DOI:
10.1039/D1QI01203G
(Research Article)
Inorg. Chem. Front., 2022,
9, 155-164
Ion cross-linking assisted synthesis of ZIF-8/chitosan/melamine sponge with anti-biofouling activity for enhanced uranium recovery†
Received
22nd September 2021
, Accepted 8th November 2021
First published on 9th November 2021
Abstract
Micro/mesoporous powder structures and biofouling of marine microorganisms directly limit the potential of zeolite frameworks in uranium recovery from seawater. Herein, an anti-biofouling zeolite framework-8/chitosan/melamine sponge adsorbent with a three-dimensional porous structure was prepared successfully using zinc ions and chitosan as adjuvants to promote the growth of zeolite framework-8 on the surface of melamine sponge. The mechanical and anti-biofouling properties of the sponge were determined by compression and algae tests. Relative to the zeolite framework-8 powders, the zeolite framework-8/chitosan/melamine sponge exhibited superior mechanical and anti-biofouling properties with 0.565 MPa of compressive stress and a 35% algae cell death rate. The adsorption capacities of the zeolite framework-8/chitosan/melamine sponge were 129.90 mg g−1 at pH 8.0 and 95.47 mg g−1 at the fifth adsorption–desorption cycle, which were higher than that of ZIF-8 powder (91.45 mg g−1). Furthermore, the excellent low-concentration U(VI) adsorption performance of zeolite framework-8/chitosan/melamine in natural seawater (Bohai) also confirmed its practical application potentiality.
Introduction
Nuclear power as clean energy to replace traditional fossil fuels has gained massive attention in recent decades.1 Uranium is a crucial strategic resource to ensure the developmental demand for the nuclear power industry, but terrestrial uranium resources are scarce and difficult to supply the long-term global consumption of nuclear power.2 Fortunately, potential uranium reserves in seawater are more than 4.5 billion tons, which is nearly a thousand times as many as terrestrial reservations.3–5 At present, the adsorption technique has been regarded as the most potential method to separate uranium from seawater due to its economy and universality.6,7 However, the extremely low concentration of uranium and the abundant competitive ions seriously hinder the highly effective extraction of uranium from seawater.8 Therefore, many advanced uranium adsorbents have been designed, such as inorganic materials, organic/polymeric materials, porous materials, protein-based materials, and nanostructure materials.9–14
Among these, metal–organic frameworks (MOFs) with a large surface area and ordered and tunable pore structures have aroused the attention of many researchers.15 In particular, zeolite frameworks (ZIFs), as the sub-category of MOFs, have become promising uranium adsorbent candidates, benefiting from their easy preparation, high porosities, large specific surface areas, abundant active sites and biocompatibility.16–19 Unfortunately, traditional ZIF micro/mesoporous powder structures have become a significant obstacle for recovery in actual applications.
Melamine sponge as a structural support has received extensive attention from researchers in various fields because of its unique three-dimensional porous structure, high tensile strength and excellent flexibility.20–22 Wang et al. prepared a poly(imide dioxime)/alginate composite sponge adsorbent with excellent uranium adsorption performance and sufficient mechanical strength using melamine sponge as a structural support.23 Thus, integrating ZIFs into melamine sponge with a more hierarchical and macroscopic 3D structure becomes an effective method to enhance the potential application of ZIF-based materials.24 Nevertheless, the chemical inertness of melamine sponge makes it difficult to meet the requirements of the nucleation and growth of ZIF materials on the surface. In addition, biofouling is also a factor limiting the performance of the adsorbent in natural seawater. Biofouling, caused by unfavourable adhesion of bacteria, algae or protozoa on the surface of a material, could damage the adsorbent structure and substantially reduce the uranium uptake capacity of the material.25,26
Generally, to overcome the chemical inertness of melamine sponge, carbonization and bioinspired methods have been developed to promote the nucleation and growth of nanoparticles on the sponge. Kim et al. designed a ZIF/carbon nitride foam via the fast carbonization of melamine foam.27 Lei et al. prepared a ZIF-8 coated melamine sponge using a solvothermal method with bioinspired polydopamine.28 However, few studies focused on the integration of antifouling and adsorption properties by ZIF sponge composites.
Herein, we constructed a ZIF-8/chitosan/melamine (CMZ8) sponge for uranium adsorption using chitosan and zinc ions as adjuvants to achieve the integration of anti-fouling, adsorption and separation properties. Chitosan (CS) is a natural, non-toxic, biocompatible polymer, has efficient antimicrobial ability and is regarded as an environmentally friendly antifoulant in various fields.29 Importantly, chitosan is a highly effective adsorbent, owing to the abundant –OH and –NH2 groups that supply more cross-linking active sites and promote the chelation to U(VI).30 Moreover, different from the traditional carbonization and bioinspired polydopamine for the sponge fabrication, we further selected zinc ions as auxiliary agents to accelerate the cross-linking of chitosan and overcome the sponge chemical inertness. Zinc ions can also be used as anchor points to induce the growth of ZIF-8 on the melamine sponge. Then, the characterization of the CMZ8 sponge was performed by scanning electron microscopy (SEM), energy-dispersive X-ray spectroscopy (EDS), X-ray diffraction (XRD) and Fourier-transform infrared spectroscopy (FTIR). A series of compression, anti-biofouling and adsorption tests were carried out to evaluate the mechanical properties, algae inhibitory effect and U(VI) adsorption performance of the CMZ8 sponge. Subsequently, we combined zeta potential, FTIR and X-ray photoelectron spectroscopy (XPS) with adsorption model fitting analysis to explore the adsorption mechanism of the CMZ8 sponge; furthermore, the cyclic adsorption and low-concentration adsorption performance in seawater (Bohai, China) were also investigated to inspect its actual potential application.
