DOI:
10.1039/D1PY01489G
(Paper)
Polym. Chem., 2022,
13, 80-84
Facile synthesis of PEGylated dendritic polyurethane as unimolecular micelles for ultrasound-triggered localized drug delivery†
Received
5th November 2021
, Accepted 18th November 2021
First published on 23rd November 2021
Abstract
The ultrasound-mediated approach has attracted attention for enhanced localized drug delivery in tumor chemotherapy. Here, well-defined PEGylated dendritic polyurethane (Ph-DPU-PEG) was synthesized as unimolecular micelles with a hydrophobic core via a facile one-pot method for ultrasound-triggered localized drug delivery. The chemotherapeutic drug could be encapsulated in the hydrophobic cores, and the drug-loaded micelles (DOX@Ph-DPU-PEG) were obtained as nanomedicine, which would hardly release the drug without ultrasound, regardless of the acidity of the releasing media. However, they showed an excellent ultrasound-triggered intracellular drug release performance, with a cumulative drug release of 81.6% in the simulated tumor intracellular microenvironment within 12 h. Furthermore, the ultrasound-triggered intracellular drug release was revealed with the CLSM technique and an enhanced tumor growth inhibition was achieved on the HepG2 cells compared to free DOX.
1. Introduction
Polymer micelles have been wisely investigated as drug carriers for tumor chemotherapy in the last decades,1 owing to their unique diameter and distribution. However, conventional multimolecular micelles, which are fabricated via the self-assembly of copolymers with their hydrophobic blocks as the cores and the hydrophilic ones as the shell, are usually not so stable and will re-assemble upon dilution due to the critical micelle concentration (CMC). The re-assembly would cause drug leakage before accumulation in the tumor sites.2 To be specific, the loaded drug could be released during the blood circulation after intravenous injection, resulting in severe toxic side effects on normal cells and tissues.
Recently, unimolecular micelles have attracted intense interests as drug carriers, because of their superior stability in comparison with conventional multimolecular micelles.3 By now, various unimolecular micelles with unique spherical shapes have been reported as DDSs for tumor treatment, mainly with dendritic polymers4–11 or hyperbranched polymers12–14 containing a hydrophobic core and hydrophilic brushes in their topological structure. Moreover, the cavities in the hydrophobic cores provide space to load hydrophobic chemotherapeutics.
For unimolecular micelles with lower hydrophobic cores or weaker interaction between the drugs and the cores, the loaded hydrophobic chemotherapeutic drugs easily leaked into the normal physiological medium, up to more than 50%
12,13 and even 80%.4,14 To enhance chemotherapy efficacy and minimize anticancer drug side effects, intramolecular crosslinked dendritic polymer-based unimolecular micelles have also been reported.15
It is easy to hypothesize that the dendritic polymer-based unimolecular micelles with higher hydrophobic cores or stronger interaction with the loaded drugs could efficiently avoid drug leakage. Moreover, drug release into the targeted media should also be suppressed. Fortunately, ultrasound has been well recognized as a powerful approach to trigger or activate the drug release by means of ultrasound cavitation, producing a significant response in the pores of the carrier to achieve an accelerated diffusion from the DDSs.16 So excellent ultrasound-triggered drug release is expected with the suitable dendritic polymer-based unimolecular micelles as the carrier, which could not release the drug without ultrasound.
Based on the above hypothesis, well-defined PEGylated dendritic polyurethane (Ph-DPU-PEG) was synthesized in the present work via a facile one-pot method, based on the high-performance reaction of the isocyanate group (Scheme 1). The unimolecular micelles could be easily formed to load DOX via hydrophobic interactions and hydrogen bonds, minimizing the drug leakage without ultrasound and achieving an enhanced localized drug release with ultrasound.
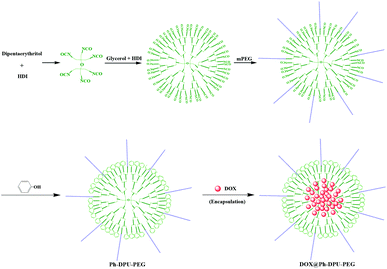 |
| Scheme 1 Fabrication of Ph-DPU-PEG unimolecular micelles and DOX@Ph-DPU-PEG. | |
2. Experimental
2.1 Materials and reagents
Polyethylene glycol monomethyl ether (mPEG, Mn = 2000, 99%) was obtained from Shanghai Jinpan Biotech Co. Ltd. Dipentaerythritol (90%), phenol (99%) and hexamethylene diisocyanate (HDI, 99%) were purchased from Aladdin Chem. Co., Ltd. Doxorubicin hydrochloride (DOX·HCl, 99.4%) was obtained from Beijing Huafeng United Technol. Co., Ltd. Glycerol (99.5%) was obtained from Sinopharm Chemical Reagent Co. Ltd. Other reagents were all analytical grade and used directly without any treatment. Double distilled water was used throughout.
