DOI:
10.1039/D1QI01185E
(Research Article)
Inorg. Chem. Front., 2022,
9, 146-154
In situ construction of FeNi2Se4-FeNi LDH heterointerfaces with electron redistribution for enhanced overall water splitting†
Received
18th September 2021
, Accepted 3rd November 2021
First published on 9th November 2021
Abstract
Developing hierarchical heterostructures as bifunctional electrocatalysts which promote renewable hydrogen production is a desirable but challenging technology in electrochemical water splitting. Herein, the interface engineering of the FeNi2Se4-FeNi LDH composite is achieved by the in situ growth of FeNi LDH nanosheets and the subsequent partial selenization treatment. Enhanced mass transfer and accelerated gas release are achieved in such a unique self-supported hierarchical heterostructure during overall water splitting. Moreover, the strong electronic interaction between FeNi2Se4 and FeNi LDH optimizes the electron redistribution of Fe and Ni sites, which significantly improves the interfacial reactivity of reactants and/or intermediates with the hybrid catalyst. Benefiting from increased active sites and enhanced intrinsic activity, the hybrid electrocatalyst requires only a potential of 205 mV for the oxygen evolution reaction (OER) and a potential of 106 mV for the hydrogen evolution reaction (HER) to realize 10 mA cm−2. Specifically, the optimized FeNi2Se4-FeNi LDH as a bifunctional electrode in overall water splitting delivers 10 mA cm−2 at a low cell voltage of 1.56 V driven by a solar cell. This strategy by rational design of heterostructures thus paves an efficient pathway to fabricate active and non-precious bifunctional electrocatalysts for overall water splitting.
Introduction
Water splitting is one of the most attractive approaches for producing hydrogen fuel in a clean and sustainable way when coupled with other renewable energy conversion technologies.1–6 Nevertheless, such an approach has not been widely deployed in industrial applications. The overall water splitting process includes two half reactions, the anodic oxygen evolution reaction (OER) and the cathodic hydrogen evolution reaction (HER).7,8 Both processes are severely restrained by the high energy barriers and sluggish reaction kinetics,9–11 which result in unfavorable overpotential and unsatisfactory energy conversion efficiency.12–15 Hence, electrocatalysts with adequate OER and HER activities are indispensable to realize efficient overall water splitting. Presently, noble metal-based electrocatalysts, such as RuO2 and Pt/C, are regarded as top-of-the-line electrocatalysts for the OER and HER, respectively. But the large-scale application of noble metal-based electrocatalysts is severely hindered due to their scarcity and high costs.16,17 On the other side, those noble metal-based catalysts are not able to catalyze both the HER and OER simultaneously. Using different catalysts for anodic and cathodic reactions will increase the cost and design complexity of the electrolyzer.18–20 Thus, it is of crucial importance to develop superior performance bifunctional electrocatalysts for overall water splitting based on abundant and non-precious elements.21,22
Layered double hydroxides (LDHs) have attracted increasing interest as OER electrocatalysts due to their unique layered structures, which are advantageous for the mass transfer process and gas release.23,24 Moreover, the tunable chemical composition and unique edge-share octahedral MO6 layer-stacking crystal structure of LDHs provide versatile pathways to regulate their activities.25,26 Such features make LDHs promising precursors to fabricate highly active catalysts. The HER process is another essential reaction in the overall water splitting process. However, it is commonly known that LDHs are inactive toward the HER in base medium, which hinders their practical application. Taking advantage of the heterogeneous interface engineering by hybridizing LDHs with other active materials in order to tune the activity has been generally explored and made significant progress.27 Many electrocatalysts with abundant heterogeneous interfaces have been well developed at present.28,29 Specifically, constructing heterogeneous interfaces effectively adjusts the electron distribution with abundant active sites, which can optimize the adsorption/desorption of hydrogen and facilitate the water splitting process.30–32 The strong coupling effects between different components result in superior overall water splitting activity compared to the pristine counterparts.33,34 Selenides are one of the most attractive HER catalysts due to their low binding energy with hydrogen atoms compared with sulfides and phosphides.33,35 The weak Se–H strength can facilitate hydrogen desorption from catalytic sites and promote the subsequent reaction.36–38 Hence, interface engineering of LDH and selenide heterostructures is a rational strategy for fabricating bifunctional catalysts in water splitting.
With due consideration of the design principles above, we prepared an excellent electrocatalyst with a hierarchical heterostructure assembled by FeNi2Se4-FeNi LDH for overall water splitting. The catalyst is endowed with enhanced electron transfer, increased active sites and promotion of intermediate adsorption, which is attributed to the strong electronic coupling induced by interfaces between FeNi LDH and FeNi2Se4. The FeNi2Se4-FeNi LDH exhibits small overpotentials of 205 mV for the OER and 106 mV for the HER at 10 mA cm−2. Specifically, a cell voltage of only 1.56 V is needed to drive the overall water splitting with FeNi2Se4-FeNi LDH as the bifunctional electrocatalyst. This work not only provides an efficient water splitting catalyst but also inspires further development of bifunctional electrocatalysts by constructing interface engineered heterostructures.
Experimental section
Chemicals and materials
All the chemicals were directly used as received without further purification. Nickel nitrate hexahydrate (Ni(NO3)2·6H2O), ammonium fluoride (NH4F), potassium hydroxide (KOH), urea (CON2H4), sodium borohydride (NaBH4), selenium (Se) powder and iron nitrate nonahydrate (Fe(NO3)3·9H2O) were purchased from Macklin. While polyvinylidene fluoride (PVDF, 99%), Nafion (5 wt%), ruthenium oxide (RuO2) and platinum on graphitized carbon (Pt/C, 20 wt%) were obtained from Aladdin (Shanghai, China). The deionized (DI) water produced by a Milli-Q water purification system was used in all experiments.
Synthesis of FeNi LDH/NF
Commercial nickel foams (NFs) were cleaned thoroughly with 2.0 M hydrochloric acid and then washed with DI water and anhydrous ethanol for 30 min. In detail, 0.5 mmol of Ni(NO3)2·6H2O, 0.5 mmol of Fe(NO3)3·9H2O, 7.5 mmol of NH4F and 3 mmol of urea were ultrasonically dispersed in 30 mL of DI water to form a homogeneous solution. After that, the solution was transferred into a 50 mL Teflon-lined stainless steel autoclave. Subsequently, a piece of a pretreated substrate (2 × 3 cm2) was leaned against the autoclave. The autoclave was sealed and reacted at 120 °C for 6 h. The sample was taken out and washed with ethanol. After drying, FeNi LDH/NF was finally obtained.
