |
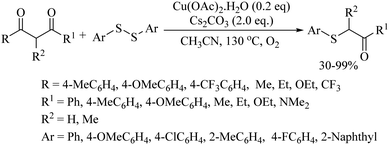 |
| Scheme 25 Copper-catalysed C–S bond formation between β-diketones/esters and disulfides. | |
Our research group35,36 then studied the catalytic efficiency of CuO for C–S coupling between aryl iodides and thiols using Cs2CO3 as a base to provide the resulting thioethers in 36–99% yields (Path A, Scheme 26). CuO was also found to be a suitable catalyst under microwave conditions for C–S bond formation between aryl iodides and thiols (Path B, Scheme 26).
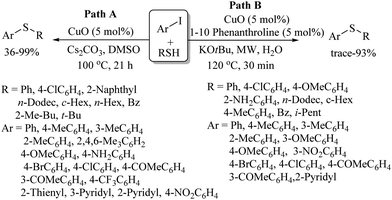 |
| Scheme 26 CuO-catalysed C–S coupling between aryl iodides and thiols. | |
A facial copper-catalyzed synthetic protocol for the preparation of benzoisothiazolones via C–S/N–S bond formation through a C–H activation process on arenes possessing a bidentate directing group (pyridine-2-yl)isopropyl amine (PIP-amine) (18) and S8 as a sulfur source was developed by Chen et al.,37 as shown in Scheme 27 in which representative examples are presented.
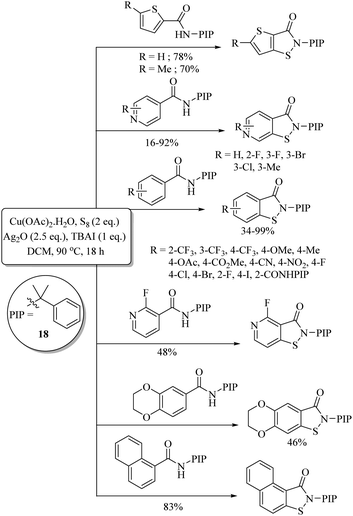 |
| Scheme 27 Copper-catalysed synthesis of benzoisothiazolones. | |
In 2015, Sekar's research group38 developed an efficient copper-catalyzed domino process for the synthesis of thioflavanones via the C–S bond formation between 2′-iodochalcones 19 (Path A) or 2′-bromochalcones 20 (Path B) and xanthate (21) as a sulfur source, as shown in Scheme 28. Later on, Sangeetha and Sekar39 also developed a copper-catalyzed synthesis of 2,3-dihydrobenzo[b]thiophene frameworks 22via a similar type of domino C–S bond formation between 2-iodoketones and commercially available xanthate (21) as a sulfur surrogate, as shown in Path C, Scheme 28. Interestingly, when the reaction was performed under the same catalytic system and conditions in the presence of H2SO4, it provided benzothiophene derivatives 23 (Path D, Scheme 28).
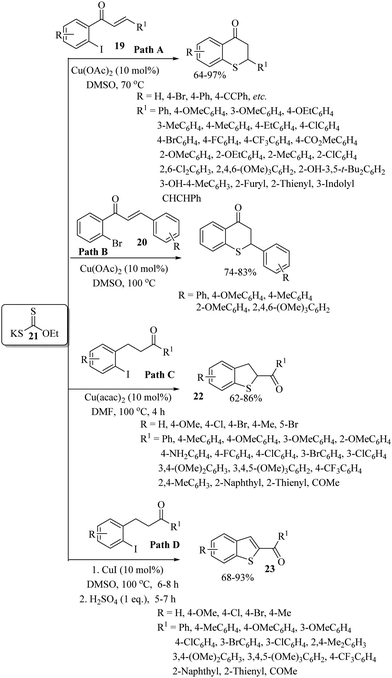 |
| Scheme 28 Xanthate as a sulfur source for C–S bond formation. | |
Messaoudi and co-workers40 reported a thioglycosylation process of the C(sp2)–H bonds of benzamide derivatives with the variety of thiosugars 24 using Cu(OAc)2·H2O as a catalyst and Ag2CO3 as an additive to afford the resulting products in good yields with exclusive β-selectivity. Representative examples are shown in Scheme 29. In 2017, Lee's research group41 developed a highly regioselective copper-catalyzed synthetic protocol for the preparation of thioethers starting from arenes. In a one-pot operation, first anisoles were injected for p-selective bromination under the catalytic influence of FeCl3 using NBS as a brominating agent. The reaction mixtures were then filtered and the filtrates were then treated with a variety of thiols using CuI as the catalyst and N,N′-dihexyloxalamide (25) as a ligand to afford the corresponding thioethers in good to excellent yields (Scheme 30). CuI along with ligand 25 was also found to be suitable for C–S bond formation between different haloarenes and thiols, as shown in Scheme 31.42
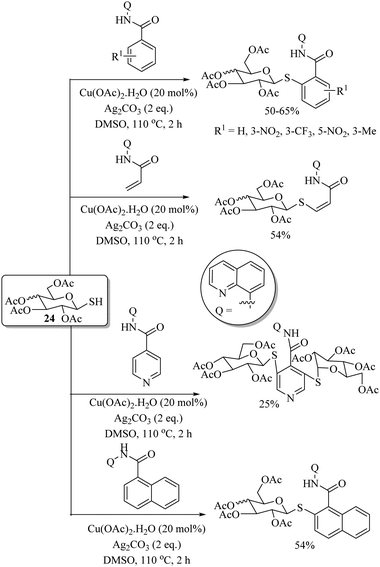 |
| Scheme 29 Thioglycosylation of the C(sp2)–H bonds of benzamide derivatives. | |
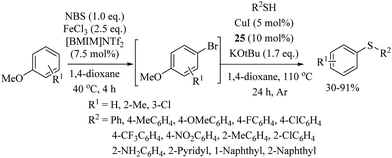 |
| Scheme 30 Copper-catalysed synthesis of thioethers from arenes. | |
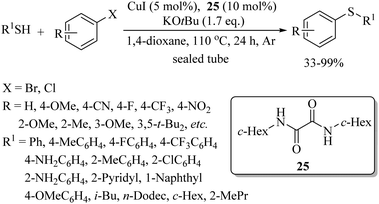 |
| Scheme 31 CuI-catalysed synthesis of thioethers. | |
Very recently, Ma and co-workers43 employed sodium sulfinate as a thiolating agent for copper-catalyzed C–S bond formation with aryl iodides, as shown in Scheme 32.
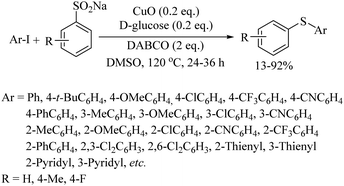 |
| Scheme 32 Sodium sulfinate as a sulfur source for C–S bond formation. | |
Lee's research group44 developed an interesting copper-catalyzed C–H thiolation of aldehydes with a variety of thiols in the presence of TBHP in aqueous media (Scheme 33). Both the alkyl and aryl thiols coupled well with both aromatic and heteroaromatic aldehydes under the reaction conditions employed to provide the resulting thioethers in 31–94% yields (Path A, Scheme 33).44a It is worthy of mention here that aliphatic aldehydes were not found to be suitable substrates in this method. When the authors performed the same C–H thiolation process of aldehydes with thiols under microwave conditions using the same catalytic system, both the aryl and alkyl aldehydes coupled well with thiols to provide the resulting thioesters in 38–99% yields (Path B, Scheme 33).44b Patel and co-workers45 also developed an interesting copper-catalyzed cross-dehydrogenative S-arylation process of thiols using methyl arenes as aryl surrogates in the presence of TBHP as an oxidant, as shown in Scheme 34. The resulting thioethers were obtained in good to excellent yields without the assistance of any directing group.
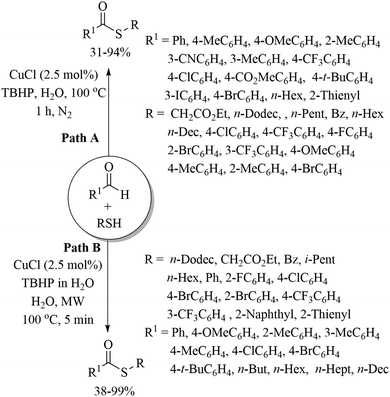 |
| Scheme 33 Copper-catalysed C–H thiolation of aldehydes with thiols. | |
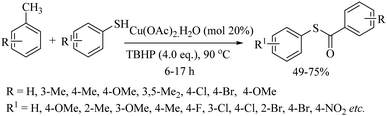 |
| Scheme 34 Copper-catalysed oxidative C–H thiolation of methyl arenes with thiols. | |
In 2016, Jiang and co-workers46 reported an interesting synthesis of unsymmetrical disulfides via the copper-catalyzed oxidative cross-coupling of arylboronic acids with masked disulfides 26, as shown in Scheme 35. The desulfurizing reagents, i.e., masked disulfides 26, were synthesised from sodium 4-methylbenzenesulfonothioate in a two-step process as shown in Scheme 35. Representative examples are presented.