2.2 Synthesis of PEGylated dendritic polyurethane (Ph-DPU-PEG)
The functionalized dendritic polyurethane was synthesized with dipentaerythritol as the core via a facile one-pot method. Typically, 0.125 mmol dipentaerythritol was dissolved in 60 mL DMF, then 0.75 mmol HDI was added and the solution was stirred at 85 °C for 3 h. After cooling to 50 °C, 5.25 mmol glycerol was added and 10.5 mmol HDI was added after 15 min, following with stirring at 85 °C for 4 h. After that, the solution was cooled to 50 °C, and 1.2 mmol mPEG (20% of the theoretical –NCO molar number) was added and stirred at 80 °C for 3 h for the PEGylation of the dendritic polyurethane (DPU).
Finally, the terminal isocyanate groups of the DPU-PEG were modified with phenol as follows: 5.4 mmol phenol was added (1.2 times of the theoretical –NCO molar number) into the above DPU-PEG solution and stirred at 80 °C for 5 h to transform the residual-NCO groups to phenyl groups. The resultant Ph-DPU-PEG was obtained after precipitating with ethyl ether and drying.
2.3 Drug loading
DOX was then loaded in the Ph-DPU-PEG with different capacities as follows: 50.0 mg Ph-HPU-PEG was dissolved in 50 mL DMF, then 18.0 mg or 50.0 mg of DOX·HCl and 40 μL or 100 μL triethylamine were added and the mixture was stirred at room temperature in the dark for 24 h. The resultant DOX-loaded dendritic polyurethane (DOX@Ph-DPU-PEG-I or DOX@Ph-DPU-PEG-II) was collected by dialyzing the reaction solution against water for 24 h (3.5 kDa), and finally lyophilization.
2.4
In vitro drug release
The in vitro DOX release from the DOX-loaded micelles was conducted in two media: pH 7.4 PBS mimicking the normal physiological medium and pH 5.0 ABS mimicking the tumor intracellular microenvironment. In brief, 10.0 mL of dispersion in buffer solution containing the proposed DDSs (4.0 mg) was dialyzed in 110.0 mL of the corresponding buffer solution (1.0 kDa) in an IS-RSD3 incubation shaker at 37 °C. The dialysate (5.0 mL) was taken out for the DOX concentration measurement with TU-1901 UV–vis spectrometry, while 5.0 mL fresh corresponding buffer solution was added to make the volume constant.
To verify the ultrasound responsiveness, the drug release from the proposed DOX-loaded micelles was also conducted at 37 °C with a low frequency ultrasound (40 kHz, 0.45 W cm−2).
2.5
In vitro cellular experiments
The HepG2 cells, purchased from the National Collection of Authenticated Cell Cultures (Shanghai, China), were seeded into 96-well plates at a density of 1 × 105 cells/well and incubated in a humid atmosphere (5% CO2) at 37 °C for 12 h. The cellular uptake and subcellular distribution were assessed on a DMI 4000B confocal laser scanning microscope CLSM. The 100.0 μL aqueous dispersion of the DOX-loaded micelles (DOX equivalent concentration was 15 μg mL−1) was added into the cultivated HepG2 cells, followed by an incubation of 12 h. Then the cells were incubated with or without ultrasound irradiation (40 kHz, 0.45 W cm−2) for 6 h around 37 °C and then cultivated for another 12 h. After washing with the culture solution, the cells were dyed with DAPI and fixed with 4% paraformaldehyde, and the inverted fluorescence microscope (Olympus, IX 71) was used to observe the fluorescence distribution of different colors in the cells.