Synthesis of hierarchical FeNi2Se4-FeNi LDH/NF
In a typical synthesis, 65 mg of NaBH4 was dissolved in 35 mL of DI water, followed by the addition of 59 mg of Se powder. After stirring for 30 min, the transparent solution was transferred into a 50 mL Teflon-lined stainless autoclave. A piece of FeNi LDH/NF (2 × 3 cm2) was immersed in an autoclave and reacted at 120 °C for 2 h for partial selenization. After cooling naturally to room temperature, the black sample was purified with DI water and ethanol several times, and dried in a vacuum oven at 60 °C for 12 h. With regard to FeNi2Se4/NF, the reaction temperature was increased to 160 °C without other conditions.
Structure and surface characterization
The morphologies and structures of the samples were characterized using a Regulus 8100 scanning electron microscope and FEI Tecnai G2 F20 microscope at an accelerating voltage of 200 kV with an energy-dispersive X-ray spectrometer (EDX), transmission electron microscope (TEM) and high-resolution transmission electron microscope (HRTEM). The chemical composition and crystallinity were analyzed using a Bruker D8 Advance X-ray diffraction (XRD) system with Cu-Kα radiation. The powder samples were peeled off from the surface of NF. The chemical states of the elements on the surface were evaluated by Thermo X-ray photoelectron spectroscopy (XPS) (ESCALAB 250Xi). The surface area analysis was carried out using the Brunauer–Emmett–Teller (BET) method from N2 adsorption–desorption isotherms (V-Sorb 2800P).
Electrochemical measurements
The electrocatalytic measurements of the catalysts were carried out on the CS electrochemical workstation in an ambient environment. The prepared FeNi LDH/NF, FeNi2Se4/NF and FeNi2Se4-FeNi LDH/NF were directly used as the working electrode (WE), respectively. The Ag/AgCl electrode saturated with KCl solution was utilized as the reference electrode (RE). A carbon rod was employed as the counter electrode (CE) for HER measurement while the platinum wire was chosen for the OER. All measured potentials were calibrated to potential versus reversible hydrogen electrode (RHE) according to the Nernst equation, E (V vs. RHE) = E (V vs. Ag/AgCl) + 0.1989 + 0.059 pH − IR. The working electrode was electrochemically activated at a scan rate of 100 mV s−1 by the cyclic voltammetry (CV) test prior to the linear sweep voltammetry (LSV) test. Then the LSV measurements were performed in 1.0 M KOH (pH = 13.6) at a scan rate of 1 mV s−1. The Tafel slope was obtained by fitting the linear part of the Tafel plots according to the equation (η = a + b
log(j)) to evaluate the kinetic performance of as-prepared catalysts, where η, a, b and j represent the overpotential, the potential at 1 mA cm−2, the Tafel slope and the current density. The electrochemical impedance spectroscopy (EIS) measurements were recorded in the frequency range from 0.1 to 105 Hz and a 5 mV amplitude, at 0.5 V versus Ag/AgCl for the OER and −1.129 V versus Ag/AgCl for the HER. The electrochemically active surface areas (ECSAs) evaluated at a potential range where the non-faradaic process occurred were obtained by CV tested with different scan rates from 40 to 120 mV s−1. The double layered capacitance (Cdl) can be calculated by the half slope of the linearly fitted curve of the capacitive current (Δj = janodic − jcathodic) plotted against the scan rate.39 In order to evaluate the long-term durability of electrocatalysts, a constant voltage was applied using chronoamperometry. All LSV curves were corrected with 80% iR-compensation.
Results and discussion
The synthetic process of the FeNi2Se4-FeNi LDH heterostructure via a two-step route is illustrated in Scheme 1, which involves the growth of the 2D FeNi LDH nanosheets and the subsequent in situ growth of FeNi2Se4 by partial selenization. The digital image (Fig. S1†) shows that the color of the sample transformed from earthy yellow to black after the selenization treatment, indicating the formation of the selenides. The scanning electron microscopy (SEM) images reveal that the FeNi LDH nanosheets with a relatively smooth surface were evenly and vertically grown on the NF (Fig. 1a and d) after the hydrothermal reaction. With the subsequent selenization treatment at dedicated controlled hydrothermal temperature, the obtained FeNi2Se4-FeNi LDH (Fig. 1b and e) exhibits a slightly rougher surface than the FeNi LDH without selenization. However, the vertically grown and spatially interconnected network is well retained, which is beneficial for exposing active sites, providing an open channel for the accessibility of electrolytes, and facilitating the rapid release of gas bubbles. Selenization at different temperatures was also carried out. FeNi2Se4-FeNi LDH-90 (Fig. S2†) shows an edge covered by FeNi2Se4 while most of the FeNi LDH maintains a smooth surface, indicating that selenization at such temperatures cannot convert the FeNi LDH to FeNi2Se4 sufficiently. With an increase in the temperature of the selenization reaction, the surface of the nanosheets became rough gradually (Fig. 1c and f). It is noteworthy that even at a high selenization temperature, the morphology of FeNi LDH is well maintained, suggesting the successfully preserved LDH layered structure under the selected selenization conditions. In addition, the low magnitude SEM images in the inset of Fig. 1a–c reveal that the NF substrate is fully covered by nanosheets. Moreover, Fig. 1g shows a typical TEM image of the as-synthesized FeNi2Se4-FeNi LDH. The area of light contrast represents the FeNi LDH, while the dark contrast area highlighted by yellow circles is attributed to FeNi2Se4. In the HRTEM (Fig. 1h), the lattice fringes with interplanar spacings of 0.223 and 0.261 nm are indexed to the (015) and (101) planes of FeNi LDH, while the lattice distances of 0.269 and 0.240 nm correspond to the (020) and (202) planes of FeNi2Se4. The selected area electron diffraction (SAED) pattern confirms the co-existence of FeNi LDH and FeNi2Se4 in the heterostructure (Fig. 1i). Specifically, the heterogeneous interface has more defects and disorder which leads to more active sites.40,41 Furthermore, the mapping images (Fig. 1j) and the EDX spectrum (Fig. S3†) of FeNi2Se4-FeNi LDH/NF manifest the uniform distribution of Fe, Ni, Se and O elements throughout the whole composite. These results confirm the successful fabrication of the FeNi2Se4-FeNi LDH heterostructure with distinct interfaces between FeNi LDH and FeNi2Se4.