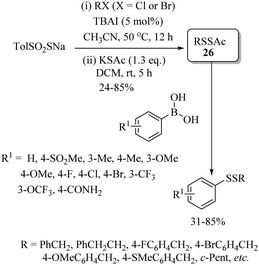 |
| Scheme 35 Synthesis of unsymmetrical disulfides. | |
2.4. Cobalt catalysis in C–S bond construction
Cobalt was first employed as a catalyst for C–S bond formation by Cheng and co-workers.47 This group used a variety of bromo- and iodo-arenes for C–S bond formation with thiols in the presence of the CoI2(dppe) catalyst using Zn as a co-catalyst to afford the corresponding thioethers as shown in Scheme 36. Interestingly, this catalytic system was also found to be effective for C–S coupling between vinyl iodides and thiols. Later on, Dong et al.48 also extended the applicability of cobalt catalysis to C–S bond formation between aryl zinc pivalates and diaryl disulfides, as shown in Scheme 37. Various electron-rich and electron-poor organozinc pivalates were found to be suitable for C–S coupling with different aryl disulfides, and the resulting thioethers were obtained in 60–95% yields.
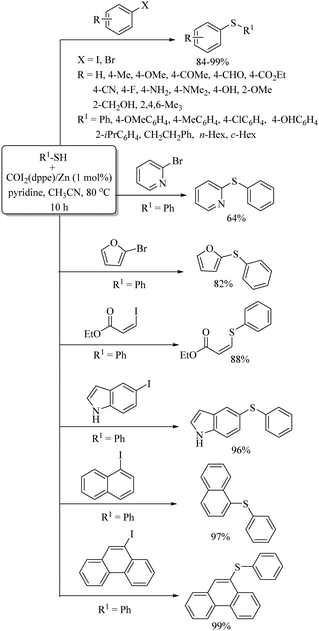 |
| Scheme 36 Cobalt-catalysed C–S bond formation between aryl halides and thiols. | |
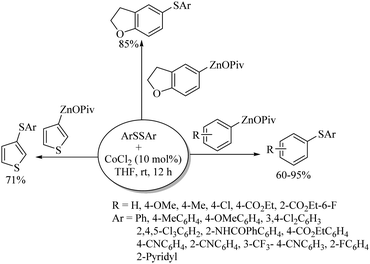 |
| Scheme 37 Cobalt-catalysed C–S bond formation between organozinc pivalates and disulfides. | |
2.5. Iron catalysis in C–S bond construction
In 2008, Bolm and co-workers49 for the first time reported the catalytic applicability of iron salts for C–S bond formation. After screening different iron salts, they observed that FeCl3 with DMEDA (27) as a ligand was suitable for the C–S bond construction between a variety of aryl iodides and thiols to afford the resulting thioethers in 32–98% yields (Scheme 38). It is worthy of mention here that only aryl iodides were found to be suitable coupling partners, and aryl chlorides and bromides could not couple with thiols under this catalytic system. Also, FeCl3 was not suitable for C–S coupling between aryl iodides and alkyl thiols.
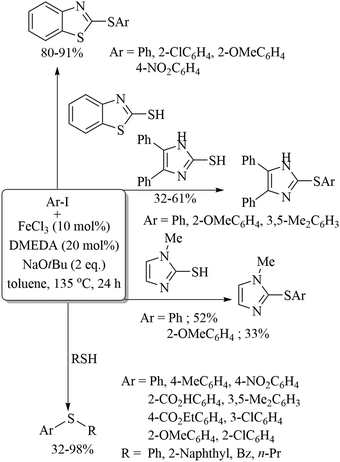 |
| Scheme 38 FeCl3-catalysed C–S bond formation between aryl iodides and thiols. | |
In 2009, almost at the same time, our research group50 and Tsai's research group51 also developed iron-catalyzed synthetic protocols for thioethers via the coupling between aryl iodides and thiols (Scheme 39). We carried out the C–S bond-forming reaction between aryl iodides and thiols (both alkyl and aryl) in the presence of FeCl3 using phosphine ligands 1 and 28 and NaOtBu, which affords the corresponding thioethers in 62–99% yields (Paths A and B, Scheme 39). Aryl iodides that possess both electron-rich and electron-poor groups reacted well with both aryl and alkyl thiols under the reaction conditions employed. Tsai's group51 used ligand 29 with FeCl3 for C–S bond formation between aryl iodides and thiols (Path C, Scheme 39). However, low yields were obtained in the case of alkyl thiols. Later on in 2012, Lee and co-workers52 also found FeCl3 to be a suitable catalyst with phosphine (1) for the C–S coupling reaction between vinyl halides and thiols (Scheme 40). The catalytic system was found to be suitable for the C–S coupling of vinyl chloride, bromide and iodide with thiols to afford the desired vinyl thioethers in good yields.
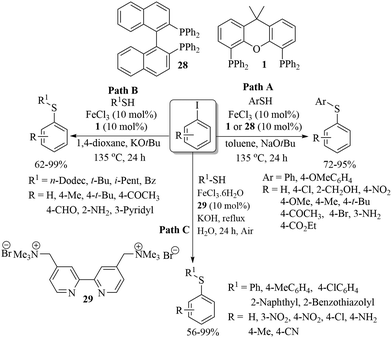 |
| Scheme 39 FeCl3-catalysed synthesis of thioethers. | |
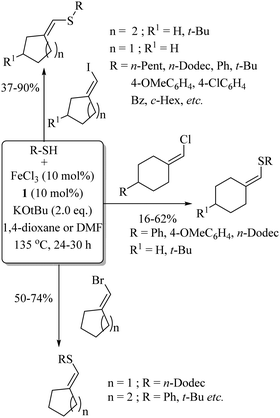 |
| Scheme 40 FeCl3-catalysed C–S bond formation between vinyl halides and thiols. | |
In 2012, Lei and co-workers53 also employed FeCl3 along with Na2S2O8 as a catalytic system for the preparation of benzothiazole derivatives 30via intramolecular C–H functionalization and C–S bond formation as shown in Scheme 41. Representative examples are presented. Ranu and co-workers54 used iron nanoparticles with tBuONO for the coupling between amine and disulfides to provide the corresponding thioethers in 70–81% yields (Scheme 42). In 2014, Lee's research group55 developed an interesting FeBr2-catalyzed C–H thiolation process of aldehydes with thiols in the presence of TBHP in aqueous media, as shown in Scheme 43. Both, the aryl and alkyl aldehydes were coupled with thiols under the influence of this catalytic system.
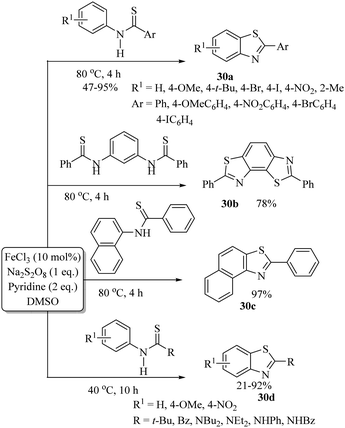 |
| Scheme 41 FeCl3/Na2S2O8-catalysed synthesis of benzothiazoles. | |
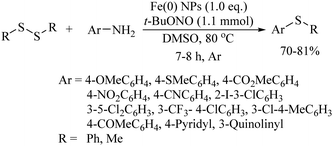 |
| Scheme 42 Iron nanoparticle-catalyzed synthesis of thioethers. | |
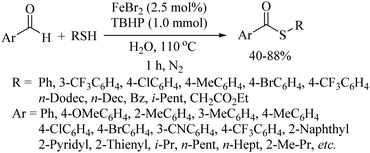 |
| Scheme 43 FeBr2-catalysed C–H thiolation of aldehydes with thiols. | |
2.6. Miscellaneous metal catalysis in C–S bond construction
Yadavalli and co-workers56 developed a manganese-catalyzed C–S bond formation between aryl iodides and thiols (Path A, Scheme 44). This group also extended this method to vinyl iodides with thiols (Path B, Scheme 44). Lee's research group57 also reported a manganese-catalyzed cross-coupling reaction of aryl iodides with thiols using 31 or 32 as ligands to afford the corresponding aryl thioethers in good to excellent yields (Path C, Scheme 44).
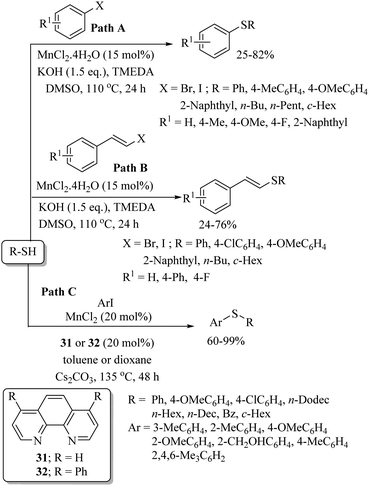 |
| Scheme 44 Manganese-catalysed synthesis of thioethers. | |
An interesting rhodium-catalyzed C–S bond formation reaction between aryl fluorides and aryl disulfides was reported by Yamaguchi and co-workers58a,b (Scheme 45). A variety of aryl fluorides possessing both electron-donating as well as electron-withdrawing functionalities underwent C–S bond formation with disulfides under the influence of RhH(PPh3)4 in the presence of excess dppBz to afford the resulting thioethers in good to excellent yields. Interestingly, the fluoro group was found to be more prone towards C–S bond formation compared with the bromo and chloro groups. The authors58a also studied the polyarylthiolation reaction on polyfluorobenzenes with disulfides, and interestingly observed that the product formation depends on the amount of dppBz and PPh3, as shown in Scheme 45, i.e., an enhancement in the amounts of dppBz and PPh3 leads to the formation of a large amounts of polyarylthiolated products. Later on, the same research group58c also extended this interesting strategy to the synthesis of symmetrical polyfluorinated diaryl sulfides using S8 or di-tert-butyl tetrasulfides as a sulfur source via the C–S cross-coupling reaction as shown in Scheme 46.