The in vitro cytotoxicity was assessed with the MTT assays as follows: after the HepG2 cells were cultivated for 12 h, blank Ph-DPU-PEG, DOX@Ph-DPU-PEG-I micelles or free DOX was added separately at different concentrations. After further incubation at 37 °C for 12 h, the cells were incubated with or without ultrasound irradiation (40 kHz, 0.45 W cm−2) for 6 h around 37 °C and then cultivated for another 12 h. Then, 20 μL (5 mg mL−1) of MTT solution was added to each well for an incubation of 4 h. The MTT solution in the hole was sucked out and washed with PBS 3 times. Finally, the absorbance of the solution was evaluated at 490 nm with a microplate analyzer to detect the cell viability.
2.6 Characterization
1H NMR analysis was conducted on a JEOL 400 MHz NMR spectrometer with DMSO-d6 as the solvent. BI-200SM dynamic light scattering was used to measure the hydrodynamic diameter and distribution of the micelles at 25 °C. The morphology of the samples was characterized on a JEM-1200EX transmission electron microscope (TEM). DOX concentration was measured on a UV/vis Spectrometer (TU-1901) at 480 nm at room temperature.
3. Results and discussion
3.1 Synthesis and characterization of PEGylated dendritic polyurethane (Ph-DPU-PEG)
Owing to the perfect 3-D spherical topology, dendritic polymer-based unimolecular micelles show a better drug encapsulation performance than hyperbranched polymer-based ones. However, the synthesis procedure for dendritic polymers is usually tedious in comparison with hyperbranched polymers. Here, a facile one-pot method was established via the high-performance reaction of the isocyanate group. By adding the qualitative monomers (HDI and glycerol) for a G3.0 dendrimer in one pot with dipentaerythritol as the core (Scheme 1), dendritic polyurethane (DPU) was obtained with surface isocyanate groups. To eliminate the effect of the end-groups17 and introducing hydrophilic blocks, the surface isocyanate groups were modified with phenol (80%) and mPEG (20%). After precipitation, PEGylated dendritic polyurethane (Ph-DPU-PEG) with inert hydrophobic surface end-groups and hydrophilic PEG brushes was obtained.
In the 1H NMR spectrum (Fig. 1), the characteristic signals of the protons a, b and c in the inert end-groups, the proton d in the carbamate bond and the protons e in PEG could be seen. The integral area ratio of the protons (a
:
d
:
e) was 1
:
4.42
:
43.53, near to the theoretical value of 1
:
4.68
:
44 for perfect PEGylated dendritic polyurethane, indicating the successful synthesis of the well-defined functionalized G3.0 dendritic polymer Ph-DPU-PEG, via the facile one-pot approach.
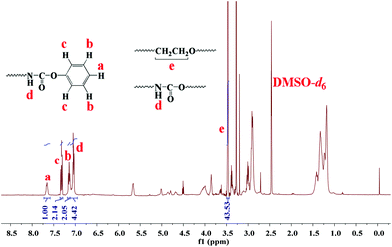 |
| Fig. 1
1H NMR spectrum of PEGylated dendritic polyurethane (Ph-DPU-PEG). | |
3.2 Ph-DPU-PEG unimolecular micelles and drug loading
The lyophilized Ph-DPU-PEG could be easily dispersed into water. Spherical nanoparticles around 10 nm could be seen in the TEM image (Fig. 2a). They showed the mean hydrodynamic diameter (Dh) of 26.2 nm in the good solvent DMF and 17.5 nm in the bad solvent water (Fig. 3a and b) in the DLS analysis. The Dh was bigger in DMF than that in water, which resulted from the swelling of the hydrophobic dendritic polyurethane as the core.18 A similar hydrodynamic diameter and distribution was achieved with Ph-DPU-PEG in water with a concentration of 0.0005–0.1 mg mL−1 (Fig. S1†), indicating the formation of unimolecular micelles.19 Their hydrophobic cores are expected for loading the hydrophobic chemotherapeutic drug with minimal drug leakage.
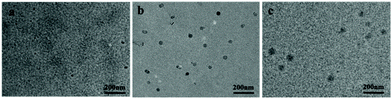 |
| Fig. 2 TEM images of Ph-DPU-PEG (a), DOX@Ph-DPU-PEG-I (b) and DOX@Ph-DPU-PEG-II (c). | |
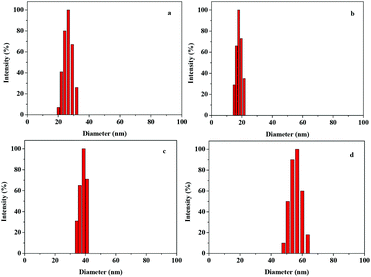 |
| Fig. 3 Hydrodynamic diameters and distributions of Ph-DPU-PEG in DMF (a), Ph-DPU-PEG (b), DOX@Ph-DPU-PEG-I (c) and DOX@Ph-DPU-PEG-II (d) in water. | |
Then DOX was encapsulated into the Ph-DPU-PEG unimolecular micelles. After DOX-loading, the DOX-loaded micelles maintained a spherical shape (Fig. 2b and c) and the particle size increased to 25 nm and 40 nm for the DOX@Ph-DPU-PEG-I and DOX@Ph-DPU-PEG-II with a drug-loading capacity (DLC) of 18.3% and 35.3%, respectively. Their Dh increased to 37.3 nm and 55.6 nm, favoring the passive targeting via the EPR effect.