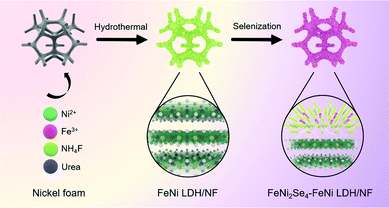 |
| Scheme 1 Schematic illustration for the preparation of FeNi2Se4-FeNi LDH on NF by in situ growth of FeNi LDH and the subsequent partial selenization. | |
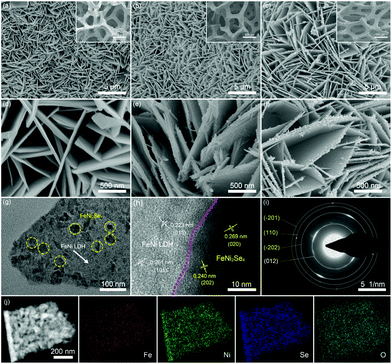 |
| Fig. 1 (a–c) Low magnitude SEM images and (d–f) high magnitude SEM images of FeNi LDH, FeNi2Se4-FeNi LDH and FeNi2Se4, respectively. (g) TEM, (h) HRTEM, (i) SAED pattern and (j) the corresponding elemental mapping images of FeNi2Se4-FeNi LDH. | |
For the purpose of investigating the chemical phases and crystal structures of the as-synthesized electrocatalysts, the XRD measurements were carried out (Fig. 2a). The appearance of peaks at 11.6°, 23.4° and 34.5° is assigned to the (003), (006) and (012) lattice planes of FeNi LDH, respectively. After the selenization process, the FeNi2Se4-FeNi LDH shows newly emerged characteristic diffraction peaks at 33.4°, 39.1°, 45.1° and 51.0° which are consistent with the (111), (−312), (−511) and (−313) lattice planes of FeNi2Se4, indicating the successful formation of selenides. It is worth noting that the increase of the selenization temperature to 160 °C can ultimately convert the FeNi LDH into FeNi2Se4 (FeNi2Se4-160) while a low selenization temperature of 90 °C cannot convert the FeNi LDH sufficiently (FeNi2Se4-FeNi LDH-90), as evidenced by the peaks that are only attributed to FeNi2Se4 in FeNi2Se4-160 and the apparent peaks of FeNi LDH in FeNi2Se4-FeNi LDH-90 (Fig. S4†). Besides, the Raman spectra in Fig. S5† show that both FeNi LDH and FeNi2Se4-FeNi LDH possess two peaks at 467.39 and 553.12cm−1, manifesting the characteristic peaks of NiFe(OH)x.42 It reflects that FeNi2Se4-FeNi LDH retains the characteristic peaks of FeNi LDH.
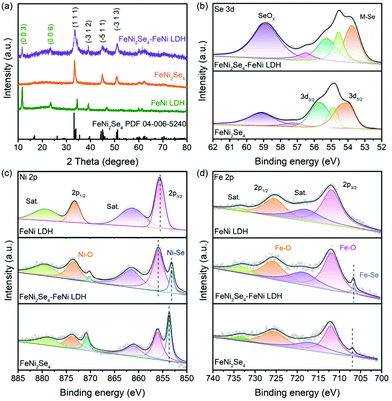 |
| Fig. 2 (a) XRD patterns of the electrocatalysts. (b) Se 3d spectra, (c) Ni 2p spectra and (d) Fe 2p spectra of the electrocatalysts FeNi LDH, FeNi2Se4 and FeNi2Se4-FeNi LDH, respectively. | |
Further insight into the variation of surface chemical states and electronic interaction through partial selenization is provideed by XPS spectra (Fig. S6†). In the Se 3d region (Fig. 2b), the XPS spectrum confirmed the formation of a metal selenide bond (M–Se) with a peak at 54.51 eV along with the oxidized Se species at 58.89 eV, commonly seen when exposed to the air.43–45 The O 1s spectrum of the heterostructure in Fig. S7† can be deconvoluted into three signals, which corresponds to metal–oxygen (M–O) bonds, chemically adsorbed O–H species and physically adsorbed H2O molecules, respectively.46 The peak-fitting analysis of Ni 2p spectra is shown in Fig. 2c; the peaks at 873.56 and 855.94 eV along with satellite peaks are attributed to Ni 2p1/2 and Ni 2p3/2 which belonged to the Ni–O bond, while the other two peaks at 853.21 and 870.19 eV belonged to the Ni–Se bond.47–50 Specifically, the Ni–Se bond shifts slightly to lower binding energies compared to FeNi2Se4, while the Ni–O bond shifts slightly to higher binding energies compared to FeNi LDH, indicating that the electron transfer occurred on the interface between LDH and FeNi2Se4. For the Fe 2p spectrum of FeNi2Se4-FeNi LDH (Fig. 2d), the high-resolution peaks at 725.90 and 712.00 eV are attributed to Fe 2p1/2 and Fe 2p3/2 corresponding to the Fe–O bond, respectively. The other two peaks at 733.26 and 719.05 eV correspond to the shakeup satellite peaks. The peak at 706.72 eV is attributed to the Fe–Se bond.51,52 As shown in Fig. S8, S9 and S10,† the SBET value of FeNi2Se4-FeNi LDH is 22.19 m2 g−1, which is much higher than FeNi LDH (18.07 m2 g−1) and FeNi2Se4 (18.06 m2 g−1). This result proves that the FeNi2Se4-FeNi LDH has a higher surface area, offering more catalytic active sites for the HER/OER. The above results indicate the electron transfer between the FeNi2Se4-FeNi LDH heterostructure interfaces, simultaneously manifesting that a strong electronic coupling has been formed at the interfaces, leading to the electron redistribution.53