Experimental
Synthesis of ZIF-8 nanoparticles, CMZn and CMZ8 sponges
The commercial melamine sponge (MS, 2 cm × 2 cm × 1 cm) was washed with deionized water and ethanol solution before use. CS (0.3 g) and Zn(NO3)2·6H2O (4 mmol) were dissolved in 30 mL of 1% acetic acid (HAc) solution. Then, the MS sponge was immersed and repeatedly squeezed into the CS-zinc ion solution completely. A glutaraldehyde (GLA) aqueous solution (60 μL, 25%) was added and stirred to further assist in cross-linking. Then, the sponge was retained in the solution for 4 h at 60 °C to ensure sufficient cross-linking. The zinc ion-crosslinked chitosan sponge was cooled naturally, followed by washing with deionized water to remove the excess chitosan and zinc ions on the sponge. Afterwards, the sponge was further freeze-dried for 48 h. The CS–zinc ions composite sponge is labelled as CMZn. Subsequently, the CMZn sponge was added into 40 mL of 2-methylimidazole (HMeIM, 1.642 g) methanol solution and stirred for 4 h. The sponge was washed and further freeze-dried for 48 h. The as-obtained sample was labelled as the CMZ8 sponge. Moreover, we carried out the control samples (CMZ8-2 and CMZ8-6) with 2 and 6 mmol of Zn(NO3)2·6H2O to investigate the influence of zinc ion adjuvants. The experimental material information is provided in section ESI.1.† In addition, ZIF-8 (Z8) nanoparticles as the control sample were synthesized as described in section ESI.2.†
Property experiments
The characterization instrumentation, anti-biofouling assay tests, batch adsorption experiments to explore the effect of pH, contact time and kinetics study, different initial concentrations of U(VI) and adsorption isotherm studies on the adsorption amounts, adsorption cycle experiments, related low concentration and coexisting ion adsorption experiments in natural seawater are shown in ESI S3–S9.†
Results and discussion
Characterization of MS, CMZn and CMZ8 sponges
The preparation schematic diagram of the CMZ8 sponge is shown in Fig. 1. The porous MS substrate was filled with chitosan–zinc ion HAc solution to fast form the “egg-box” sponges using zinc ions as assisted cross-linking agents. Subsequently, zinc ions as nucleation anchor points combined with the active sites of the ligand to overcome the chemical inertness of the MS sponge and promote the formation of ZIF-8 on the sponge. The surface morphology and structure of MS, CMZn and CMZ8 sponges are shown in Fig. 2. The macropores (80–120 μm) and the smooth skeleton surface can be observed on the pure MS sponge (Fig. 2a and d). In contrast, the pore diameters of the CMZn and CMZ8 sponge retain macropores (50–100 μm) due to the ZIF-8/chitosan coating. This result confirms that the 3D macropore structure of pristine MS is inherited by the CMZ8 sponge, along with the slight decrease in porosity (Fig. 2c). In the magnified images (Fig. 2e and f), the skeleton surface of the CMZ8 sponge is rougher than MS and CMZn; obviously, many nanoparticles were grown on the surface of the CMZ8 sponge. Moreover, we investigated the influence of zinc ions on the morphology and structure of the sponge. As shown in Fig. S1a and b† and Fig. 2c, the cross-linking of chitosan increases when the content of zinc ions increases, making the excessive polymer flakes gradually occupy the interspaces of MS sponge and destroy the three-dimensional substrate structure. In contrast, the increase of the zinc ion content is beneficial for the growth of ZIF-8 nanoparticles (Fig. S1c and d† and Fig. 2f). The result confirms that zinc ions as adjuvants can simultaneously control the three-dimensional porous structure and the growth of ZIF-8. Therefore, we selected CMZ8 sponge with 4 mmol of zinc nitrate hexahydrate for further testing.
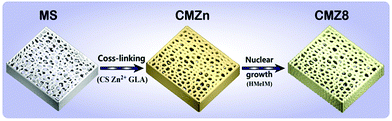 |
| Fig. 1 Synthesis diagram of CMZ8 sponge. | |
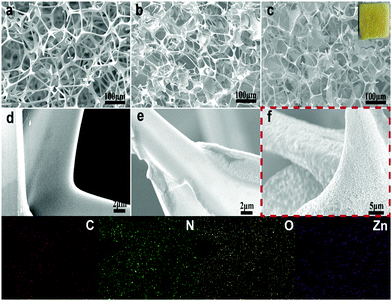 |
| Fig. 2 SEM images of MS (a and d), CMZn (b and e), and CMZ8 sponge (c and f) and EDS-mapping images of CMZ8 sponge (inset image is a digital photo of the CMZ8 sponge). | |
The related EDS mappings exhibit well distributed C, N, O, and Zn elements on the entire surface of the CMZ8 sponge, further affirming the formation of the ZIF-8 crystal layer. Moreover, the roughness surface of the CMZ8 sponge enhances the solid/liquid interface area to promote the efficient diffusion of uranium. Subsequently, Fig. S2† shows the TEM images of pure Z8 nanoparticles and the CMZ8 sponge. In contrast, the morphology of nanoparticles grown on CMZ8 is similar to that of pure Z8 with a particle size of about 80 nm, which confirms that ZIF-8 nanoparticles are successfully grown on melamine sponges.
In addition, the crystal and chemical structures of Z8, MS, CMZn and CMZ8 were investigated by XRD and FTIR tests. The representative crystalline diffraction peaks of ZIF-8 of the CMZ8 sponge are shown in Fig. 3a.31 The FTIR spectra of Z8, MS, CMZn and CMZ8 sponges are displayed in Fig. 3b. Compared to pristine MS, the spectra of CMZn and CMZ8 retain the triazine ring bending of MS at 811 cm−1.32 For the CMZn sponge, an obvious absorption peak can be observed at 3344 cm−1 due to the stretching vibration of N–H and O–H; the absorption peaks of 1626 cm−1 and 1560 cm−1 are attributed to amide and imine groups of chitosan.33 After the growth of ZIF-8, the CMZ8 sponge exhibits the typical absorption peak of N–H and O–H groups at around 3400 cm−1. In comparison, the absorption peak of amide groups changes to 1629 cm−1, and the peak of imine and C
N (with imidazole) overlaps at 1585 cm−1; moreover, some new peaks of the CMZ8 sponge at 1500–500 cm−1 are consistent with the typical absorption band of ZIF-8.34,35 These results further imply the successful growth of Z8 nanoparticles on the MS sponge. Moreover, chitosan does not change the morphological and crystal structures of ZIF-8 and endows the ZIF-8 composite sponge with abundant organic functional groups.