3.3
In vitro drug release
The drug release property of the DOX@Ph-DPU-PEG was then investigated in pH 7.4 PBS and pH 5.0 ABS, mimicking the normal physiological medium and the tumor intracellular microenvironment, respectively. As shown in Fig. 4a, a very low drug release could be found at pH 7.4 (4.5%) and pH 5.0 (4.7%) from DOX@Ph-DPU-PEG-I without ultrasound. As for the DOX@Ph-DPU-PEG-II with a higher DOX-loading capacity, the drug leakage and release increased to 6.8% and 16.4% at pH 7.4 and pH 5.0, respectively, showing a pH-responsive drug release due to the protonation of the amino group in DOX, which would enhance its solubility.
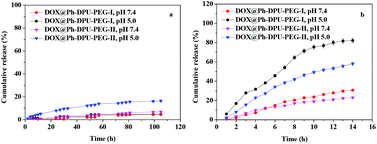 |
| Fig. 4
In vitro drug release profiles from the DOX@Ph-DPU-PEG-I and DOX@Ph-DPU-PEG-II without (a) and with ultrasound (b) (n = 3). | |
Comparing the drug release from the two DOX@Ph-DPU-PEG without ultrasound, it could be concluded that DOX was mainly encapsulated in the inner cavities of the hydrophobic DPU cores in DOX@Ph-DPU-PEG-I with a lower DLC, while the DOX molecules might be encapsulated in the inner cavities and embedded in the skin layer of the hydrophobic DPU cores in the DOX@Ph-DPU-PEG-II with a higher DLC. The latter caused drug leakage and release in the simulated media.
As for the ultrasound-mediated drug release profiles, DOX@Ph-DPU-PEG-I showed higher drug leakage and drug release than DOX@Ph-DPU-PEG-II because of the lower DOX dose. The cumulative drug release reached 80.1% and 53.2% for DOX@Ph-DPU-PEG-I and DOX@Ph-DPU-PEG-II at pH 5.0 within 12 h, with a drug leakage of 27.9% and 20.9%, respectively. The results demonstrated that the drugs loaded in the Ph-DPU-PEG unimolecular micelles could be released via ultrasound-activation,16 no matter where they were loaded, whether in the inner cavities or the skin layer of the hydrophobic DPU cores.
The drug release profiles were fitted with the Higuchi and Korsmeyer–Peppas models to explain the drug release mechanism (Fig. S2†). As shown in Table 1, all the correlation coefficients obtained with the Higuchi model were higher than those with the Korsmeyer–Peppas model, indicating the drug release should be controlled by diffusion.20 Furthermore, the k values with ultrasound were significantly higher than those without ultrasound, demonstrating that ultrasound irradiation could efficiently promote the diffusion.
Table 1 Fitted parameters with the Higuchi and Korsmeyer–Peppas models
Material |
Condition |
Releasing media |
Higuchi |
Korsmeyer–Peppas |
R
2
|
k
|
R
2
|
n
|
DOX@Ph-DPU-PEG-I |
Non-ultrasound |
pH 7.4 |
0.8778 |
0.0931 |
0.7667 |
1.0510 |
pH 5.0 |
0.8255 |
0.1030 |
0.8543 |
0.6462 |
Ultrasound |
pH 7.4 |
0.9835 |
1.5820 |
0.9324 |
1.7412 |
pH 5.0 |
0.9841 |
4.0472 |
— |
— |
DOX@Ph-DPU-PEG-II |
Non-ultrasound |
pH 7.4 |
0.9935 |
0.1105 |
0.9382 |
1.3140 |
pH 5.0 |
0.9869 |
0.2365 |
0.9713 |
0.6648 |
Ultrasound |
pH 7.4 |
0.9929 |
1.0647 |
0.9230 |
1.1343 |
pH 5.0 |
0.9926 |
2.7745 |
0.9394 |
1.1795 |
Overall, the encapsulated DOX was hardly released from the DOX@Ph-DPU-PEG-I without ultrasound but could be released in a sustained mode in the simulated tumor intracellular microenvironment with ultrasound-activation. So, the DOX@Ph-DPU-PEG-I is expected to be an ideal ultrasound-mediated DDS for located drug release with minimal drug leakage.