The electrocatalytic OER performance of the as-synthesized electrocatalysts was examined in base medium. The LSV measurement of different selenization temperatures (Fig. S11†) reveals that FeNi2Se4-FeNi LDH/NF-120 possesses a lower overpotential than FeNi2Se4-FeNi LDH/NF-90 and FeNi2Se4-160. Hence it is chosen for further studies. As shown in Fig. 3a, the optimal FeNi2Se4-FeNi LDH on NF possesses an η10 of 205 mV (acquired from the chronoamperometry test), which is lower than that of FeNi LDH/NF (240 mV), FeNi2Se4/NF (226.9 mV) RuO2/NF (257.6 mV) and bare nickel foam (360 mV). The mass activity (Fig. S12†) also confirms the superior intrinsic OER activity of FeNi2Se4-FeNi LDH. Moreover, FeNi2Se4-FeNi LDH requires a lower overpotential of 266 mV to afford a higher current density of 100 mA cm−2 (Fig. 3b). Besides, the smallest Tafel slope (Fig. 3c) of FeNi2Se4-FeNi LDH/NF (30.14 mV dec−1) amongst FeNi LDH/NF (46.05 mV dec−1), FeNi2Se4/NF (56.91 mV dec−1) and RuO2/NF (100.16 mV dec−1), implying its strong OER kinetics owing to the fast electron transfer. The EIS can also be used to understand the catalytic kinetics. The diameter of the semicircle of FeNi2Se4-FeNi LDH in the high-frequency region is much smaller than those of its counterparts (Fig. 3d), indicating the higher conductivity and faster interfacial charge-transfer rate. The ECSA was calculated by the cyclic voltammetry (CV) strategy, which is identified to be positively correlated with the number of active sites (Fig. S13†). The FeNi2Se4-FeNi LDH/NF possesses a larger double-layer capacitance (Cdl) of 70.46 mF cm−2 than FeNi2Se4/NF (Fig. 3e). Stability is another important parameter to evaluate the performance of the catalyst. Fig. 3f shows the excellent durability of the FeNi2Se4-FeNi LDH/NF for 24 h. The inset shows the SEM image of the FeNi2Se4-FeNi LDH/NF after the chronoamperometry test for 10 h. It can be seen that the original morphology is maintained, indicating remarkable stability. The polarization curves of the FeNi2Se4-FeNi LDH/NF reveal little decay after 3000 CV cycles (Fig. S14†). Meanwhile, the XPS results after the OER stability test indicate that the binding energies of Fe 2p and Ni 2p shift slightly to higher binding energy regions due to the formation of metal oxide/hydroxide species during the OER process (Fig. S15†)38,54 The OER performance of the FeNi2Se4-FeNi LDH/NF is superior to most transition metal-based electrocatalysts reported recently (Fig. 3g and Table S1†), as the coupling interfaces in the composite can reduce the polarization by facilitating electron transfer. Moreover, the heterostructure could appreciably give rise to edge-enriched active sites, expand the active areas and then improve the OER activity.40,55
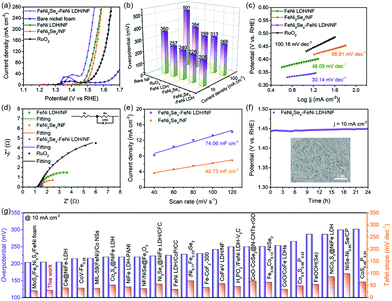 |
| Fig. 3 (a) LSV curves of bare NF, FeNi LDH/NF, FeNi2Se4-FeNi LDH/NF, FeNi2Se4/NF and RuO2/NF. (b) Overpotentials, (c) Tafel plots, (d) Nyquist plots, (e) Values of Cdl and (f) chronopotentiometry durability test with the inset SEM image of FeNi2Se4-FeNi LDH/NF after CV cycles. (g) η10 and Tafel slope comparison of the catalysts in this work with other reported high-performance OER electrocatalysts. | |
Besides the OER activity, the FeNi2Se4-FeNi LDH/NF also possesses outstanding HER performance in the same electrolyte. As shown in Fig. 4a, the FeNi2Se4-FeNi LDH/NF requires a low overpotential of 106 mV to deliver a current density of 10 mA cm−2, which is lower than the FeNi LDH/NF (227 mV), FeNi2Se4/NF (133 mV) and bare nickel foam (251 mV), respectively (Fig. 4b). It is worth noting that the FeNi2Se4-FeNi LDH still exhibits superior HER performance even after ruling out the influence of the catalyst loading (Fig. S16†). The Tafel slope values of the Pt/C, FeNi LDH/NF, FeNi2Se4-FeNi LDH/NF and FeNi2Se4/NF are 25.95, 138.58, 115.21 and 128.11 mV dec−1 (Fig. 4c), reflecting the stronger HER kinetics of the FeNi2Se4-FeNi LDH/NF. Fig. 4d shows the EIS measurement which aimed at studying the reaction kinetics of the electrocatalysts. All samples show only one semicircle in the Nyquist plot, indicating the electron transfer controlling feature of the process. Furthermore, a much smaller semicircle diameter of the Nyquist plot is observed in FeNi2Se4-FeNi LDH/NF, compared to the FeNi LDH/NF and FeNi2Se4/NF. Such a small diameter indicates the improved charge transfer resistance and electrode kinetics of the FeNi2Se4-FeNi LDH/NF. The stability test of the FeNi2Se4-FeNi LDH/NF was performed by chronoamperometry (Fig. 4e) and CVs after 3000 cycles (Fig. 4f). Little decay was observed, indicating high stability. The SEM images show that the nanosheet structure of FeNi2Se4-FeNi LDH remains unchanged after the HER measurement, indicating remarkable structural stability as well (Fig. S17†).