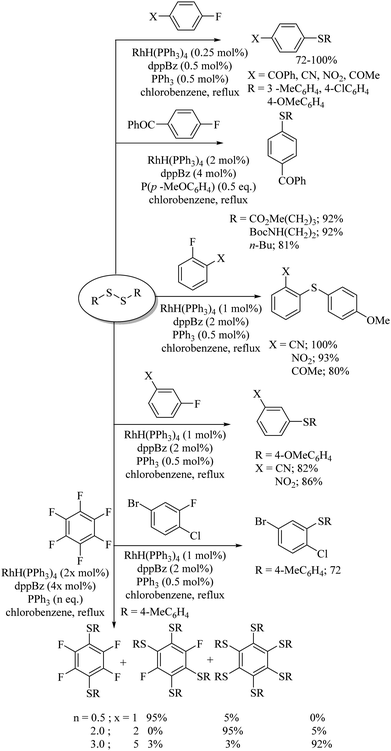 |
| Scheme 45 Rhodium-catalysed C–S bond formation between aryl fluorides and aryl disulfides. | |
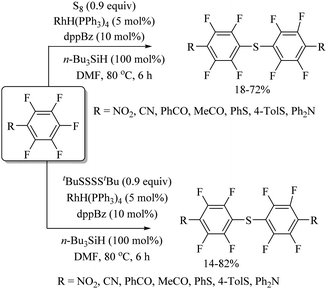 |
| Scheme 46 Synthesis of polyfluorinated diaryl sulfides. | |
Yamaguchi and co-workers58d also developed the rhodium-catalyzed synthesis of fluorinated dithioarylbenzenes via an acyl transfer reaction between thioesters and hexafluorobenzene, as shown in Scheme 47. A rhodium-catalysed synthesis of unsymmetrical diaryl sulfides via aryl exchange between symmetrical diaryl sulfides and aromatic fluorides was also reported by Yamaguchi's research group (Scheme 48).58e
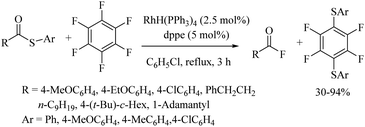 |
| Scheme 47 Rh-Catalysed synthesis of diarylthio tetrafluorobenzenes via acyl cleavage. | |
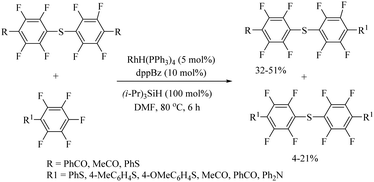 |
| Scheme 48 Rh-Catalysed synthesis of unsymmetrical diaryl sulfides via aryl exchange. | |
Zhou, Li, and co-workers59 also reported a rhodium-catalyzed C–H thiolation process on arenes possessing directing groups using disulfides as sulfur surrogates, as shown in Scheme 49. A variety of arenes underwent C–H thiolation with aryl and alkyl disulfides under the catalytic influence of [RhCp*Cl2]2 and under the influence of AgOTf/Cu(OAc)2, providing the resulting monothioethers (Paths A–C, Scheme 49), whereas AgOTf/Ag2CO3 resulted in bis-thioethers (Path D, Scheme 49). In 2021, Rui et al.60 reported a one-pot atom-economic synthesis of structurally important phenothiazines via the Rh(III)-catalyzed direct C–H thiolation of acetanilide with 2-bromothiophenol followed by an intramolecular C–N bond formation reaction sequence as shown in Path A of Scheme 50. The developed protocol was further extended to the gram-scale synthesis of the drug molecule chlorpromazine (33) (Path B, Scheme 50).
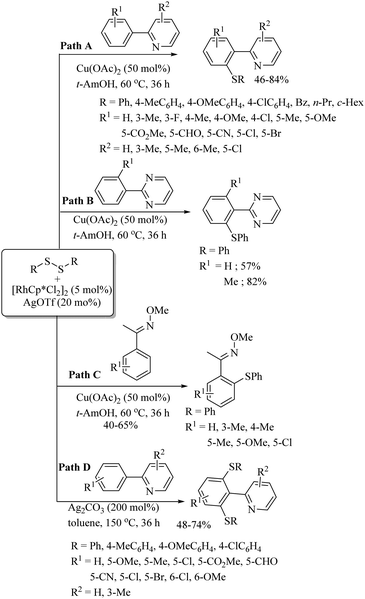 |
| Scheme 49 Rhodium-catalysed C–H thiolation of arenes. | |
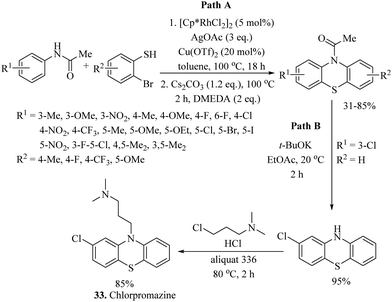 |
| Scheme 50 Rhodium-catalysed C–H thiolation of acetanilide and synthesis of the drug molecule chlorpromazine. | |
An interesting iridium-catalyzed decarbonylation-C–H bis-arylsulfenylation of indoles at the C-2 and C-4 C–H bonds using disulfides was reported by Kathiravan et al.61 A variety of indole frameworks possessing C-2, and C-4 C–H bonds underwent C–S bond formation under the influence of the pentamethylcyclopentadienyl iridium dichloride dimer ([Cp*IrCl2]2) as a catalyst to afford the resulting bis-thioethers in 32–76% yields (Scheme 51).
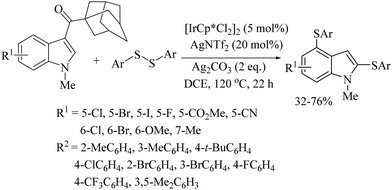 |
| Scheme 51 Iridium-catalysed decarbonylation-C–H bis-arylsulfenylation of indoles. | |
Baidya and co-workers62 performed a ruthenium-catalyzed o-C–H thiolation of aromatic and heteroaromatic carboxylic acids using disulfides as a thiolating agent, as shown in Scheme 52. The resulting o-thioethers were then utilized for the synthesis of thioxanthones 34via treatment with TfOH.
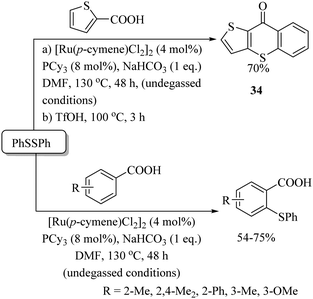 |
| Scheme 52 Ruthenium-catalysed o-C–H thiolation of carboxylic acids. | |
A silver-catalyzed synthetic protocol for diaryl thioethers from arylboronic acids and aryl thiols using KOH as a base was developed in 2012 by Chakraborty and Das,63 as shown in Scheme 53.
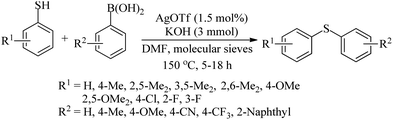 |
| Scheme 53 Silver-catalysed C–S bond formation between arylboronic acids and aryl thiols. | |
Rao and co-workers64 employed In(OTf)3 along with TMEDA (35) as a ligand for C–S bond formation between aryl halides and thiols, as shown in Path A of Scheme 54. Rao's research group65 also performed the same C–S coupling reaction using In2O3 nanoparticles in the absence of ligands using KOH as a base to afford the resulting thioethers in 5–97% yields (Path B, Scheme 54).