3.4
In vitro cellular uptake and cytotoxicity
The CLSM technique was used to reveal the uptake of the DOX@Ph-DPU-PEG-I. After incubating human liver cancer cells (HepG2) with DOX@Ph-DPU-PEG-I for 30 h without ultrasound, the red fluorescence of DOX could be seen in the cytosol (Fig. 5). Interestingly, the red fluorescence of DOX could be seen in both the cytosol and nuclei after incubation for 30 h with a middle 6 h of ultrasound irradiation. Such phenomena revealed that the DOX@Ph-DPU-PEG-I could be internalized into the HepG2 cells, regardless of ultrasound. However, the encapsulated DOX could only be released inside the tumor cells with ultrasound irradiation.
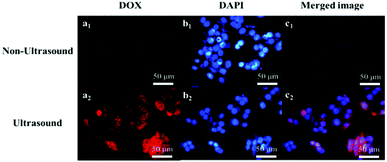 |
| Fig. 5 CLSM images of HepG2 cells after incubation with DOX@Ph-DPU-PEG-I. The labels a and b represent the images of the channels of DOX and DAPI, c is the merged images of a with b. | |
To further reveal the ultrasound-mediated located drug release from the DOX@Ph-DPU-PEG-I, the cytotoxicity on the HepG2 cells was assessed with MTT assays. Clearly, the blank carrier, Ph-DPU-PEG unimolecular micelles, did not show obvious cytotoxicity on the HepG2 cells with a cell viability of 99% and 95% without or with ultrasound at a dose of 20 μg mL−1 (Fig. 6a). The results also indicated that the ultrasound condition used in the present work caused no obvious damage to the HepG2 cells.
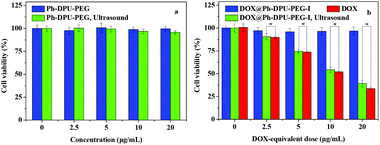 |
| Fig. 6
In vitro cytotoxicity of the Ph-DPU-PEG (a), DOX@Ph-DPU-PEG-I and DOX (b). The statistical significance was set at *p < 0.05. | |
As for the DOX@Ph-DPU-PEG-I, the cell viability could be retained at 97% at a DOX-equivalent dose of 20 μg mL−1 (Fig. 6b), meaning it had no cytotoxicity on the tumor cells without ultrasound. With the ultrasound-activation, the DOX@Ph-DPU-PEG-I showed an obvious dose-dependent cytotoxicity similar to the free DOX. The IC50 was calculated as 6.80 and 7.87 μg mL−1 for the ultrasound-mediated DOX@Ph-DPU-PEG-I and the free DOX, respectively, meaning an enhanced tumor growth inhibition of the proposed DDS compared to the free DOX, owing to the ultrasound-mediated sustained DOX release from the DOX@Ph-DPU-PEG-I, which could overcome the P-glycoprotein-mediated efflux.21
4. Conclusions
In summary, ultrasound-triggered localized drug delivery was developed with well-defined PEGylated dendritic polyurethane-based unimolecular micelles as the carrier, which was synthesized via a facile one-pot approach. Owing to the perfect spherical topology and high hydrophobicity of the DPU cores, as well as the strong interaction with DOX, the premature drug leakage could be efficiently avoided when the drug was encapsulated into the inner cavities of the hydrophobic DPU cores. After internalization into the tumor cells, the encapsulated DOX could be quickly released with ultrasound activation, showing an enhanced tumor growth inhibition compared to free DOX, while no obvious cytotoxicity was observed without ultrasound-activation. The ultrasound-triggered localized drug delivery strategy is expected to be a promising candidate for precise site-oriented drug delivery in future tumor treatment, with enhanced antitumor efficacy but minimal toxic side effects on normal cells and tissues.
Conflicts of interest
There are no conflicts to declare.