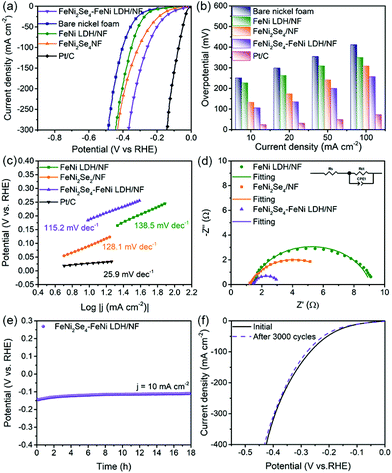 |
| Fig. 4 (a) HER LSV curves of bare NF, FeNi LDH/NF, FeNi2Se4-FeNi LDH/NF, FeNi2Se4/NF and Pt/C. (b) Overpotentials, (c) Tafel plots, (d) Nyquist plots and (e) chronopotentiometry durability test of the provided catalysts. (f) The LSV curves before and after CV cycles. | |
Inspired by the above results, the as-prepared FeNi2Se4-FeNi LDH/NF was directly employed as the self-standing anode and cathode simultaneously in an overall water splitting electrolyzer. As shown in Fig. 5a and Fig. S18,† the optimal selenization temperature is 120 °C, and the overpotential of FeNi2Se4-FeNi LDH/NF was substantially lower than those of its counterparts. Fig. 5b shows that the potentials to deliver the water splitting at 10 and 50 mA cm−2 are 1.56 V and 1.68 V for the FeNi2Se4-FeNi LDH/NF, which is among the best performances (Table S2†). The EIS measurement in Fig. 5c and inset demonstrates a clearly smaller semicircle of FeNi2Se4-FeNi LDH/NF than the FeNi LDH/NF and FeNi2Se4/NF. This result indicates that the heterostructure also exhibits an enhanced electron transfer process in overall water splitting. The electrolyzer still exhibits a high activity after 20 h of continuous water electrolysis, manifesting its high durability in the overall water splitting (Fig. 5d). The volumes of produced O2 and H2 during the process were recorded at regular intervals. It can be calculated to obtain the faradaic efficiency value of the FeNi2Se4-FeNi LDH/NF, which is nearly 90%. Besides, the ratio of generated O2 and H2 is close to 1
:
2 (Fig. 5e and Fig. S19†).56 In addition, we assembled a water splitting equipment system using commercial solar cells (Fig. 5f). This assembly can convert light energy into electric energy, which is environmentally friendly and provides hope for the applications of overall water splitting in the future. These results further reveal that the FeNi2Se4-FeNi LDH/NF acts as a bifunctional water splitting catalyst that exhibits highly active and stable electrocatalytic performance with great promise, which is superior to most other non-precious electrocatalysts reported previously (Fig. 5g).
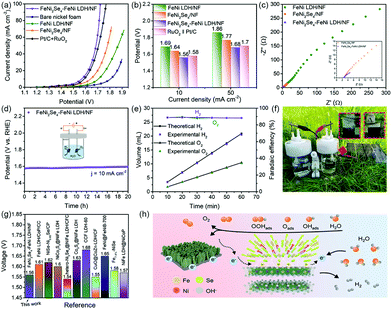 |
| Fig. 5 (a) Polarization. (b) Potentials at current densities of 10 mA−2 and 50 mA−2. (c) Nyquist plots. (d) Chronopotentiometry durability test. (e) The Faraday efficiency of water splitting. (f) Actual operation diagram of water splitting under the sun. (g) Comparison of overpotentials at 10 mA cm−2. (h) Diagrammatic sketch of the probable charge separation and transformation process of the alkaline water electrolysis on the interface of FeNi2Se4-FeNi LDH. | |
Based on the above discussion, we present a possible charge separation and mass transfer process of FeNi2Se4-FeNi LDH during the overall water splitting process (Fig. 5h). The merits of the impressive electrocatalytic activity of FeNi2Se4-FeNi LDH can be concluded as follows. On the one hand, the nanosheets can provide a more accessible electrochemically active surface area which is beneficial for mass transfer, exposing active sites and facilitating gas release. In addition, it has been demonstrated that a self-supported nanostructure is in favor of increasing the electrical conductivity.57,58 The electrolyte diffusion and gas release during the water splitting process can be enhanced due to the high porous flexibility and large specific surface area of NFs. Due to the high electrical conductivity, electrons can pass through the substrate quickly to dissociate H2O and OH− adsorbed on the nanosheets and simultaneously produce H2 and O2. On the other hand, the heterostructure builds a conductive meshwork which is beneficial for electron conversion in the whole structure. The abundant heterogeneous interfaces can effectively boost the electrocatalytic performance thanks to the strong interfacial coupling between FeNi LDH and FeNi2Se4, leading to electron redistribution and then changing the building energies of OER and HER reaction intermediates.59,60
Conclusion
In summary, we prepared a synergetic heterostructure consisting of FeNi LDH and FeNi2Se4 through a facile partial selenization strategy. The bifunctional FeNi2Se4-FeNi LDH/NF electrocatalyst exhibits superior activity for the OER and HER in 1.0 M KOH solution with overpotentials of only 205 and 106 mV to arrive at 10 mA cm−2. The two-electrode water splitting electrolyzer employing the FeNi2Se4-FeNi LDH/NF as a cathode and anode requires a cell voltage of 1.56 V to drive a current density of 10 mA cm−2. The partial selenization strategy in this work can strengthen the electronic interaction and speed up the charge transfer at the interfaces between FeNi2Se4 and FeNi LDH, which results in enhanced electroconductivity and increased active sites, accounting for superior electrocatalytic properties. It is anticipated that this work can offer novel design strategies that can inspire the exploration of high-efficient non-noble metal catalysts for electrochemical energy conversion applications.
Conflicts of interest
There are no conflicts to declare.
Acknowledgements
This work was supported by the National Natural Science Foundation of China (51871119 and 22075141), High-Level Entrepreneurial and Innovative Talents Program on Jiangsu Province, Jiangsu Provincial Founds for Natural Science Foundation (BK20180015), China Postdoctoral Science Foundation (2018M640481 and 2019T120426), and Jiangsu Postdoctoral Research Fund (2019K003).
Notes and references
- F. Podjaski, D. Weber, S. Zhang, L. Diehl, R. Eger, V. Duppel, E. Alarcón-Lladó, G. Richter, F. Haase, A. Fontcuberta i Morral, C. Scheu and B. V. Lotsch, Rational strain engineering in delafossite oxides for highly efficient hydrogen evolution catalysis in acidic media, Nat. Catal., 2020, 3, 55–63 CrossRef CAS.
- H. Huang, A. Cho, S. Kim, H. Jun, A. Lee, J. W. Han and J. Lee, Structural design of amorphous CoMoPx with abundant active sites and synergistic catalysis effect for effective water splitting, Adv. Funct. Mater., 2020, 30, 2003889 CrossRef CAS.
- H. Huang, S. Zhou, C. Yu, H. Huang, J. Zhao, L. Dai and J. Qiu, Rapid and energy-efficient microwave pyrolysis for high-yield production of highly-active bifunctional electrocatalysts for water splitting, Energy Environ. Sci., 2020, 13, 545–553 RSC.
- D. Yu, Y. Ma, F. Hu, C. C. Lin, L. Li, H. Y. Chen, X. Han and S. Peng, Dual-sites coordination engineering of single atom catalysts for elexible metal–air batteries, Adv. Energy Mater., 2021, 11, 2101242 CrossRef CAS.