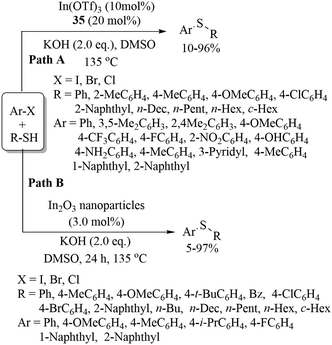 |
| Scheme 54 In(OTf)3-catalysed C–S bond formation between aryl halides and thiols. | |
3. Oxidant-mediated C–S bond construction
Various research groups66–70 have employed iodine as a catalyst for the C–H thiolation process on various substituents using a variety of sulfur surrogates. Representative examples are shown in Schemes 55 and 56. Similarly, other iodide sources, such as NH4I, PhI(OAc)2, KI, etc., were also found to be suitable for the C–H thiolation process, as shown in Scheme 57.71–73
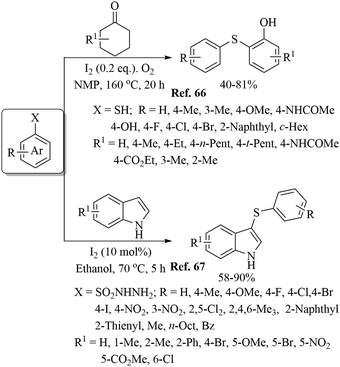 |
| Scheme 55 Iodine-catalysed C–H thiolation of cyclic ketones and indoles. | |
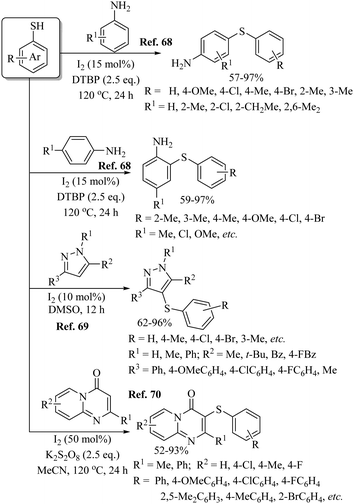 |
| Scheme 56 Iodine-catalysed C–H thiolation using thiols as thiolating agents. | |
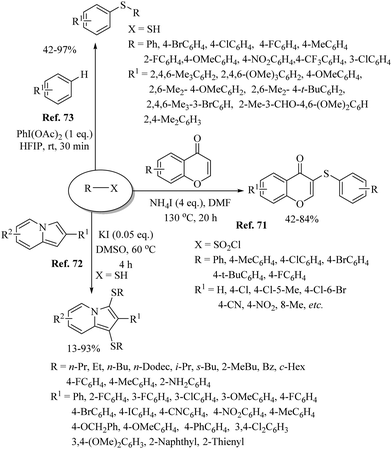 |
| Scheme 57 C–H thiolation process catalysed by different iodide sources. | |
An interesting I2/DTBP-catalyzed C–H thiolation process for the sp3 C–H bond of methyl arenes/alkanes with thiols was reported by Lie and co-workers,74 as shown in Scheme 58. A variety of methyl arenes/alkanes underwent C–S bond formation with different thiols to afford the corresponding thioethers in 33–90% yields. In 2013, Guo et al.75 reported a facile DTBP-promoted direct oxidative thiolation of the sp3 C–H bond of ethers with disulfides to afford the resulting alkyl aryl thioethers in good to excellent yields (Paths A and B in Scheme 59). This protocol was also extended to the sp3 C–H thiolation of amides (Path C, Scheme 59). Later on, Han and co-workers76 also employed DTBP as a catalyst for the sp3 C–H bond thiolation of cycloalkanes with disulfides as shown in Path D of Scheme 59. Almost at the same time, Sun and co-workers77 used TBHP for the sp3 C–H bond thiolation of alkanes with disulfides, as shown in Path E, Scheme 59. Lee's research group78 developed an interesting iodine-catalyzed oxidative C–S coupling reaction between 1,3-diketones and disulfides using K2S2O8 as an oxidant to afford the α-thio-β-dicarbonyl compounds as shown in Path A of Scheme 60. Almost at the same time, Lie, Hu, and co-workers79 also reported similar types of oxidative C–S coupling between 1,3-diketones and thiols using the I2/DTBP catalytic system as shown in Path B, Scheme 60. Later on, in 2018, Wang and co-workers80 performed the oxidative C–S bond formation of 1,3-diketone frameworks with thiols using KI as a catalyst, as shown in Path C of Scheme 60.
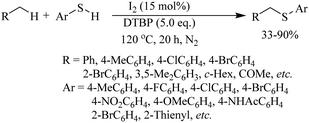 |
| Scheme 58 I2/DTBP-catalysed sp3C–H thiolation process for the C–H bond of methyl arenes. | |
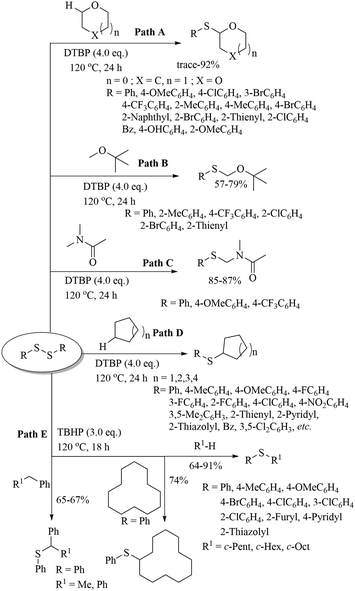 |
| Scheme 59 I2/oxidant-catalysed sp3C–H thiolation process. | |
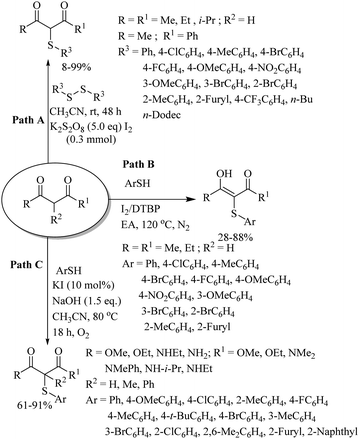 |
| Scheme 60 Oxidative C–S bond formation between 1,3-diketone frameworks and thiols. | |
In 2017, Badsara and co-workers81,82 developed the interesting solvent-dependent regio- and stereo-selective syntheses of allylic thioethers 36–39 under metal-free conditions (Scheme 61). The C–S coupling of allyl halides with disulfides was carried out under the influence of DCP using DMSO/DPSO as a solvent to provide allylic thioethers 36 and 37 with complete stereo- and regio-selectivity (Paths A and B, Scheme 61).81 However, the same reaction using CH3CN as the solvent and DTBP as the oxidant provided aryl/alkyl allyl thioethers 38 and 39 in 63–92% yields with complete stereo- and regio-selectivity (Paths C and D, Scheme 61).82
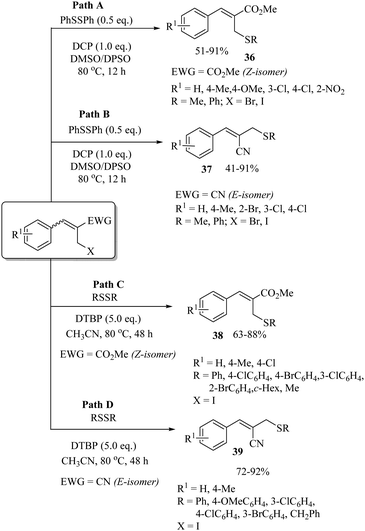 |
| Scheme 61 Regio- and stereo-selective syntheses of allylic thioethers. | |
Lee's research group83 developed a facile I2-catalyzed synthetic protocol for o-hydroxybenzyl thioethers via C–S bond formation between o-hydroxybenzyl alcohols 40 and thiols (Scheme 62). The reaction proceeded via the in situ preparation of o-quinone methides that upon thio-Michael reaction with thiols resulted in the corresponding o-hydroxybenzyl thioethers as shown in Path A, Scheme 62. The developed protocol was further extended to functionalized thiols 41 as shown in Path B, Scheme 62.
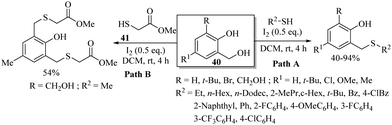 |
| Scheme 62 I2-catalysed synthesis of o-hydroxybenzyl thioethers. | |
Zhu and co-workers84 reported the tetraethylammonium bromide (TEAB)-catalyzed oxidative thioesterification of aldehydes and alcohols with thiols using K2S2O8 as an oxidant, as shown in Scheme 63. A variety of aromatic and heteroaromatic alcohols and aldehydes underwent oxidative C–S bond formation with different thiols to provide the corresponding thioesters in good to excellent yields.
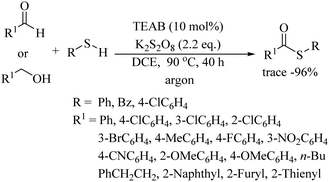 |
| Scheme 63 TEAB/K2S2O8-catalysed oxidative thioesterification of aldehydes and alcohols. | |
Our research group85 also developed an interesting DTBP-catalyzed oxidative C–H thiolation of the aldehydic C–H bond with disulfides as shown in Scheme 64. Both aromatic as well as heteroaromatic aldehydes possessing an electron-rich or electron-poor functionality underwent C–H thiolation with both aryl and alkyl disulfides to afford the corresponding thioethers in 40–99% yields.
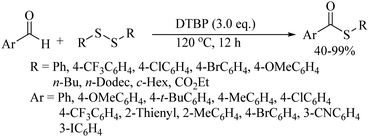 |
| Scheme 64 DTBP-catalysed oxidative C–H thiolation of aldehydes. | |
Subsequently, in 2014, our research group86 also performed the temperature-dependent metal-free DTBP-catalysed oxidative C–H thiolation process of the sp3 C–H bond of methyl arenes/heteroarenes with disulfides to provide the corresponding thioesters in 47–88% yields (Scheme 65). Methyl arenes and heteroarenes possessing different functionalities underwent oxidative C–H thiolation with both aryl and alkyl disulfides, as shown in Path A, Scheme 65. Interestingly, the reaction of ethyl arenes with disulfides under the influence of DTBP could yield only the thioether via thiolation, as shown in Path B, Scheme 65.