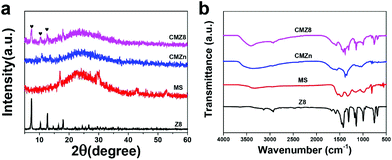 |
| Fig. 3 The XRD (a) and FTIR (b) images of Z8, MS, CMZn and CMZ8 sponges. | |
Mechanical properties
As the mechanical properties of adsorbents are a key factor in practical application, we carried out compressive tests. A series of compressive stress–strain curves of pristine MS, CMZn and CMZ8 sponges are shown in Fig. 4. The CMZn sponge presents similar stress–strain behaviour to pure MS sponge. It also exhibits a higher mechanical performance with 0.321 MPa of the compressive strength at 95% of strain than MS (0.114 MPa) due to the cross-linking of chitosan–zinc ions–GLA. In contrast, the compressive strength of CMZ8 (0.565 MPa) increases about 395.61% for pure MS sponge, owing to the strong and robust structure by integrating inorganic ZIF-8 nanoparticles and the chitosan matrix. Thus, the CMZ8 sponge displays superior mechanical properties.
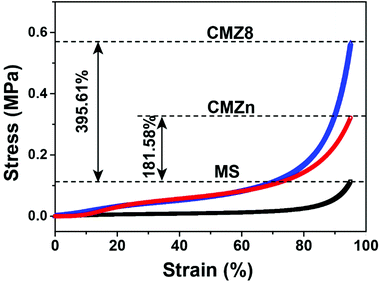 |
| Fig. 4 Compressive stress–strain curves of MS, CMZn and CMZ8 sponges. | |
Anti-biofouling properties
The anti-biofouling abilities of samples were further studied via Nitzschia as a model alga in Fig. 5 and 6. The algae concentration of pure MS is close to that of blank algae during 7 days to prove its non-cytotoxic effect. The algae survival amount of Z8 also retains a relatively high level (more than 90%) which exhibits a slight inhibition activity. Notably, both CMZn and CMZ8 sponges show similar inhibition for the algae. The algae death rate of CMZn is nearly 37%, which affirms that the antimicrobial property of chitosan is preserved in the sponge. Importantly, the algae cell death rate of the CMZ8 sponge is still nearly 35%, suggesting that chitosan is the main active ingredient for the inhibitory effect on Nitzschia.
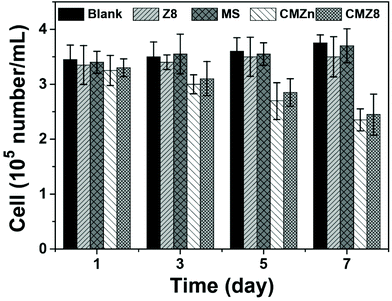 |
| Fig. 5 Algae density of blank, Z8, MS, CMZn and CMZ8 sponges in solutions on different days. | |
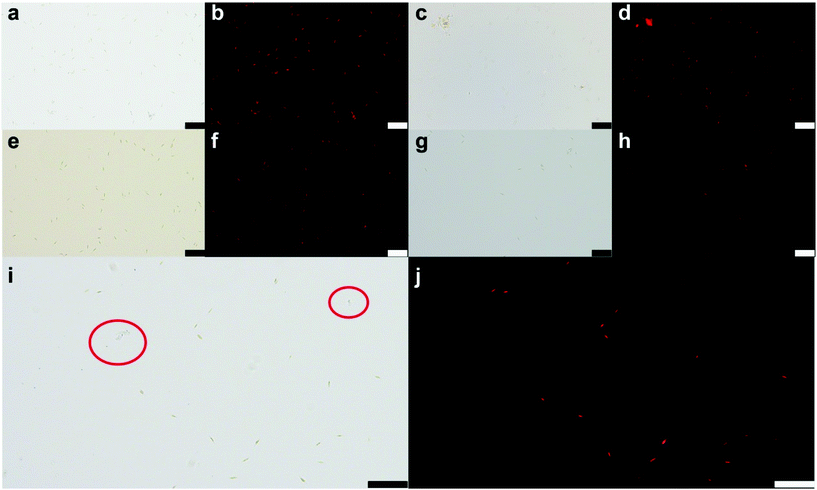 |
| Fig. 6 The natural light and fluorescence images of blank (a and b), Z8 (c and d), MS (e and f), CMZn (g and h) and CMZ8 (i and j) sponge cultivated in the algae solution after 7 days (all scale bars are 50 μm). | |
As shown in Fig. 6, massive active algae cells (red) can be observed in the blank algae solution and MS sponge. Of note, the concentration of active cells (red) in the solution of CMZn and CMZ8 sponge is significantly reduced. Moreover, the partial algae cell structure is severely damaged after 7 days of incubation with CMZ8 sponge (Fig. 6j). It is speculated that chitosan in the CMZ8 sponge changes the permeability of the microbial cell membrane and causes the collapse of the algae cells.36,37 Moreover, we also investigated the adhesion behaviour of algae on the surface of the CMZ8 sponge (Fig. S3†). There are only a few active algae cells that adhere to the CMZ8 sponge. This indicates that the sponge might exert an inhibitory effect on the growth and adhesion of algae cells by destroying the cell structure. These results demonstrate the practical perspective for applications of the CMZ8 sponge.
Effect of pH and ionic strength on uranium adsorption
In view of the effect of solution pH on adsorbent surface properties and the form of U(VI), the adsorption amounts of the as-synthesized adsorbents were probed into different pH values. In Fig. 7, pure MS shows weak U(VI) uptake at pH 2.0–9.0, but Z8 and CMZn exhibit different pH values for uranium uptake. Z8 achieves a high uptake capacity at pH 3.0.38 However, its adsorption capacity decreases obviously in the range of high pH and reaches 91.45 mg g−1 at pH 8.0 with nearly seawater environment, because the content of the negative charged uranium species complex form increases at pH 7.0–9.0.39,40 In practical seawater environment, the powder form and optimum adsorption pH of Z8 limit its potentiality. In contrast, the U(VI)-uptake capacity of CMZn is 103.85 mg g−1 and higher than that of pure Z8 at pH 8.0, confirming that the addition of chitosan is more beneficial for the adsorption of the complex uranium species. For the CMZ8 sponge, its optimal pH is still 3.0 and identical to that of Z8 nanoparticles, but the U(VI)-uptake amount of CMZ8 increases significantly in the range of pH 7.0–9.0. Of note, the adsorption capacity of the CMZ8 sponge further enhanced and reached 129.9 mg g−1 at pH 8.0. This result is mainly beneficial for chitosan which increases the active functional groups to promote the negatively charged U(VI) form adsorption of the CMZ8 sponge.