- D. Ji, L. Fan, L. Li, S. Peng, D. Yu, J. Song, S. Ramakrishna and S. Guo, Atomically transition metals on self-supported porous carbon flake arrays as binder-free air cathode for wearable zinc−air batteries, Adv. Mater., 2019, 31, 1808267 CrossRef.
- X. Wu, L. Xia, Y. Wang, W. Lu, Q. Liu, X. Shi and X. Sun, Mn3O4 Nanocube: An efficient electrocatalyst toward artificial N2 fixation to NH3, Small, 2018, 14, 6–11 Search PubMed.
- J. Lee, A. Kumar, T. Yang, X. Liu, A. R. Jadhav, G. H. Park, Y. Hwang, J. Yu, C. T. K. Nguyen, Y. Liu, S. Ajmal, M. G. Kim and H. Lee, Stabilizing the OOH* intermediate: Via pre-adsorbed surface oxygen of a single Ru atom-bimetallic alloy for ultralow overpotential oxygen generation, Energy Environ. Sci., 2020, 13, 5152–5164 RSC.
- A. R. Jadhav, A. Kumar, J. Lee, T. Yang, S. Na, J. Lee, Y. Luo, X. Liu, Y. Hwang, Y. Liu and H. Lee, Stable complete seawater electrolysis by using interfacial chloride ion blocking layer on catalyst surface, J. Mater. Chem. A, 2020, 8, 24501–24514 RSC.
- X. Shi, A. Wu, H. Yan, L. Zhang, C. Tian, L. Wang and H. Fu, A ‘MOFs plus MOFs’ strategy toward Co-Mo2N tubes for efficient electrocatalytic overall water splitting, J. Mater. Chem. A, 2018, 6, 20100–20109 RSC.
- J. Liu, Z. Wang, K. Su, D. Xv, D. Zhao, J. Li, H. Tong, D. Qian, C. Yang and Z. Lu, Self-supported hierarchical IrO2@NiO nanoflake arrays as an efficient and durable catalyst for electrochemical oxygen evolution, ACS Appl. Mater. Interfaces, 2019, 11, 25854–25862 CrossRef CAS PubMed.
- L. Deng, F. Hu, M. Ma, S. Huang, Y. Xiong, H. Chen, L. Li and S. Peng, Electronic modulation caused by interfacial Ni–O–M (M=Ru, Ir, Pd) bonding for accelerating hydrogen evolution kinetics, Angew. Chem., Int. Ed., 2021, 60, 22276–22282 CrossRef CAS.
- H. Jin, S. Sultan, M. Ha, J. N. Tiwari, M. G. Kim and K. S. Kim, Simple and scalable mechanochemical synthesis of noble metal catalysts with single atoms toward highly efficient hydrogen evolution, Adv. Funct.
Mater., 2020, 30, 2000531 CrossRef CAS.
- Z. Kou, T. Wang, Q. Gu, M. Xiong, L. Zheng, X. Li, Z. Pan, H. Chen, F. Verpoort, A. K. Cheetham, S. Mu and J. Wang, Rational design of holey 2D nonlayered transition metal carbide/nitride heterostructure nanosheets for highly efficient water oxidation, Adv. Energy Mater., 2019, 9, 1803768 CrossRef.
- N. K. Dang, J. N. Tiwari, S. Sultan, A. Meena and K. S. Kim, Multi-site catalyst derived from Cr atoms-substituted CoFe nanoparticles for high-performance oxygen evolution activity, Chem. Eng. J., 2021, 404, 126513 CrossRef CAS.
- H. Yan, Y. Xie, A. Wu, Z. Cai, L. Wang, C. Tian, X. Zhang and H. Fu, Anion-modulated HER and OER activities of 3D Ni-V-based interstitial compound heterojunctions for high-efficiency and stable overall water splitting, Adv. Mater., 2019, 31, 1901174 CrossRef PubMed.
- S. Peng, F. Gong, L. Li, D. Yu, D. Ji, T. Zhang, Z. Hu, Z. Zhang, S. Chou, Y. Du and S. Ramakrishna, Necklace-like multishelled hollow spinel oxides with oxygen vacancies for efficient water electrolysis, J. Am. Chem. Soc., 2018, 140, 13644–13653 CrossRef CAS.
- U. Aftab, A. Tahira, R. Mazzaro, V. Morandi, M. I. Abro, M. M. Baloch, J. A. Syed, A. Nafady and Z. H. Ibupoto, Facile NiCo2S4/C nanocomposite: an efficient material for water oxidation, Tungsten, 2020, 2, 403–410 CrossRef.
- X. Cao, T. Wang and L. Jiao, Transition-metal (Fe, Co, and Ni)-based nanofiber electrocatalysts for water splitting, Adv. Fiber Mater., 2021, 3, 210–228 CrossRef.
- X. Ma, W. Chen, Q. Li, L. Xue and C. Peng, Nitrogen–doped hierarchical heterostructured aerophobic MoSx/Ni3S2 nanowires by one–pot synthesis: system engineering and synergistic effect in electrocatalysis of hydrogen evolution reaction, Energy Environ. Mater., 2021, 4, 658–663 CrossRef CAS.
- Z.-Y. Pan, Z. Tang, Y.-Z. Zhan and D. Sun, Three-dimensional porous CoNiO2@reduced graphene oxide nanosheet arrays/nickel foam as a highly efficient bifunctional electrocatalyst for overall water splitting, Tungsten, 2020, 2, 390–402 CrossRef.
- W. Zhou, D. D. Huang, Y. P. Wu, J. Zhao, T. Wu, J. Zhang, D. S. Li, C. Sun, P. Feng and X. Bu, Stable hierarchical bimetal–organic nanostructures as high performance electrocatalysts for the oxygen evolution reaction, Angew. Chem., Int. Ed., 2019, 58, 4227–4231 CrossRef CAS PubMed.
- T. Liu, P. Li, N. Yao, G. Cheng, S. Chen, W. Luo and Y. Yin, CoP-doped MOF-based electrocatalyst for pH-universal hydrogen evolution reaction, Angew. Chem., Int. Ed., 2019, 58, 4679–4684 CrossRef CAS PubMed.
- L. Yu, H. Zhou, J. Sun, F. Qin, F. Yu, J. Bao, Y. Yu, S. Chen and Z. Ren, Cu nanowires shelled with NiFe layered double hydroxide nanosheets as bifunctional electrocatalysts for overall water splitting, Energy Environ. Sci., 2017, 10, 1820–1827 RSC.