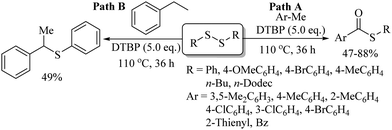 |
| Scheme 65 DTBP-catalysed oxidative C–H thiolation process of the sp3 C–H bond of methyl arenes/heteroarenes with disulfides. | |
A variety of other catalysts/catalytic systems, such as VA004/CTAB, DMP (Dess–Martin periodinane), DBU, etc., were also employed for the thioesterification of aldehydes with thiol/disulfides by various research groups, as shown in Paths A–D of Scheme 66.87–90
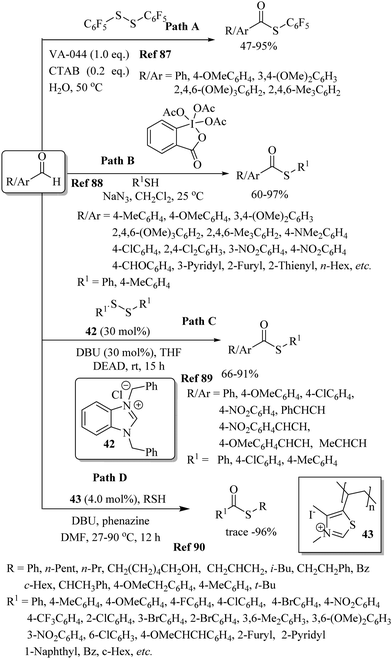 |
| Scheme 66 Thioesterification of aldehydes with thiols/disulfides. | |
4. Photocatalyzed C–S bond construction
Fu, Peters, and co-workers91 developed for the first time a photoinduced copper-catalyzed C–S cross-coupling reaction between aryl halides and thiols following a single-electron-transfer process. Both aromatic and heteroaromatic halides and thiols possessing electron-rich as well as electron-poor functionalities underwent C–S bond formation to afford the corresponding thioethers in 59–90% yields (Path A, Scheme 67). Later on, Fu and co-workers92 also reported the room-temperature visible-light-induced photo redox arylation of thiols with aryl halides using 44 as a photocatalyst, as shown in Path B, Scheme 67. This photoinduced protocol was found to be effective on aryl chlorides and both the aryl and alkyl thiols coupled well under the reaction conditions employed. Later on, in 2017, an interesting visible-light-promoted C–S cross-coupling reaction between aryl halides and thiols in the presence of Cs2CO3 without using any photocatalyst was developed by Miyake and co-workers93 (Path C, Scheme 67). The authors93 also demonstrated the pharmaceutical utility of the developed protocol for the synthesis of pharmaceutical ingredients such as indomethacin, fenofibrate, moclobemide, hydrochlorothiazide, etc., via late-stage modification. Majek and Wangelin94 also developed a visible-light-mediated C–S bond-formation reaction between the arene diazonium salt and dimethyl disulfides using Eosin Y as a photocatalyst to afford the corresponding aryl methyl thioethers in 31–89% yields (Scheme 68). The reaction also proceeded via a single-electron-transfer mechanism.
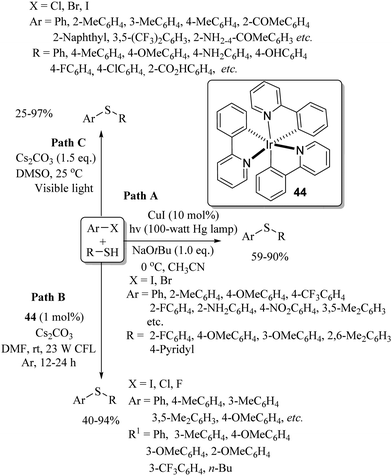 |
| Scheme 67 Photoinduced C–S cross-coupling reactions between aryl halides and thiols. | |
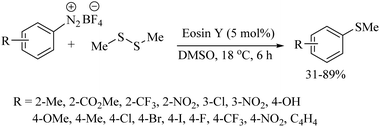 |
| Scheme 68 Visible-light-mediated C–S bond formation between the arene diazonium salts and dimethyl disulfides. | |
In 2018, Hajra's research group95 also developed the visible-light-mediated synthesis of aryl thioethers via a cross-coupling reaction between aryl hydrazines and thiols using rose bengal as a photocatalyst, as shown in Scheme 69.
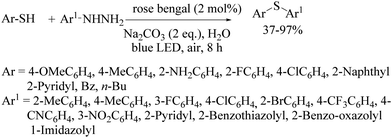 |
| Scheme 69 Visible-light-mediated C–S cross-coupling reaction between aryl hydrazines and thiols. | |
Lee's research group96 also developed an interesting light-induced tBuONO-catalyzed C–S bond-formation reaction between anilines and thiols using a 10 W blue LED as a light source in the absence of photocatalyst. The resulting thioethers were obtained in 33–96% yields as shown in Scheme 70.
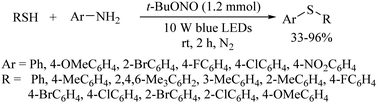 |
| Scheme 70 Light-induced C–S bond formation between anilines and thiols. | |
The first visible-light photo-reduced thioacetalization process of aldehydes with thiols using Eosin Y as a photocatalyst to afford dithioacetals under metal-free and solvent-free conditions was developed by Lee's research group.97 A variety of aromatic, heteroaromatic and α,β-unsaturated aldehydes underwent the thioacetalization process with aromatic aliphatic thiols to afford the resulting diacetals in 30–99% yields (Path A, Scheme 71). This thioacetalization proceeded via a radical pathway and the developed protocol was further extended to the preparation of cyclic dithioacetals (Path B, Scheme 71). Lee's research group98 also employed the visible-light metal-free protocol for the oxathiacetalization of aldehydes and ketones with 2-mercaptoethanol/3-mercaptopropanol using Eosin Y as the photocatalyst to afford the resulting 1,3-oxathiolanes and 1,3-oxathianes, respectively (Path C, Scheme 71 in which representative examples are presented). Sekhar and co-workers99 reported a facile visible-light-induced synthesis of heteroaryl aryl thioethers via I2/TBHP-catalyzed C–S bond formation between heteroarenes and thiols as shown in Scheme 72. This one-pot synthesis of thioethers involves two sequential reactions, i.e., formation of the iodinated heteroarenes followed by C–S coupling reactions. Representative examples are presented in Scheme 72.
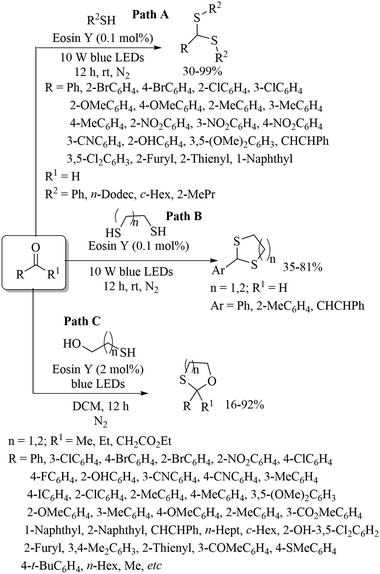 |
| Scheme 71 Visible-light photo-reduced thioacetalization process of aldehydes with thiols. | |
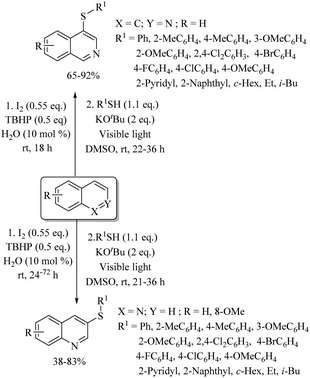 |
| Scheme 72 Visible-light-induced C–H thiolation of heteroarenes. | |
In 2017, Jiang and co-workers100 developed an interesting photochemical-condition-controlled protocol for the sulfenylation and sulfoxidation of diaryl iodonium salts with organic thiosulphate salts using eosin Y as the photocatalyst in the presence of DIPEA and Zn(OAc)2via tandem electron/energy-transfer and single-electron transfer, respectively (Scheme 73). When the C–S coupling was carried out using a 23 W CFL, it provided the resulting thioethers (Path A), whereas an 18 W green LED provided the sulfoxides (Path B). The developed protocol was further employed for late-stage sulfoxidation to access the pharmaceutically important metronidazole, furanose and pyranose derivatives. Furthermore, mechanistic study of these interesting transformations was also carried out by Jiang and co-workers101 using electron paramagnetic resonance experiments.
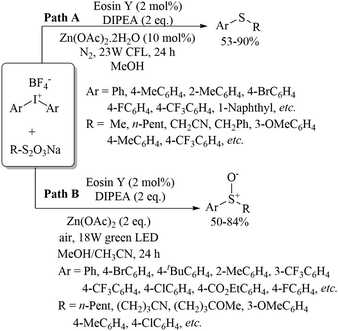 |
| Scheme 73 Photochemical-condition-controlled sulfenylation and sulfoxidation of diaryl iodonium salts. | |
5. Electricity-promoted C–S bond constructions
Long ago in 1974, Tabakovic and co-workers102 performed the electrochemical synthesis of 2-phenylbenzothiazole via intramolecular C–S bond formation on thiobenzanilide as shown in Scheme 74. In 1979, this group103 extended their electrochemical C–S bond-forming protocol to the preparation of 1,3-thiazole derivatives as shown in Scheme 74. In 2017, Qian et al.104 performed a TEMPO-catalyzed electrochemical intramolecular C–H thiolation process on thioamide derivatives using an RVC/Pt electrode in an undivided cell to obtain benzothiazoles and thiazolopyridines (Scheme 75). This work was an extension of the work of Tabakovic's group.102 Representative examples are shown.