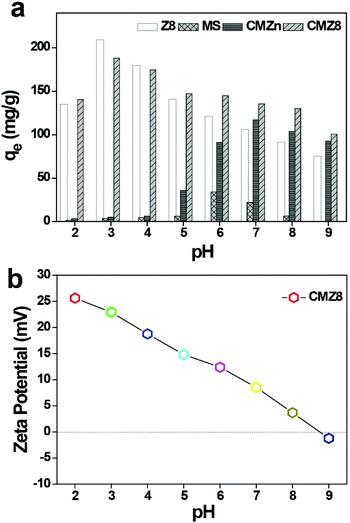 |
| Fig. 7 The adsorption amounts of Z8, MS, CMZn and CMZ8 sponges at different pH values (T = 298 K, m = 0.01 g, V = 50 mL, t = 12 h, CU-initial = 50 mg L−1) (a); the zeta potential of CMZ8 sponge with different pH values (b). | |
Subsequently, a zeta potential test was performed to determine the surface properties of the CMZ8 sponge. As shown in Fig. 7b, CMZ8 exhibits positive potential characteristics in the pH range of 2.0–9.0 and reaches 3.67 mV of the surface potential at pH 8.0. The type of U(VI)41 is mainly distributed with UO2(CO3)22− and UO2(CO3)34− at pH 8.0, implying that there is an electrostatic interaction between U(VI) and the surface of CMZ8. These results indicate that the chitosan and Zn ions promote the formation of the ZIF-8 sponge and improve the adsorption performance of conventional ZIF-8 materials. Therefore, the CMZ8 sponge is more beneficial for the practical seawater environment.
We further investigated the effect of NaCl and NaNO3 with different concentrations on the U(VI) adsorption of the CMZ8 sponge. As shown in Fig. S4,† the adsorption capacity of the CMZ8 sponge is reduced to about 120 mg g−1 after adding 0.001 mol L−1 NaCl or NaNO3. We suggest that the decrease in the adsorption capacity is mainly ascribed to the hydrated layer formed by sodium hydrated ions in the solution to block the contact between U(VI) and the material.42 Moreover, as the content of sodium ions increases, the hydrated layer on the surface of the material will be compressed.43 However, as the concentration of NaCl and NaNO3 increases to 0.1 mol L−1, the adsorption capacity of CMZ8 remains about 120 mg g−1 without significant changes. Therefore, these results confirm that the U(VI)-uptake of CMZ8 does not obviously change with ionic strength, and U(VI) overcomes the hydration layer effect to interact with the functional groups of the CMZ8 sponge during the adsorption process.
Kinetics and isotherms of the U(VI) adsorption
The effect of contact time on the U(VI)-uptake amount of CMZ8 was investigated using Z8 as a comparison. As shown in Fig. 8, CMZ8 exhibits a longer adsorption time (almost 120 min) than Z8 (nearly 60 min). However, both Z8 and CMZ8 exhibit a rapid adsorption behaviour at the initial 30 min. Notably, a higher adsorption capacity of CMZ8 can be observed to affirm its tremendous potentiality. The experimental data were fitted by pseudo-first-order, pseudo-second-order and Morris–Weber models with eqn (S4)–(S6).† The linear fitting curves and parameters of kinetic models are shown in Fig. 8 and Table S1.† The pseudo-second-order kinetic models of Z8 and CMZ8 display higher correlation coefficients (R2 = 0.999) than the pseudo-first-order kinetic equation (R2Z8 = 0.809 and R2CMZ8 = 0.921). Furthermore, the calculated adsorption amounts (qe cal) of CMZ8 and Z8 are nearly the value of experimental adsorption capacity (qe exp). The results suggest that the adsorption process of CMZ8 and Z8 is more inclined to follow pseudo-second-order so that the adsorption is mainly controlled by the chemisorption mechanism. Subsequently, the Morris–Weber model linear fitting curves of the Z8 and CMZ8 sponge (Fig. 8d) exhibit the typical multi-stage adsorption process. It is different from Z8 in that the fitting curve of the CMZ8 sponge has tri-linearity stages due to its 3D composite porous structure. The initial U(VI) uptake is mainly through external diffusion and is adsorbed to the surface by coordination with CMZ8.44 As the adsorption time increases, U(VI) further diffuses into the hole and is adsorbed on the inner surface-active site of the hole. Then, the N2 adsorption–desorption isotherms and pore size distribution curves of CMZ8 were further obtained and are shown in Fig. S5.† CMZ8 shows a high specific surface area (141.45 m2 g−1) and a hierarchically porous structure with a large number of micropores and macropores, which corresponds to the typical pore structure of ZIF-8 nanoparticles and the MS sponge matrix. Furthermore, the previous SEM images also determined that the CMZ8 sponge possessed a three-dimensional porous composite structure which was conducive to exposing active sites to facilitate the internal diffusion of U(VI) until the final adsorption equilibrium.45 Therefore, these results suggest that the U(VI) adsorption of the CMZ8 sponge is mainly controlled by the coordination action, accompanied by the partially intra-particle diffusion of U(VI).