- T. Zhang, W. Zong, Y. Ouyang, Y. Wu, Y.-E. Miao and T. Liu, Carbon fiber supported binary metal sulfide catalysts with multi-dimensional structures for electrocatalytic nitrogen reduction reactions over a wide pH range, Adv. Fiber Mater., 2021, 3, 229–238 CrossRef.
- Y. Wang, Y. Zhang, Z. Liu, C. Xie, S. Feng, D. Liu, M. Shao and S. Wang, Layered double hydroxide nanosheets with multiple cacancies obtained by dry exfoliation as highly efficient oxygen evolution electrocatalysts, Angew. Chem., Int. Ed., 2017, 56, 5867–5871 CrossRef CAS.
- W. Xie, Y. Song, S. Li, M. Shao and M. Wei, Integrated nanostructural electrodes based on layered double hydroxides, Energy Environ. Mater., 2019, 2, 158–171 CrossRef CAS.
- Q. Liang, L. Zhong, C. Du, Y. Luo, J. Zhao, Y. Zheng, J. Xu, J. Ma, C. Liu, S. Li and Q. Yan, Interfacing epitaxial dinickel phosphide to 2D nickel thiophosphate nanosheets for boosting electrocatalytic water splitting, ACS Nano, 2019, 13, 7975–7984 CrossRef CAS.
- W. Lu, T. Liu, L. Xie, C. Tang, D. Liu, S. Hao, F. Qu, G. Du, Y. Ma, A. M. Asiri and X. Sun, In situ derived Co-B nanoarray: a high-efficiency and durable 3D bifunctional electrocatalyst for overall alkaline water splitting, Small, 2017, 13, 1700805 CrossRef.
- X. Xu, T. Wang, L. Dong, W. Lu and X. Miao, Energy-efficient hydrogen evolution reactions via hydrazine oxidation over facile synthesis of cobalt tetraoxide electrodes, ACS Sustainable Chem. Eng., 2020, 8, 7973–7980 CrossRef CAS.
- J. Diao, Y. Qiu, S. Liu, W. Wang, K. Chen, H. Li, W. Yuan, Y. Qu and X. Guo, Interfacial engineering of W2N/WC heterostructures derived from solid-state synthesis: A highly efficient trifunctional electrocatalyst for ORR, OER, and HER, Adv. Mater., 2020, 32, 1905679 CrossRef CAS.
- M. Kuang, J. Zhang, D. Liu, H. Tan, K. N. Dinh, L. Yang, H. Ren, W. Huang, W. Fang, J. Yao, X. Hao, J. Xu, C. Liu, L. Song, B. Liu and Q. Yan, Amorphous/crystalline heterostructured cobalt-vanadium-Iron (oxy)hydroxides for highly efficient oxygen evolution reaction, Adv. Energy Mater., 2020, 10, 2002215 CrossRef CAS.
- W. Huang, J. Zhang, D. Liu, W. Xu, Y. Wang, J. Yao, H. T. Tan, K. N. Dinh, C. Wu, M. Kuang, W. Fang, R. Dangol, L. Song, K. Zhou, C. Liu, J. W. Xu, B. Liu and Q. Yan, Tuning the electronic structures of multimetal oxide nanoplates to realize favorable adsorption energies of oxygenated intermediates, ACS Nano, 2020, 14, 17640–17651 CrossRef CAS.
- S. Anantharaj, S. R. Ede, K. Sakthikumar, K. Karthick, S. Mishra and S. Kundu, Recent trends and perspectives in electrochemical water splitting with an emphasis on sulfide, selenide, and phosphide catalysts of Fe, Co, and Ni: A review, ACS Catal., 2016, 6, 8069–8097 CrossRef CAS.
- C. F. Du, K. N. Dinh, Q. Liang, Y. Zheng, Y. Luo, J. Zhang and Q. Yan, Self-assemble and in situ formation of Ni1−xFexPS3 nanomosaic-decorated MXene hybrids for overall water splitting, Adv. Energy Mater., 2018, 8, 1801127 CrossRef.
- Z. Zhu, J. Hao, H. Zhu, S. Sun, F. Duan, S. Lu and M. Du, In situ fabrication of electrospun carbon nanofibers–binary metal sulfides as freestanding electrode for electrocatalytic water splitting, Adv. Fiber Mater., 2021, 3, 117–127 CrossRef.
- J. Yu, Y. Tian, F. Zhou, M. Zhang, R. Chen, Q. Liu, J. Liu, C. Y. Xu and J. Wang, Metallic and superhydrophilic nickel cobalt diselenide nanosheets electrodeposited on carbon cloth as a bifunctional electrocatalyst, J. Mater. Chem. A, 2018, 6, 17353–17360 RSC.
- X. Cao, E. Johnson and M. Nath, Expanding multinary selenide based high-efficiency oxygen evolution electrocatalysts through combinatorial electrodeposition: Case study with Fe-Cu-Co selenides, ACS Sustainable Chem. Eng., 2019, 7, 9588–9600 CrossRef CAS.
- Y. Dou, C. T. He, L. Zhang, H. Yin, M. Al-Mamun, J. Ma and H. Zhao, Approaching the activity limit of CoSe2 for oxygen evolution via Fe doping and Co vacancy, Nat. Commun., 2020, 11, 1664 CrossRef CAS.
- S. Sultan, J. N. Tiwari, J. H. Jang, A. M. Harzandi, F. Salehnia, S. J. Yoo and K. S. Kim, Highly efficient oxygen reduction reaction activity of graphitic tube encapsulating nitrided CoxFey Alloy, Adv. Energy Mater., 2018, 8, 1801002 CrossRef.
- M. Yuan, S. Dipazir, M. Wang, Y. Sun, D. Gao, Y. Bai, M. Zhang, P. Lu, H. He, X. Zhu, S. Li, Z. Liu, Z. Luo and G. Zhang, Polyoxometalate-assisted formation of CoSe/MoSe2 heterostructures with enhanced oxygen evolution activity, J. Mater. Chem. A, 2019, 7, 3317–3326 RSC.
- S. Hou, W. Li, S. Watzele, R. M. Kluge, S. Xue, S. Yin, X. Jiang, M. Döblinger, A. Welle, B. Garlyyev, M. Koch, P. Müller–Buschbaum, C. Wöll, A. S. Bandarenka and R. A. Fischer, Metamorphosis of heterostructured surface–mounted metal–organic frameworks yielding record oxygen evolution mass activities, Adv. Mater., 2021, 33, 2103218 CrossRef CAS.