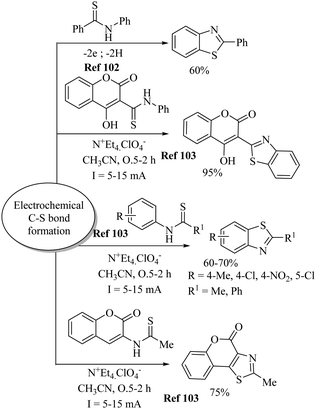 |
| Scheme 74 Electrochemical synthesis of 2-phenylbenzothiazole and 1,3-thiazole derivatives. | |
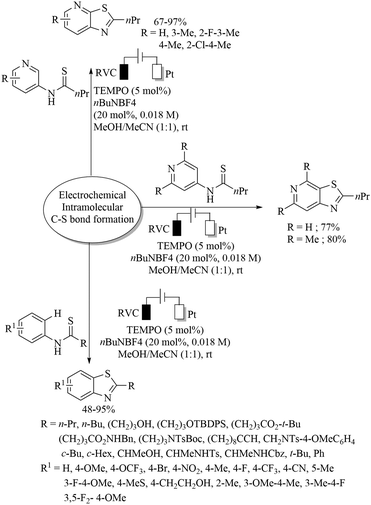 |
| Scheme 75 Electrochemical synthesis of benzothiazoles and thiazolopyridines. | |
Lei and co-workers105 reported an electrochemical oxidant-free dehydrogenative C–H/C–S cross-coupling reaction at the C-3 position of indoles using thiols as a sulfur surrogate and Pt as the electrodes in an undivided cell to provide the resulting indole thioethers in 36–99% yields (Scheme 76). This group105 also extended this electrochemical strategy for the C–H thiolation of the sp2 C–H bond of arenes with thiols. Representative examples are presented. Mei and co-workers106 also developed a nickel-catalyzed electrochemical organic synthesis of aryl thioethers via the thiolation of aryl/heteroaryl halides with thiols, as shown in Scheme 77. In this electrochemical organic synthesis, magnesium was used as the anode and nickel as the cathode. A variety of halides possessing electron-rich and electron-poor groups underwent thiolation with different thiols.
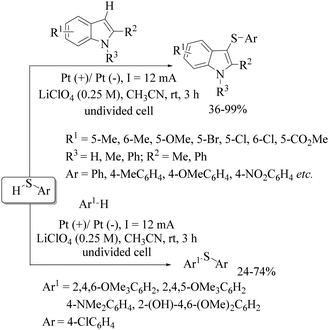 |
| Scheme 76 Electrochemical dehydrogenative C–H/C–S cross-coupling reactions between indoles and thiols. | |
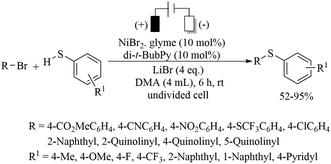 |
| Scheme 77 Nickel-catalysed electrochemical thiolation of aryl/heteroaryl halides with thiols. | |
Recently, Wu and co-workers107 also developed the electro-catalyzed C–H thiolation of the p-sp2 C–H bonds of aromatic ethers using thiols as thiolating agents and platinum electrodes in an undivided cell, as shown in Scheme 78.
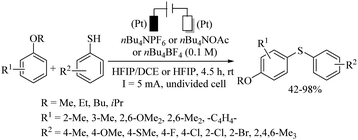 |
| Scheme 78 Electrochemical thiolation of the p-sp2 C–H bonds of aromatic ethers using thiols. | |
6. Conclusions
In conclusion, we have summarised the recent important developments in C–S bond formation in terms of various catalytic systems and substrates. A variety of metal-catalysed C–S cross-coupling protocols are discussed in detail by emphasising the original contributions along with the contributions of our group. Similarly, the utility of other sustainable catalytic methods such as oxidant-mediated, photo- and electro-catalysis for the construction of C–S bonds between a variety of substrates and sulphur surrogates are also discussed.
Conflicts of interest
There are no conflicts to declare.
Acknowledgements
This work was financially supported by the Ministry of Science and Technology, Taiwan (Most 110-2113-M-005-014-MY3), National Chung Hsing University, i-Center for Advanced Science and Technology (iCAST), the “Innovation and Development Center of Sustainable Agriculture (IDCSA)” from The Featured Areas Research Center Program within the framework of the Higher Education Sprout Project by the Ministry of Education (MOE) in Taiwan and Science and Engineering Research Board-Department of Science and Technology, Government of India, New Delhi, India (CRG/2020/003634).
Notes and references
- For reviews on C–S coupling reaction, see:
(a) C. C. Eichman and J. P. Stambuli, Molecules, 2011, 16, 590 CrossRef CAS;
(b) S. V. Ley and A. W. Thomas, Angew. Chem., Int. Ed., 2003, 42, 5400 CrossRef CAS;
(c) T. Kondo and T.-A. Mitsudo, Chem. Rev., 2000, 100, 3205 CrossRef CAS;
(d) I. P. Beletskaya and V. P. Ananikov, Chem. Rev., 2011, 111, 1596 CrossRef CAS PubMed;
(e) D. J. Procter, J. Chem. Soc., Perkin Trans. 1, 2001, 335 RSC;
(f) I. P. Beletskaya and V. P. Ananikov, Eur. J. Org. Chem., 2007, 3431 CrossRef CAS;
(g) H. Liu and X. Jiang, Chem. – Asian J., 2013, 8, 2546 CrossRef CAS;
(h) C.-F. Lee, R. S. Basha and S. S. Badsara, Top. Curr. Chem., 2018, 376, 25 CrossRef PubMed;
(i) C.-F. Lee, Y.-C. Liu and S. S. Badsara, Chem. – Asian J., 2014, 9, 706 CrossRef CAS;
(j) P. Annamalai, K.-C. Liu, S. S. Badsara and C.-F. Lee, Chem. Rec., 2021 DOI:10.1002/tcr.202100133.
-
(a) A. Dondoni, Angew. Chem., Int. Ed., 2008, 47, 8995 CrossRef CAS;
(b) A. B. Lowe, Polym. Chem., 2010, 1, 17 RSC;
(c) J. Liu, J. Yang, Q. Yang, G. Wang and Y. Li, Adv. Funct. Mater., 2005, 15, 1297 CrossRef CAS;
(d) X. Cao, L. Cao, W. Zhang, R. Lu, J.-S. Bian and X. Nie, Pharmacol. Ther., 2020, 216, 107687 CrossRef CAS;
(e) N. Wang, P. Saidhareddy and X. Jiang, Nat. Prod. Rep., 2020, 37, 246 RSC;
(f) J. Staunton and K. J. Weissman, Nat. Prod. Rep., 2001, 18, 380 RSC;
(g) J. Hutton, A. D. Jones, S. A. Lee, D. M. G. Martin, B. R. Meyrick, I. Patel, R. F. Peardon and L. Powell, Org. Process Res. Dev., 1997, 1, 61 CrossRef CAS;
(h) G. De Martino, M. C. Edler, G. La Regina, A. Coluccia, M. C. Barbera, D. Barrow, R. I. Nicholson, G. Chiosis, A. Brancale, E. Hamel, M. Artico and R. Silvestri, J. Med. Chem., 2006, 49, 947 CrossRef CAS;
(i) S. W. Kaldor, V. J. Kalish, J. F. Davies, B. V. Shetty, J. E. Fritz, K. Appelt, J. A. Burgess, K. M. Campanale, N. Y. Chirgadze, D. K. Clawson, B. A. Dressman, S. D. Hatch, D. A. Khalil, M. B. Kosa, P. P. Lubbehusen, M. A. Muesing, A. K. Patick, S. H. Reich, K. S. Su and J. H. Tatlock, J. Med. Chem., 1997, 40, 3979 CrossRef CAS PubMed;
(j) G. Liu, J. R. Huth, E. T. Olejniczak, R. Mendoza, P. DeVries, S. Leitza, E. B. Reilly, G. F. Okasinski, S. W. Fesik and T. W. von Geldern, J. Med. Chem., 2001, 44, 1202 CrossRef CAS PubMed;
(k) M. Feng, B. Tang, S.-H. Liang and X. Jiang, Curr. Top. Med. Chem., 2016, 16, 1200 CrossRef CAS.
-
(a) M. Kosugi, T. Shimizu and T. Migita, Chem. Lett., 1978, 13 CrossRef CAS;
(b) T. Migita, T. Shimizu, Y. Asami, J. Shiobara, Y. Kato and M. Kosugi, Bull. Chem. Soc. Jpn., 1980, 53, 1385 CrossRef CAS.
-
(a) N. Sundaravelu, S. Sangeetha and G. Sekar, Org. Biomol. Chem., 2021, 19, 1459 RSC;
(b) S. S. Badsara, C.-C. Cheng and C.-F. Lee, Asian J. Org. Chem., 2014, 3, 1197 CrossRef CAS.