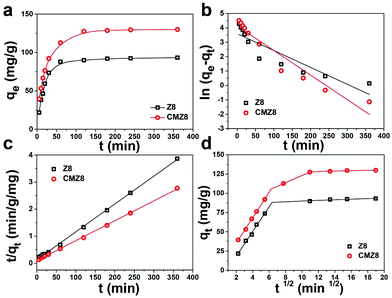 |
| Fig. 8 U(VI) uptake capacity of Z8 and CMZ8 sponge at different times (a), pseudo-first-order (b), pseudo-second-order (c) and Weber–Morris (d) models linearly fitted curves (T = 298 K, m = 0.01 g, V = 50 mL, CU-initial = 50 mg L−1, pH = 8.0). | |
Different initial U(VI) concentrations were applied for evaluating the adsorption behaviour of the CMZ8 sponge. As shown in Fig. 9, the U(VI)-uptake capacity of CMZ8 is significantly higher than that of Z8, which proves that chitosan could increase the active sites of the sponge to improve its adsorption capacity. The experimental data were fitted by Langmuir, Freundlich and Dubinin–Radushkevich models (eqn (S7)–(S10)†). The linear fitting curves and the calculated relevant parameters are shown in Fig. 9b–d and Table S2.† According to the correlation coefficient, both Z8 and the CMZ8 sponge display better fitting results (R2 > 0.97) of the Langmuir and Freundlich models than the Dubinin–Radushkevich model (R2Z8 = 0.634 and R2CMZ8 = 0.579). As calculated from the Langmuir model, the saturated U(VI) uptake capacity of CMZ8 is 299.4 mg g−1,higher than that of Z8 (180.51 mg g−1) at pH 8.0. However, Z8 conforms to the Langmuir model (R2 = 0.997), but the CMZ8 sponge tends to the Freundlich model (R2 = 0.991). The result implies that heterogeneous U(VI) adsorption occurs on the CMZ8 sponge, due to the uneven distribution of adsorption sites caused by its three-dimensional porous structure.46
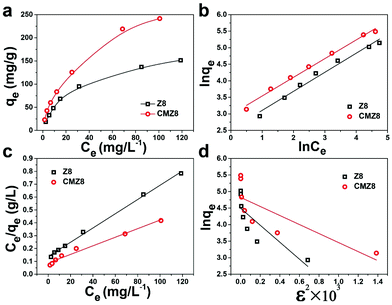 |
| Fig. 9 Adsorption isotherms of Z8 and CMZ8 sponge (a), Freundlich (b), Langmuir (c), and D–R (d) models linearly fitted curves (T = 298 K, m = 0.01 g, V = 50 mL, t = 3 h, pH = 8.0). | |
The comparison results on the U(VI)-uptake adsorption capacity of CMZ8 with other adsorbents are shown in Table S3,† including the chitosan composites, carbon materials, conventional MOF materials, ZIF-8 composites and antifouling materials. The saturated U(VI)-uptake capacity of the CMZ8 sponge is higher than most chitosan composites, carbon materials, conventional MOFs and other antifouling materials. Significantly, the CMZ8 sponge is more suitable for applications than other ZIF composite adsorbents, in the seawater environment (pH ≈ 8.3). Moreover, the pre-crosslinked chitosan–zinc ions and the sponge matrix provide abundant active sites and structure to support the macroscopic shape of the ZIF-8 composite adsorbent and facilitate adsorption, separation and recovery in seawater.
Potential adsorption mechanism of CMZ8 sponge
The U(VI) uptake mechanism of CMZ8 sponge was further investigated by exploring the chemical compositional change between CMZ8 and CMZ8-U. Compared with CMZ8 (Fig. 10a), a new peak appears in CMZ8-U at 910 cm−1 and belongs to the O
U
O characteristic absorption peak,47 and the overlapping peak of –OH and N–H at 3400 cm−1 is shifted to 3416 cm−1. The C
N peak at 1585 cm−1 is moved to 1597 cm−1, indicating that U(VI) is successfully adsorbed on CMZ8 and there is a coordination effect of U(VI) with the surface nitrogen and oxygen functional groups. Subsequently, the XPS spectrogram comparison of CMZ8 and CMZ8-U (Fig. 10b) shows that the new peak of CMZ8-U corresponds to the U 4f.48 In addition, for CMZ8-U, the binding energies of N 1s and O 1s change, indicating that the nitrogen and oxygen functional groups of CMZ8 sponge participated in the adsorption process of U(VI). Then, the N 1s and O 1s spectrograms were further analysed and are shown in Fig. 10c and d. The N 1s spectra of the original CMZ8 sponge are located at the 399.12 eV and 399.7 eV of binding energy peaks, corresponding to imidazole nitrogen and imide structures;49 and they shift the lower binding energy after U(VI) adsorption with 0.58 eV and 0.2 eV, respectively. According to the O 1s spectra of CMZ8 and CMZ8-U (Fig. 10d), the hydroxyl binding energy at 532.3 eV moved to 531.8 eV along with adsorbed water (529.9 eV), possibly due to the differences in the synthesis and adsorption processes or continuous exposure to air. Therefore, combining the previous zeta, kinetics, FTIR and XPS data, we speculated three interactions in the CMZ8 sponge during the adsorption of U(VI) (Fig. 11). These adsorption interactions include the electrostatic effects, intraparticle diffusion and coordination of functional groups (imidazole nitrogen, imide, amino and hydroxyl) of the CMZ8 sponge with U(VI).
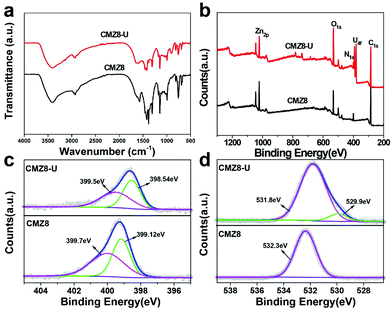 |
| Fig. 10 FTIR (a), XPS survey spectra (b), N 1s (c) and O 1s (d) of XPS spectroscopy of CMZ8 and CMZ8-U sponges. | |
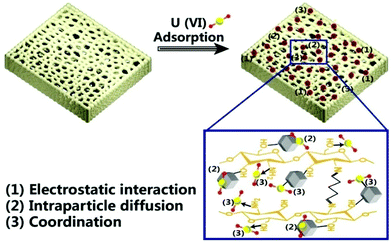 |
| Fig. 11 Schematic diagram of the adsorption mechanism of U(VI) on CMZ8 sponge. | |
Recyclability study of the CMZ8 sponge
Generally, the adsorbent cyclic adsorption performance is considered as a necessary condition to ensure economic feasibility and long-term cost-effectiveness. We investigated the effect of different desorbents on the eluent efficiency of the CMZ8 sponge. As shown in Fig. S6,† the eluent efficiency of EDTA is 85.12% and is significantly higher than those of other desorbents. Therefore, the EDTA solution was selected as the eluent for the next test. Subsequently, the effects of different EDTA concentrations and the number of adsorption–desorption cycles on the performance of the CMZ8 sponge were determined. As shown in Fig. 12a, the eluent efficiency of the CMZ8 sponge increases with the EDTA concentration. When the concentration of EDTA is 0.5 mol L−1, the eluent efficiency increases to 92.47%. This may be attributed to the increased concentration of EDTA, which promotes the complex ability and releases enough H+ to compete with U(VI). However, when the concentration of EDTA was increased to 1 mol L−1, the eluent efficiency retains 92.68%, indicating that there are some non-renewable active sites on the CMZ8 sponge to cause the incomplete desorption. Therefore, 0.5 mol L−1 of EDTA was selected as the desorbent.