References
- N. Majumder, N. G. Das and S. K. Das, Ther. Delivery, 2020, 11, 613 CrossRef CAS.
- X. Fan, Z. Li and X. J. Loh, Polym. Chem., 2016, 7, 5898 RSC.
- A. Kavand, N. Anton, T. Vandamme and C. A. Serra, J. Controlled Release, 2020, 321, 285 CrossRef CAS PubMed.
- X. J. Li, Y. F. Qian, T. Liu, X. L. Hu, G. Y. Zhang, Y. Z. You and S. Y. Liu, Biomaterials, 2011, 32, 6595 CrossRef CAS.
- S. Zhang, J. B. Xu, H. Chen, Z. F. Song, Y. L. Wu and J. Kong, Macromol. Biosci., 2017, 17, 1600258 CrossRef.
- G. Liu, H. J. Gao, Y. X. Zuo, X. W. Zeng, W. Tao, H. I. Tsai and L. Mei, ACS Appl. Mater. Interfaces, 2017, 9, 112 CrossRef CAS PubMed.
- X. S. Fan, W. W. Zhang, Z. G. Hu and Z. B. Li, J. Mater. Chem. B, 2017, 5, 1062 RSC.
- R. Jaskula-Sztul, G. J. Chen, A. Dammalapati, A. Harrison, W. P. Tang, S. Q. Gong and H. Chen, J. Mater. Chem. B, 2017, 5, 151 RSC.
- J. Yang, W. F. Lu, J. L. Xiao, Q. Zong, H. X. Xu, Y. H. Yin, H. Hong and W. J. Xu, Acta Biomater., 2018, 79, 306 CrossRef CAS.
- W. W. Liu, J. J. Li, Z. H. Qin, M. M. Yao, X. L. Tian, Z. M. Zhang, L. Zhang, Q. Guo, L. H. Zhang, D. W. Zhu and F. L. Yao, Langmuir, 2020, 36, 3356 CrossRef CAS.
- L. Zhang, D. J. Shi, Y. Y. Gao, T. Y. Zhou and M. Q. Chen, Polym. Chem., 2020, 11, 2252 RSC.
- W. J. Xu, J. F. Burke, S. Pilla, H. Chen, R. Jaskula-Sztul and S. Q. Gong, Nanoscale, 2013, 5, 9924 RSC.
- W. J. Xu, I. A. Siddiqui, M. Nihal, S. Pilla, K. Rosenthal, H. Mukhtar and S. Q. Gong, Biomaterials, 2013, 34, 5244 CrossRef CAS.
- S. J. T. Rezaei, H. S. Abandansari, M. R. Nabid and H. Niknejad, J. Colloid Interface Sci., 2014, 425, 27 CrossRef PubMed.
- H. S. Abandansari, M. Abuali, M. R. Nabid and H. Niknejad, Polymer, 2017, 116, 16 CrossRef CAS.
- A. Ahmadi, S. Hosseini-Nami, Z. Abed, J. Beik, L. Aranda-Lara, H. Samadian, E. Morales-Avila, M. Jaymand and A. Shakeri-Zadeh, Drug Discovery Today, 2020, 25, 2182 CrossRef CAS.
- M. G. Zhang, R. Guo, M. Keri, I. Banyai, Y. Zhang, M. Cao, X. Y. Cao and X. Y. Shi, J. Phys. Chem. B, 2014, 118, 1696 CrossRef CAS PubMed.
- H. H. Li, H. T. Zhao, L. Yao, L. F. Zhang, Z. P. Cheng and X. L. Zhu, Polym. Chem., 2021, 12, 2335 RSC.
- Y. Wang, L. N. Li, J. J. Li, B. G. Yang, C. Y. Wang, W. C. Fang, F. Ji, Y. Wen and F. L. Yao, RSC Adv., 2016, 6, 17728 RSC.
- F. Raza, Y. Zhu, L. Chen, X. R. You, J. Y. Zhang, A. Khan, M. W. Khan, M. Hasnat, H. Zafar, J. Wu and L. Ge, Biomater. Sci., 2019, 7, 2023 RSC.
- Y. M. Dong, P. C. Du, M. L. Pei and P. Liu, J. Mater. Chem. B, 2019, 7, 5640 RSC.
Footnote |
† Electronic supplementary information (ESI) available. See DOI: 10.1039/d1py01489g |
|
This journal is © The Royal Society of Chemistry 2022 |
Click here to see how this site uses Cookies. View our privacy policy here.