- S. Lee, L. Bai and X. Hu, Deciphering iron-dependent activity in oxygen evolution catalyzed by nickel–iron layered double hydroxide, Angew. Chem., Int. Ed., 2020, 59, 8072–8077 CrossRef CAS PubMed.
- Y. Chen, Z. Ren, H. Fu, X. Zhang, G. Tian and H. Fu, NiSe-Ni0.85Se heterostructure nanoflake arrays on carbon paper as efficient electrocatalysts for overall water splitting, Small, 2018, 14, 1800763 CrossRef PubMed.
- C. Xia, Q. Jiang, C. Zhao, M. N. Hedhili and H. N. Alshareef, Selenide-based electrocatalysts and scaffolds for water oxidation applications, Adv. Mater., 2016, 28, 77–85 CrossRef CAS PubMed.
- K. Guo, Z. Zou, J. Du, Y. Zhao, B. Zhou and C. Xu, Coupling FeSe2 with CoSe: An effective strategy to create stable and efficient electrocatalysts for water oxidation, Chem. Commun., 2018, 54, 11140–11143 RSC.
- B. Zhao, J. Liu, C. Xu, R. Feng, P. Sui, L. Wang, J. Zhang, J. L. Luo and X. Z. Fu, Hollow NiSe nanocrystals heterogenized with carbon nanotubes for efficient electrocatalytic methanol upgrading to boost hydrogen Co-production, Adv. Funct. Mater., 2021, 31, 2008812 CrossRef CAS.
- J. Sun, X. Hu, Z. Huang, T. Huang, X. Wang, H. Guo, F. Dai and D. Sun, Atomically thin defect-rich Ni-Se-S hybrid nanosheets as hydrogen evolution reaction electrocatalysts, Nano Res., 2020, 13, 2056–2062 CrossRef CAS.
- T. Hao, Y. Liu, G. Liu, C. Peng, B. Chen, Y. Feng, J. Ru and J. Yang, Insight into faradaic mechanism of polyaniline@NiSe2 core-shell nanotubes in high-performance supercapacitors, Energy Storage Mater., 2019, 23, 225–232 CrossRef.
- Z. Feng, E. Wang, S. Huang and J. Liu, A bifunctional nanoporous Ni-Co-Se electrocatalyst with a superaerophobic surface for water and hydrazine oxidation, Nanoscale, 2020, 12, 4426–4434 RSC.
- J. Cao, K. Wang, J. Chen, C. Lei, B. Yang, Z. Li, L. Lei, Y. Hou and K. Ostrikov, Nitrogen-doped carbon-encased bimetallic selenide for high-performance water electrolysis, Nano-Micro Lett., 2019, 11, 67 CrossRef CAS PubMed.
- Y. Lan, J. Zhou, K. Xu, Y. Lu, K. Zhang, L. Zhu and Y. Qian, Synchronous synthesis of Kirkendall effect induced hollow FeSe2/C nanospheres as anodes for high performance sodium ion batteries, Chem. Commun., 2018, 54, 5704–5707 RSC.
- B. Xu, H. Yang, L. Yuan, Y. Sun, Z. Chen and C. Li, Direct selenylation of mixed Ni/Fe metal-organic frameworks to NiFe-Se/C nanorods for overall water splitting, J. Power Sources, 2017, 366, 193–199 CrossRef CAS.
- Y. Xu, X. Jiang, G. Shao, H. Xiang, S. Si, X. Li, T. S. Hu, G. Hong, S. Dong, H. Li, Y. Feng and S. Liu, Interface effect of Ru-MoS2 nanoflowers on lignin substrate for enhanced hydrogen evolution activity, Energy Environ. Mater., 2021, 4, 117–125 CrossRef CAS.
- X. Xu, F. Song and X. Hu, A nickel iron diselenide-derived efficient oxygen-evolution catalyst, Nat. Commun., 2016, 7, 12324 CrossRef CAS.
- S. P. Figerez, K. K. Tadi, K. R. Sahoo, R. Sharma, R. K. Biroju, A. Gigi, K. A. Anand, G. Kalita and T. N. Narayanan, Molybdenum disulfide–graphene van der Waals heterostructures as stable and sensitive electrochemical sensing platforms, Tungsten, 2020, 2, 411–422 CrossRef.
- J. Chen, F. Zheng, S. J. Zhang, A. Fisher, Y. Zhou, Z. Wang, Y. Li, B. B. Xu, J. T. Li and S. G. Sun, Interfacial interaction between FeOOH and Ni-Fe LDH to modulate the local electronic structure for enhanced OER electrocatalysis, ACS Catal., 2018, 8, 11342–11351 CrossRef CAS.
- S. Parvin, A. Kumar, A. Ghosh and S. Bhattacharyya, An earth-abundant bimetallic catalyst coated metallic nanowire grown electrode with platinum-like pH-universal hydrogen evolution activity at high current density, Chem. Sci., 2020, 11, 3893–3902 RSC.
- B. Liu, Y. F. Zhao, H. Q. Peng, Z. Y. Zhang, C. K. Sit, M. F. Yuen, T. R. Zhang, C. S. Lee and W. J. Zhang, Nickel–cobalt diselenide 3D mesoporous nanosheet networks supported on Ni foam: An all-pH highly efficient integrated electrocatalyst for hydrogen evolution, Adv. Mater., 2017, 29, 1606521 CrossRef.
- H. Lu, Y. Zhang, Y. Huang, C. Zhang and T. Liu, Reaction packaging CoSe2 nanoparticles in N-doped carbon polyhedra with bifunctionality for overall water splitting, ACS Appl. Mater. Interfaces, 2019, 11, 3372–3381 CrossRef CAS PubMed.
- K. Li, J. Zhang, R. Wu, Y. Yu and B. Zhang, Anchoring CoO domains on CoSe2 nanobelts as bifunctional electrocatalysts for overall water splitting in neutral media, Adv. Sci., 2015, 3, 1500426 CrossRef.
Footnote |
† Electronic supplementary information (ESI) available. See DOI: 10.1039/d1qi01185e |
|
This journal is © the Partner Organisations 2022 |
Click here to see how this site uses Cookies. View our privacy policy here.