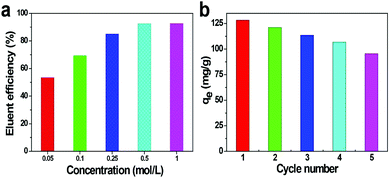 |
| Fig. 12 The elution efficiency of CMZ8 sponge with different concentrations of EDTA solution (a) and cyclic adsorption amount of CMZ8 sponge (b). | |
As shown in Fig. 12b, the adsorption capacity of the CMZ8 sponge reduced after continuous adsorption–desorption cycles. This phenomenon is caused by the degradation of the composite layer on the CMZ8 sponge during the adsorption and desorption process, which leads to a decrease in the adsorption active sites, as shown in the SEM image of CMZ8-D5 (Fig. S7a†). On the other hand, some active sites are still occupied by U(VI) due to the incomplete desorption during the desorption process. Fortunately, the CMZ8-D5 sponge still retains the characteristic diffraction peaks of ZIF-8 to confirm the relative stability of the CMZ8 adsorbent (Fig. S7b†). Moreover, the adsorption capacity of CMZ8 can reach 95.47 mg g−1 at the fifth cycle, which is higher than the initial adsorption capacity of the ZIF-8 adsorbent (91.45 mg g−1). The results show that CMZ8 sponge is better than the original ZIF-8 and more suitable for U(VI) extraction from seawater.
Uranium capture from seawater studies
In order to study the practical application effect of CMZ8, we evaluated the low-concentration U(VI) adsorption performance of CMZ8 under seawater conditions. As shown in Fig. 13, the U(VI)-uptake amounts of the CMZ8 sponge reached 11.39 μg g−1 and the U(VI) removal rate (65.45%) is significantly higher than those of other coexisting ions. The selective uranium adsorption is ascribed to the special affinity between U(VI) and a large number of nitrogen-containing functional groups on the surface of the CMZ8 sponge is relatively strong.50 In addition, we further explored the adsorption performance of the CMZ8 sponge in simulated polluted seawater (Fig. 13b). The removal rate of CMZ8 is nearly 70% at different initial U(VI) concentrations. These results show that CMZ8 sponge has excellent application potential for extracting uranium from seawater with a low concentration of uranium.
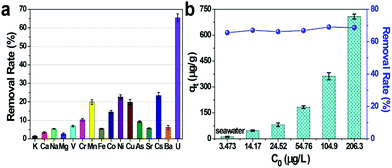 |
| Fig. 13 U(VI) selectivity removal rate (a) and adsorption capacity and removal rate (b) of CMZ8 sponge in seawater. | |
Conclusions
An antifouling ZIF-8/chitosan/melamine sponge uranium adsorbent (CMZ8) was synthesized by combining ion-assisted cross-linking with in situ growth, which overcomes the challenges in MS sponge matrix chemical inertness and ZIF traditional powder for actual applications. The adsorbent exhibited an obvious 3D porous structure and ZIF-8 nanoparticles were evenly distributed on the surface of the sponge. The CMZ8 sponge showed excellent mechanical properties with a compressive stress of up to 0.565 MPa. Moreover, the algae cell death rate of the CMZ8 sponge was 35% and was significantly higher than those of MS (∼0%) and Z8 (<10%), indicating that chitosan was the main anti-biofouling ingredient. At pH 8.0, the adsorption amount of CMZ8 sponge (129.9 mg g−1) is 142% of ZIF-8 powder (91.45 mg g−1). Importantly, in the fifth adsorption–desorption cycle, the CMZ8 sponge adsorbent can reach a uranium adsorption capacity of 95.47 mg g−1 which is higher than the initial adsorption capacity of pure ZIF-8 powders. The low-concentration uranium in the seawater adsorption experiment displayed that the uranium removal rate of the sponge was close to 70%. These results confirmed that zinc ions and chitosan as adjuvants overcome the chemical stability of the sponge matrix to provide sufficient active sites for the growth of ZIF-8 and also can effectively improve the antibiofouling and adsorption properties of ZIF-8 to make the CMZ8 sponge more suitable for the seawater environment.
Author contributions
Xuejie Guo: Writing – original draft and investigation. Haocheng Yang: Software, validation and writing – review & editing. Jun Wang: Conceptualization, supervision and writing – review & editing.
Conflicts of interest
There are no conflicts to declare.
Acknowledgements
This work was supported by the Funding for school-level research projects of Yancheng Institute of Technology (xjr2021034) and the National Natural Science Foundation of China (NSFC 21905066 and NSFC 51872057).
References
- S. Chu and A. J. n. Majumdar, Opportunities and challenges for a sustainable energy future, Nature, 2012, 488, 294–303 CrossRef CAS.
- H. Lindner and E. Schneider, Review of cost estimates for uranium recovery from seawater, Energy Econ., 2015, 49, 9–22 CrossRef.
- J. Kim, C. Tsouris, R. T. Mayes, Y. Oyola, T. Saito, C. J. Janke, S. Dai, E. Schneider and D. Sachde, Recovery of uranium from seawater: a review of current status and future research needs, Sep. Sci. Technol., 2013, 48, 367–387 CrossRef CAS.
- C. W. Abney, R. T. Mayes, T. Saito and S. Dai, Materials for the recovery of uranium from seawater, Chem. Rev., 2017, 117, 13935–14013 CrossRef CAS PubMed.
- Z. Wang, Q. Meng, R. Ma, Z. Wang, Y. Yang, H. Sha, X. Ma, X. Ruan, X. Zou and Y. J. C. Yuan, Constructing an ion pathway for uranium extraction from seawater, Chem, 2020, 6, 1683–1691 CAS.
- P. Zhang, L. Wang, K. Du, S. Wang, Z. Huang, L. Yuan, Z. Li, H. Wang, L. Zheng, Z. Chai and W. Shi, Effective removal of U(VI) and Eu(III) by carboxyl functionalized MXene nanosheets, J. Hazard. Mater., 2020, 396, 122731 CrossRef CAS PubMed.
- S. Shi, Y. Qian, P. Mei, Y. Yuan, N. Jia, M. Dong, J. Fan, Z. Guo and N. Wang, Robust flexible poly(amidoxime) porous network membranes for highly efficient uranium extraction from seawater, Nano Energy, 2020, 71, 104629 CrossRef CAS.
- F. Endrizzi, C. J. Leggett and L. Rao, Scientific basis for efficient extraction of uranium from seawater I: understanding the chemical speciation of uranium under seawater conditions, Ind. Eng. Chem. Res., 2016, 55, 4249–4256 CrossRef CAS.
- M. Ahmad, J. Chen, K. Yang, T. Shah, M. u. d. Naik, Q. Zhang and B. Zhang, Preparation of amidoxime modified porous organic polymer flowers for selective uranium recovery from seawater, Chem. Eng. J., 2021, 418, 129370 CrossRef CAS.