-
(a) F. Zhao, Q. Tan, D. Wang and G.-J. Deng, Green Chem., 2020, 22, 427 RSC;
(b) Y.-Y. Jiang, S. Liang, C.-C. Zeng, L.-M. Hua and B.-G. Sun, Green Chem., 2016, 18, 6311 RSC.
-
(a) S. S. Badsara, P. Singh, R. Choudhary, R. Bai and M. C. Sharma, New J. Chem., 2019, 43, 11045 RSC;
(b) R. J. Reddy and A. H. Kumari, RSC Adv., 2021, 11, 9130 RSC;
(c) R. J. Reddy, A. H. Kumari and J. J. Kumar, Org. Biomol. Chem., 2021, 19, 3087 RSC;
(d) J.-H. Cheng, C. Ramesh, H.-L. Kao, Y.-J. Wang, C.-C. Chen and C.-F. Lee, J. Org. Chem., 2012, 77, 10369 CrossRef CAS PubMed;
(e) I. M. Yonova, C. A. Osborne, N. S. Morrissette and E. R. Jarvo, J. Org. Chem., 2014, 79, 1947 CrossRef CAS;
(f) M. Wang, Z. Dai and X. Jiang, Nat. Commun., 2019, 10, 2661 CrossRef.
- T. Itoh and T. Mase, Org. Lett., 2004, 6, 4587 CrossRef CAS.
- M. Murata and S. L. Buchwald, Tetrahedron, 2004, 60, 7397 CrossRef CAS.
- M. A. Fernandez-Rodrguez, Q. Shen and J. F. Hartwig, Chem. – Eur. J., 2006, 12, 7782 CrossRef.
- P. Anbarasan, H. Neumann and M. Beller, Chem. Commun., 2011, 47, 3233 RSC.
- M. Iwasaki, M. Iyanaga, Y. Tsuchiya, Y. Nishimura, W. Li, Z. Li and Y. Nishihara, Chem. – Eur. J., 2014, 20, 2459 CrossRef CAS.
- Z. Qiao, J. Wei and X. Jiang, Org. Lett., 2014, 16, 1212 CrossRef CAS PubMed.
- R. A. A. AL-Shuaeeb, G. Galvani, G. Bernadat, J.-D. Brion, M. Alami and S. Messaoudi, Org. Biomol. Chem., 2015, 13, 10904 RSC.
- M. Wang, Z. Qiao, J. Zhao and X. Jiang, Org. Lett., 2018, 20, 6193 CrossRef CAS.
- S. Chen, M. Wang and X. Jiang, Chin. J. Chem., 2018, 36, 921 CrossRef CAS.
- N. Ichiishi, C. A. Malapit, L. Wozniak and M. S. Sanford, Org. Lett., 2018, 20, 44 CrossRef CAS PubMed.
- H. J. Cristau, B. Chabaud, A. Chene and H. Christol, Synthesis, 1981, 892 CrossRef CAS.
- K. Takagi, Chem. Lett., 1987, 2221 CrossRef CAS.
- Y. Zhang, K. C. Ngeow and J. Y. Ying, Org. Lett., 2007, 9, 3495 CrossRef CAS PubMed.
- S.-Y. Yan, Y.-J. Liu, B. Liu, Y.-H. Liu, Z.-Z. Zhang and B.-F. Shi, Chem. Commun., 2015, 51, 7341 RSC.
- C. Lin, W. Yu, J. Yao, B. Wang, Z. Liu and Y. Zhang, Org. Lett., 2015, 17, 1340 CrossRef CAS PubMed.
- C. Liu and M. Szostak, Chem. Commun., 2018, 54, 2130 RSC.
- K. Ishitobi, R. Isshiki, K. K. Asahara, C. Lim, K. Muto and J. Yamaguchi, Chem. Lett., 2018, 47, 756 CrossRef CAS.
- T. Yamamoto and Y. Sekine, Can. J. Chem., 1984, 62, 1544 CrossRef CAS.
- F. Y. Kwong and S. L. Buchwald, Org. Lett., 2002, 4, 3517 CrossRef CAS.
- P. Naus, L. Leseticky, S. Smrcek, I. Tislerova and M. Sticha, Synlett, 2003, 2117 CrossRef CAS.
- C. Savarin, J. Srogl and L. S. Liebeskind, Org. Lett., 2002, 4, 4309 CrossRef CAS PubMed.
- J.-T. Yu, H. Guo, Y. Yi, H. Fei and Y. Jiang, Adv. Synth. Catal., 2014, 356, 749 CrossRef CAS.
- T.-T. Wang, F.-L. Yang and S.-K. Tian, Adv. Synth. Catal., 2015, 357, 928 CrossRef CAS.
- X. Chen, X.-S. Hao, C.-E. Goodhue and J.-Q. Yu, J. Am. Chem. Soc., 2006, 128, 6790 CrossRef CAS PubMed.
- C.-K. Chen, Y.-W. Chen, C.-H. Lin, H.-P. Lin and C.-F. Lee, Chem. Commun., 2010, 46, 282 RSC.
- H.-L. Kao and C.-F. Lee, Org. Lett., 2011, 13, 5204 CrossRef CAS.
- J.-H. Cheng, C.-L. Yi, T.-J. Liu and C.-F. Lee, Chem. Commun., 2012, 48, 8440 RSC.
- L.-H. Zou, D. L. Priebbenow, L. Wang, J. Mottweiler and C. Bolm, Adv. Synth. Catal., 2013, 355, 2558 CrossRef CAS.
- Y.-T. Huang, W.-T. Tsai, S. S. Badsara, C.-C. Chan and C.-F. Lee, J. Chin. Chem. Soc., 2014, 61, 967 CrossRef CAS.
- Y.-A. Chen, S. S. Badsara, W.-T. Tsai and C.-F. Lee, Synthesis, 2015, 181 Search PubMed.
- F.-J. Chen, G. Liao, X. Li, J. Wu and B.-F. Shi, Org. Lett., 2014, 16, 5644 CrossRef CAS.
- S. Sangeetha, P. Muthupandi and G. Sekar, Org. Lett., 2015, 17, 6006 CrossRef CAS PubMed.
- S. Sangeetha and G. Sekar, Chem. Commun., 2020, 56, 10906 RSC.
- A. Chabrier, A. Bruneau, S. Benmahdjoub, B. Benmerad, S. Belaid, J.-D. Brion, M. Alami and S. Messaoudi, Chem. – Eur. J., 2016, 22, 15006 CrossRef CAS.
- W.-C. Hsu, C.-E. Li and C.-F. Lee, Asian J. Org. Chem., 2017, 6, 1667 CrossRef CAS.
- C.-W. Chen, Y.-L. Chen, D. M. Reddy, K. Du, C.-E. Li, B.-H. Shih, Y.-J. Xue and C.-F. Lee, Chem. – Eur. J., 2017, 23, 10087 CrossRef CAS.
- Y. Liu, L. Y. Lam, J. Ye, N. Blanchard and C. Ma, Adv. Synth. Catal., 2020, 362, 2326 CrossRef CAS.
-
(a) C.-L. Yi, Y.-T. Huang and C.-F. Lee, Green Chem., 2013, 15, 2476 RSC;
(b) H.-S. Jhuang, Y.-W. Liu, D. M. Reddy, Y.-Z. Tzeng, W.-Y. Lin and C.-F. Lee, J. Chin. Chem. Soc., 2018, 65, 24 CrossRef CAS.
- W. Ali, S. Guin, S. K. Rout, A. Gogoi and B. K. Patel, Adv. Synth. Catal., 2014, 356, 3099 CrossRef CAS.
- X. Xiao, M. Feng and X. Jiang, Angew. Chem., Int. Ed., 2016, 55, 14121 CrossRef CAS.
- Y.-C. Wong, T. T. Jayanth and C.-H. Cheng, Org. Lett., 2006, 8, 5613 CrossRef CAS.
- Z.-B. Dong, M. Balkenhohl, E. Tan and P. Knochel, Org. Lett., 2018, 20, 7581 CrossRef CAS.
- A. Correa, M. Carril and C. Bolm, Angew. Chem., Int. Ed., 2008, 47, 2880 CrossRef CAS.
- J.-R. Wu, C.-H. Lin and C.-F. Lee, Chem. Commun., 2009, 4450 RSC.
- W.-Y. Wu, J.-C. Wang and F.-Y. Tsai, Green Chem., 2009, 11, 326 RSC.
- Y.-Y. Lin, Y.-J. Weng, C.-H. Lin, J.-H. Cheng and C.-F. Lee, J. Org. Chem., 2012, 77, 6100 CrossRef CAS.
- H. Wang, L. Wang, J. Shang, X. Li, H. Wang, J. Gui and A. Lei, Chem. Commun., 2012, 48, 76 RSC.
- S. Panja, P. Maity, D. Kundu and B. C. Ranu, Tetrahedron Lett., 2017, 58, 3441 CrossRef CAS.
- Y.-T. Huang, S.-Y. Lu, C.-L. Yi and C.-F. Lee, J. Org. Chem., 2014, 79, 4561 CrossRef CAS.