- Y. Yuan, S. Feng, L. Feng, Q. Yu, T. Liu and N. Wang, Spatial structure bio-inspired nano-pocket for targeting uranyl capture, Angew. Chem., Int. Ed., 2020, 59, 4262–4268 CrossRef CAS.
- T. Zhang, J. Chen, H. Xiong, Z. Yuan, Y. Zhu and B. Hu, Constructing new Fe3O4@MnOx with 3D hollow structure for efficient recovery of uranium from simulated seawater, Chemosphere, 2021, 283, 131241 CrossRef CAS PubMed.
- X. H. Xiong, Z. W. Yu, L. L. Gong, Y. Tao, Z. Gao, L. Wang, W. H. Yin, L. X. Yang and F. Luo, Ammoniating covalent organic framework (COF) for high - performance and selective extraction of toxic and radioactive uranium ions, Adv. Sci., 2019, 6, 1900547 CrossRef.
- Q. Yu, Y. Yuan, L. Feng, T. Feng, W. Sun and N. Wang, Spidroin-inspired, high-strength, loofah-shaped protein fiber for capturing uranium from seawater, Angew. Chem., Int. Ed., 2020, 59, 15997–16001 CrossRef CAS.
- W.-R. Cui, C.-R. Zhang, W. Jiang, F. F. Li, R. P. Liang, J. Liu and J. D. Qiu, Regenerable and stable sp(2) carbon-conjugated covalent organic frameworks for selective detection and extraction of uranium, Nat. Commun., 2020, 11, 1–10 Search PubMed.
- W. Yang, Q. Pan, S. Song and H. Zhang, Metal-organic framework-based materials for the recovery of uranium from aqueous solutions, Inorg. Chem. Front., 2019, 6, 1924–1937 RSC.
- Y. Liu, Y. Huo, X. Wang, S. Yu, Y. Ai, Z. Chen, P. Zhang, L. Chen, G. Song and N. S. Alharbi, Impact of metal ions and organic ligands on uranium removal properties by zeolitic imidazolate framework materials, J. Clean. Prod., 2021, 278, 123216 CrossRef CAS.
- Y. Liu, H. Pang, X. Wang, S. Yu, Z. Chen, P. Zhang, L. Chen, G. Song, N. S. Alharbi, S. O. Rabah and X. Wang, Zeolitic imidazolate framework-based nanomaterials for the capture of heavy metal ions and radionuclides: A review, Chem. Eng. J., 2021, 406, 127139 CrossRef CAS.
- H. N. Abdelhamid and A. P. Mathew, Biointerface between ZIF-8 and biomolecules and their applications, Biointerface Res. Appl. Chem., 2021, 11, 8283–8297 CAS.
- H. N. Abdelhamid, Zeolitic Imidazolate Frameworks (ZIF-8) for Biomedical Applications: A Review, Curr. Med. Chem., 2021, 28, 7023–7075 CrossRef CAS.
- H. y. Wu, S. t. Li, Y. w. Shao, X. z. Jin, X. d. Qi, J. h. Yang, Z. w. Zhou and Y. Wang, Melamine foam/reduced graphene oxide supported form-stable phase change materials with simultaneous shape memory property and light-to-thermal energy storage capability, Chem. Eng. J., 2020, 379, 122373 CrossRef CAS.
- Y. Zhan, S. He, J. Hu, S. Zhao, G. Zeng, M. Zhou, G. Zhang and A. Sengupta, Robust super-hydrophobic/super-oleophilic sandwich-like UIO-66-F-4@rGO composites for efficient and multitasking oil/water separation applications, J. Hazard. Mater., 2020, 388, 121752 CrossRef CAS PubMed.
- J. Ma, H. Fan, W. Zhang, J. Sui, C. Wang, M. Zhang, N. Zhao, A. K. Yadav, W. Wang, W. Dong and S. Wang, High sensitivity and ultra-low detection limit of chlorine gas sensor based on In2O3 nanosheets by a simple template method, Sens. Actuators, B, 2020, 305, 127456 CrossRef CAS.
- D. Wang, J. Song, S. Lin, J. Wen, C. Ma, Y. Yuan, M. Lei, X. Wang, N. Wang and H. Wu, A marine-inspired hybrid sponge for highly efficient uranium extraction from seawater, Adv. Funct. Mater., 2019, 29, 1901009 CrossRef.
- H. N. Abdelhamid and A. P. Mathew, Cellulose–metal organic frameworks (CelloMOFs) hybrid materials and their multifaceted Applications: A review, Coord. Chem. Rev., 2022, 451, 214263 CrossRef CAS.
- J. Park, G. A. Gill, J. E. Strivens, L. J. Kuo, R. T. Jeters, A. Avila, J. R. Wood, N. J. Schlafer, C. J. Janke, E. A. Miller, M. Thomas, R. S. Addleman and G. T. Bonheyo, Effect of biofouling on the performance of amidoxime-based polymeric uranium adsorbents, Ind. Eng. Chem. Res., 2016, 55, 4328–4338 CrossRef CAS.
- Q. Yu, Y. Yuan, J. Wen, X. Zhao, S. Zhao, D. Wang, C. Li, X. Wang and N. Wang, A universally applicable strategy for construction of anti-biofouling adsorbents for enhanced uranium recovery from seawater, Adv. Sci., 2019, 6, 1900002 CrossRef.
- D. Kim, D. W. Kim, O. Buyukcakir, M. K. Kim, K. Polychronopoulou and A. Coskun, Highly hydrophobic ZIF-8/Carbon Nitride Foam with hierarchical porosity for oil capture and chemical fixation of CO2, Adv. Funct. Mater., 2017, 27, 1700706 CrossRef.
- Z. Lei, Y. Deng and C. Wang, Multiphase surface growth of hydrophobic ZIF-8 on melamine sponge for excellent oil/water separation and effective catalysis in a Knoevenagel reaction, J. Mater. Chem. A, 2018, 6, 3258–3263 RSC.
- Z. Ma, A. Garrido-Maestu and K. C. Jeong, Application, mode of action, and in vivo activity of chitosan
and its micro- and nanoparticles as antimicrobial agents: A review, Carbohydr. Polym., 2017, 176, 257–265 CrossRef CAS.
- G. Michailidou, I. Koumentakou, E. V. Liakos, M. Lazaridou, D. A. Lambropoulou, D. N. Bikiaris and G. Z. Kyzas, Adsorption of Uranium, Mercury, and Rare Earth Elements from Aqueous Solutions onto Magnetic Chitosan Adsorbents: A Review, Polymers, 2021, 13, 3137 CrossRef CAS.
- M. Zhu, S. R. Venna, J. B. Jasinski and M. A. Carreon, Room-temperature synthesis of zif-8: the coexistence of zno nanoneedles, Chem. Mater., 2011, 23, 3590–3592 CrossRef CAS.
- V. H. Pham and J. H. Dickerson, Superhydrophobic silanized melamine sponges as high efficiency oil absorbent materials, ACS Appl. Mater. Interfaces, 2014, 6, 14181–14188 CrossRef CAS.
- X. Zhong, Y. Sun, Z. Zhang, Y. Dai, Y. Wang, Y. Liu, R. Hua, X. Cao and Y. Liu, A new hydrothermal cross-linking ion-imprinted chitosan for high-efficiency uranium removal, J. Radioanal. Nucl. Chem., 2019, 322, 901–911 CrossRef CAS.
- M. J. C. Ordoñez, K. J. Balkus, J. P. Ferraris and I. H. Musselman, Molecular sieving realized with ZIF-8/Matrimid® mixed-matrix membranes, J. Membr. Sci., 2010, 361, 28–37 CrossRef.
- Y. Feng, Y. Y. Wang, Y. Q. Wang, X. F. Zhang and J. F. Yao,
In situ gelation of sodium alginate supported on melamine sponge for efficient removal of copper ions, J. Colloid Interface Sci., 2018, 512, 7–13 CrossRef CAS PubMed.
- T. Kean and M. Thanou, Biodegradation, biodistribution and toxicity of chitosan, Adv. Drug Delivery Rev., 2010, 62, 3–11 CrossRef CAS PubMed.
- X. Guo, H. Yang, Q. Liu, J. Liu, R. Chen, H. Zhang, J. Yu, M. Zhang, R. Li and J. Wang, A chitosan-graphene oxide/ZIF foam with anti-biofouling ability for uranium recovery from seawater, Chem. Eng. J., 2020, 382, 122850 CrossRef CAS.
- C. Wang, T. Zheng, R. Luo, C. Liu, M. Zhang, J. Li, X. Sun, J. Shen, W. Han and L. Wang, In situ growth of ZIF-8 on PAN fibrous filters for highly efficient U(VI) removal, ACS Appl. Mater. Interfaces, 2018, 10, 24164–24171 CrossRef CAS.
- Y. Sun, Z. Y. Wu, X. Wang, C. Ding, W. Cheng, S. H. Yu and X. Wang, Macroscopic and microscopic investigation of U(VI) and Eu(III) adsorption on carbonaceous nanofibers, Environ. Sci. Technol., 2016, 50, 4459–4467 CrossRef CAS.
- D. Panias and A. Krestou, Uranium(VI) speciation diagrams in the UO22+/CO32−/H2O system at 25 °C, Eur. J. Miner. Process. Environ. Prot., 2004, 4, 113–129 Search PubMed.
- H. J. Schenk, L. Astheimer, E. G. Witte and K. Schwochau, Development of sorbers for the recovery of uranium from seawater 1. assessment of key parameters and screening studies of sorber materials, Sep. Sci. Technol., 1982, 17, 1293–1308 CrossRef CAS.
- F. Rao, F. J. Ramirez-Acosta, R. J. Sanchez-Leija, S. Song and A. Lopez-Valdivieso, Stability of kaolinite dispersions in the presence of sodium and aluminum ions, Appl. Clay Sci., 2011, 51, 38–42 CrossRef CAS.
- M. Manciu and E. Ruckenstein, The polarization model for hydration/double layer interactions: the role of the electrolyte ions, Adv. Colloid Interface, 2004, 112, 109–128 CrossRef CAS PubMed.
- X. Yi, J. He, Y. Guo, Z. Han, M. Yang, J. Jin, J. Gu, M. Ou and X. Xu, Encapsulating Fe3O4 into calcium alginate coated chitosan hydrochloride hydrogel beads for removal of Cu(II) and U(VI) from aqueous solutions, Ecotoxicol. Environ. Saf., 2018, 147, 699–707 CrossRef CAS PubMed.
- Q. Sun, B. Aguila, J. Perman, A. S. Ivanov, V. S. Bryantsev, L. D. Earl, C. W. Abney, L. Wojtas and S. Ma, Bio-inspired nano-traps for uranium extraction from seawater and recovery from nuclear waste, Nat. Commun., 2018, 9, 1–9 CrossRef.
- Y. R. He, S. C. Li, X. L. Li, Y. Yang, A. M. Tang, L. Du, Z. Y. Tan, D. Zhang and H. B. Chen, Graphene (rGO) hydrogel: A promising material for facile removal of uranium from aqueous solution, Chem. Eng. J., 2018, 338, 333–340 CrossRef CAS.
- P. Yang, Q. Liu, J. Liu, H. Zhang, Z. Li, R. Li, L. Liu and J. Wang, Interfacial growth of a metal-organic framework (UiO-66) on functionalized graphene oxide (GO) as a suitable seawater adsorbent for extraction of uranium(VI), J. Mater. Chem. A, 2017, 5, 17933–17942 RSC.
- X. y. Zheng, X. y. Wang, Y. h. Shen, X. Lu and T. s. Wang, Biosorption and biomineralization of uranium(VI) by saccharomyces cerevisiae-crystal formation of chernikovite, Chemosphere, 2017, 175, 161–169 CrossRef CAS.
- H. Zeng, L. Wang, D. Zhang, P. Yan, J. Nie, V. K. Sharma and C. Wang, Highly efficient and selective removal of mercury ions using hyperbranched polyethylenimine functionalized carboxymethyl chitosan composite adsorbent, Chem. Eng. J., 2019, 358, 253–263 CrossRef CAS.
- D. M. King, F. Tuna, E. J. McInnes, J. McMaster, W. Lewis, A. J. Blake and S. T. Liddle, Synthesis and structure of a terminal uranium nitride complex, Science, 2012, 337, 717–720 CrossRef CAS PubMed.
Footnote |
† Electronic supplementary information (ESI) available. See DOI: 10.1039/d1qi01203g |
|
This journal is © the Partner Organisations 2022 |
Click here to see how this site uses Cookies. View our privacy policy here.