- M. Bandaru, N. M. Sabbavarpu, R. Katla and V. D. N. Yadavalli, Chem. Lett., 2010, 39, 1149 CrossRef CAS.
- T.-J. Liu, C.-L. Yi, C.-C. Chan and C.-F. Lee, Chem. – Asian J., 2013, 8, 1029 CrossRef CAS.
-
(a) M. Arisawa, T. Suzuki, T. Ishikawa and M. Yamaguchi, J. Am. Chem. Soc., 2008, 130, 12214 CrossRef CAS;
(b) M. Arisawa, Tetrahedron Lett., 2014, 55, 3391 CrossRef CAS;
(c) M. Arisawa, T. Ichikawa and M. Yamaguchi, Org. Lett., 2012, 14, 5318 CrossRef CAS;
(d) M. Arisawa, T. Yamada and M. Yamaguchi, Tetrahedron Lett., 2010, 51, 6090 CrossRef CAS;
(e) M. Arisawa, T. Ichikawa and M. Yamaguchi, Tetrahedron Lett., 2013, 54, 4327 CrossRef CAS.
- Y. Yang, W. Hou, L. Qin, J. Du, H. Feng, B. Zhou and Y. Li, Chem. – Eur. J., 2014, 20, 416 CrossRef CAS.
- X. Rui, C. Wang, D. Si, X. Hui, K. Li, H. Wen, W. Li and J. Liu, J. Org. Chem., 2021, 86, 6622 CrossRef CAS.
- S. Kathiravan, P. Anaspure, T. Zhang and I. A. Nicholls, Org. Lett., 2021, 23, 3331 CrossRef CAS PubMed.
- A. Mandal, S. Dana, H. Sahoo, G. S. Grandhi and M. Baidya, Org. Lett., 2017, 19, 2430 CrossRef CAS PubMed.
- R. Das and D. Chakraborty, Tetrahedron Lett., 2012, 53, 7023 CrossRef CAS.
- V. P. Reddy, K. Swapna, A. V. Kumar and K. R. Rao, J. Org. Chem., 2009, 74, 3189 CrossRef CAS PubMed.
- V. P. Reddy, A. V. Kumar, K. Swapna and K. R. Rao, Org. Lett., 2009, 11, 1697 CrossRef CAS.
- Y. Liao, P. Jiang, S. Chen, H. Qi and G.-J. Deng, Green Chem., 2013, 15, 3302 RSC.
- F.-L. Yang and S.-K. Tian, Angew. Chem., Int. Ed., 2013, 52, 4929 CrossRef CAS.
- D. Yang, K. Yan, W. Wei, J. Zhao, M. Zhang, X. Sheng, G. Li, S. Lu and H. Wang, J. Org. Chem., 2015, 80, 6083 CrossRef CAS.
- D. Yang, P. Sun, W. Wei, L. Meng, L. He, B. Fang, W. Jiang and H. Wang, Org. Chem. Front., 2016, 3, 1457 RSC.
- P. Ghosh, G. Chhetri and S. Das, RSC Adv., 2021, 11, 10258 RSC.
- W. Zhao and A. Zhou, ChemCatChem, 2015, 7, 3464 CrossRef CAS.
- B. Li, Z. Chen, H. Cao and H. Zhao, Org. Lett., 2018, 20, 3291 CrossRef CAS.
- K. Choudhuri, S. Maiti and P. Mal, Adv. Synth. Catal., 2019, 361, 1092 CrossRef CAS.
- J. Yuan, X. Ma, H. Yi, C. Liu and A. Lei, Chem. Commun., 2014, 50, 14386 RSC.
- S.-r. Guo, Y.-q. Yuan and J.-n. Xiang, Org. Lett., 2013, 15, 4654 CrossRef CAS PubMed.
- J. Zhao, H. Fang, J. Han, Y. Pan and G. Li, Adv. Synth. Catal., 2014, 356, 2719 CrossRef CAS.
- B. Du, B. Jin and P. Sun, Org. Lett., 2014, 16, 3032 CrossRef CAS PubMed.
- Y.-W. Liu, S. S. Badsara, Y.-C. Liu and C.-F. Lee, RSC Adv., 2015, 5, 44299 RSC.
- H. Cao, J. Yuan, C. Liu, X. Hu and A. Lei, RSC Adv., 2015, 5, 41493 RSC.
- Y. Jiang, J.-X. Zou, L.-T. Huang, X. Peng, J.-D. Deng, L.-Q. Zhu, Y.-H. Yang, Y.-Y. Feng, X.-Y. Zhang and Z. Wang, Org. Biomol. Chem., 2018, 16, 1641 RSC.
- R. Choudhary, R. Bai, P. Singh, M. C. Sharma and S. S. Badsara, Tetrahedron, 2017, 73, 4323 CrossRef CAS.
- P. Singh, R. Bai, R. Choudhary, M. C. Sharma and S. S. Badsara, RSC Adv., 2017, 7, 30594 RSC.
- R. S. Basha, C.-W. Chen, D. M. Reddy and C.-F. Lee, Chem. – Asian J., 2018, 13, 2475 CrossRef CAS.
- X. Zhu, Y. Shi, H. Mao, Y. Cheng and C. Zhu, Adv. Synth. Catal., 2013, 355, 3558 CrossRef CAS.
- J.-W. Zeng, Y.-C. Liu, P.-A. Hsieh, Y.-T. Huang, C.-L. Yi, S. S. Badsara and C.-F. Lee, Green Chem., 2014, 16, 2644 RSC.
- S. S. Badsara, Y.-C. Liu, P.-A. Hsieh, J.-W. Zeng, S.-Y. Lu, Y.-W. Liu and C.-F. Lee, Chem. Commun., 2014, 50, 11374 RSC.
- H. Nambu, K. Hata, M. Matsugi and Y. Kita, Chem. Commun., 2002, 1082 RSC.
- S. B. Bandgar, B. P. Bandgar, B. L. Korbad and S. S. Sawant, Tetrahedron Lett., 2007, 48, 1287 CrossRef CAS.
- S. Singh and L. D. S. Yadav, Tetrahedron Lett., 2012, 53, 5136 CrossRef CAS.
- J. Chung, U. R. Seo, S. Chun and Y. K. Chung, ChemCatChem, 2016, 8, 318 CrossRef CAS.
- C. Uyeda, Y. Tan, G. C. Fu and J. C. Peters, J. Am. Chem. Soc., 2013, 135, 9548 CrossRef CAS PubMed.
- M. Jiang, H. Li, H. Yang and H. Fu, Angew. Chem., Int. Ed., 2017, 56, 874 CrossRef CAS PubMed.
- B. Liu, C.-H. Lim and G. M. Miyake, J. Am. Chem. Soc., 2017, 139, 13616 CrossRef CAS PubMed.
- M. Majek and A. J. V. Wangelin, Chem. Commun., 2013, 49, 5507 RSC.
- G. Kibriya, S. Mondal and A. Hajra, Org. Lett., 2018, 20, 7740 CrossRef CAS PubMed.
- Y.-C. Shieh, K. Du, R. S. Basha, Y.-J. Xue, B.-H. Shih, L. Li and C.-F. Lee, J. Org. Chem., 2019, 84, 6223 CrossRef CAS PubMed.
- K. Du, S.-C. Wang, R. S. Basha and C.-F. Lee, Adv. Synth. Catal., 2018, 361, 1597 CrossRef.
- Y.-C. Liu, D. M. Reddy, X.-A. Chen, Y.-C. Shieh and C.-F. Lee, Eur. J. Org. Chem., 2020, 2542 CrossRef CAS.
- A. Nandy, I. Kazi, S. Guha and G. Sekar, J. Org. Chem., 2021, 86, 2570 CrossRef CAS PubMed.
- Y. Li, M. Wang and X. Jiang, ACS Catal., 2017, 7, 7587 CrossRef CAS.
- Y. Li, W. Xie and X. Jiang, Chem. – Eur. J., 2015, 21, 16059 CrossRef CAS PubMed.
- M. Lacan, K. Jakopcic, V. Rogic, S. Damoni, O. Rogic and I. Tabakovic, Synth. Commun., 1974, 4, 219 CrossRef CAS.
- I. Tabakovic, M. Trkovnik, M. Batusic and K. Tabakovic, Synthesis, 1979, 590 CrossRef CAS.
- X.-Y. Qian, S.-Q. Li, J. Song and H.-C. Xu, ACS Catal., 2017, 7, 2730 CrossRef CAS.
- P. Wang, S. Tang, P. Huang and A. Lei, Angew. Chem., Int. Ed., 2017, 56, 3009 CrossRef CAS PubMed.
- D. Liu, H.-X. Ma, P. Fang and T.-S. Mei, Angew. Chem., Int. Ed., 2019, 58, 5033 CrossRef CAS PubMed.
- J.-H. Wang, T. Lei, H.-L. Wu, X.-L. Nan, X.-B. Li, B. Chen, C.-H. Tung and L.-Z. Wu, Org. Lett., 2020, 22, 3804 CrossRef CAS PubMed.
Footnote |
† These authors contributed equally to this work. |
|
This journal is © The Royal Society of Chemistry and the Centre National de la Recherche Scientifique 2022 |
Click here to see how this site uses Cookies. View our privacy policy here.