DOI:
10.1039/D1RA07252H
(Paper)
RSC Adv., 2022,
12, 4931-4938
Cu(II)–vitamin C-complex catalyzed photo-induced homocoupling reaction of aryl boronic acid in base-free and visible light conditions†
Received
29th September 2021
, Accepted 2nd February 2022
First published on 8th February 2022
Abstract
In this work, the photocatalytic efficiency of Cu(II)–vitamin C complex immobilized on titanium dioxide nanoparticles was exploited in the photo-assisted homocoupling reaction of aryl boronic acids under heterogeneous conditions. The homocoupling reaction affords the corresponding symmetrical biaryls in 50–97% yields at ambient temperature in the air under visible light irradiation without any need for any additive such as base or oxidant. This method tolerates various substituents on the aryl boronic acids such as halogen, carbonyl, and a nitro group. The light-dependent photocatalytic performance of the title catalyst evaluated by action spectra revealed a maximum apparent quantum efficiency (AQYs) at 410 nm demonstrating the visible-light-driven photocatalytic reaction. The as-prepared nano biophotocatalyst proved to be reusable at least six times without losing its activity. Thus this work exhibits a favorable method from the environmental and economic point of view which enables the industrially important reactions such as coupling reactions, to be carried out efficiently under photocatalytic and practically attainable conditions.
1. Introduction
Nowadays the development of new catalytic methods for efficient and selective transformations should be conditioned to environmental and economical aspects. For this, the synthesis of robust, readily available, and active catalysts is necessary and it has become a very imperative topic for scientists working across all disciplines.1–3 Moreover, it is extremely favorable that the catalytic protocols involve suitable synthetic routes based on naturally sourced catalysts. Learning from nature, employing biomolecules is highly desirable in chemical transformations. In this context, several research groups have developed vitamin–metal complexes for various organic and bioorganic transformations.4–7 It is known that vitamins are easily available compounds showing many modes of metal coordination for biological processes. They have been used as bioligands for the construction of bioactive metal complexes exhibiting different architectures. Among the bio-relevant compounds, vitamin C-based metal complexes attracted the most attention.8–12 It is also essential for the biosynthesis of the pigment melanin and invaluable role in biological systems such as halting tumor growth in mice.8,13 Therefore, there is a strong incentive to develop organic transformation in this area.
Since this conception, the combination of photoredox catalysis with bio-based metal catalysis has proven itself to be a versatile way to achieve efficient organic transformation. The photocatalytic process has mainly dominated using capabilities such as efficiency, cost-effective and eco-friendly manner. Meanwhile, the use of titanium oxide in photocatalytic reactions is well known. However, recombination of electron/hole pairs causes to be its response to the narrow range of UV light. To solve this problem, various methods have been devised, one of which is surface modification. This method has become a valuable strategy using biocompatible compounds and is highly regarded.
Aryl carbon–carbon bond formation reactions are very demanding in organic synthesis reactions; particularly in the context of designing biologically active compounds and reactive organic functionalities having a broad spectrum of applications.14,15 Biaryls are important structural motifs that occur in many natural products and pharmaceutical compounds.16–18 Moreover, biaryls have found widespread applications in agrochemicals, dyes, semi-conductor, polymers, and optically active ligands.19–23 They are also found as components in new organic materials which make these compounds attractive man-made targets. Therefore, owing to the potent importance of biaryl moieties, the development of an efficient catalyst for the synthesis of biaryls would be highly desirable and continues to be the subject of intense investigations.
The construction of biaryl skeletons can be done using transition metal-mediated reactions including name reactions such as Suzuki, Stille, and Sonogashira cross-coupling reactions as well as their homocoupling alternatives.24–27
Homocoupling of organoboron compounds is a potentially valuable method for the synthesis of symmetrical biaryls because they are non-toxic, stable, and readily available from commercial sources.27–29 A commonly applied method for these reactions relies on palladium catalysis in the presence of different ligands, and solvents.30–33 Further, it has been reported that other metals such Au,34 Rh,35 Cr,27 Cu,36,37 and V38 can be used to mediate the biaryl formation of arylboronic acids. However, in most of these reported systems, the catalysts are generally homogeneous, relatively expensive, and sensitive to moisture, compromised by low stability or selectivity, high catalyst loading, or toxicity. Therefore, the development of reusable, green, and efficient catalytic systems is highly attractive from the point of view of sustainable chemistry.
Among the transition metals, copper as a low-cost metal, abundant reserve, outstanding physical and chemical properties have drawn considerable attention in organic reactions. Recently synthesis of symmetrical biaryls has been reported using [CuI(bpy)]2,39 CuII–TRIPTA,40 CuNPs–rGO,41 and 2-OMG/Cu(OAc)2 (ref. 42) as green protocols. Nonetheless, so far only a few reports have appeared in which copper species act as catalysts for the synthesis of biaryls from the homocoupling of arylboronic acids, particularly through photocatalytic manners.
In continuation of our research into the development of novel catalytic methods for organic transformations43–45 we recently discovered that a TiO2–ascorbic acid (AA)–Cu(II), nanohybrid is active in the selective aerobic oxidation of alcohols.46 Herein, we wish to show the versatility of our previously reported catalyst and exploit its efficiency in the oxidative homocoupling of aryl boronic acids under heterogeneous conditions in air and visible light condition (Scheme 1). Furthermore, spectroscopic analysis and leaching experiments demonstrated the preservation of the structural integrity of the solid catalyst after six reaction cycles.
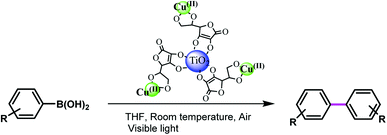 |
| Scheme 1 Homocouping of aryl boronic acids in the presence of TiO2–AA–Cu(II) nanohybrid. | |
2. Experimental
2.1. General procedure for homocoupling of arylboronic acids
To a mixture of aryl boronic acid (0.25 mmol) and TiO2–AA–Cu(II) nanohybrid (0.15 mol%) was added THF (0.5 ml) and the reaction mixture was stirred at ambient temperature under open air for 1 h in visible light (CFL light, 40 W). The reaction progress and the yields of the products were monitored by TLC and GC analysis (see ESI† for GC analysis conditions). The isolated products were obtained by plate chromatography eluted with n-hexane/ethyl acetate (10
:
1).
3. Result and discussion
TiO2–AA–Cu(II) nanohybrid were prepared and purified following the literature.46 The as-prepared nanohybrid was analyzed by FTIR, TEM, and TGA (see ESI, Fig. S1–S3†).
3.1. Catalytic activity
We started our study by exploring the reaction of phenylboronic acid (0.25 mmol) as a starting material to perform the optimization reaction by the screening of solvents, catalyst loading, and reaction time at room temperature in visible light irradiation. It should be noted that all experiments were investigated for 1 h in air condition. The results were summarized in Fig. 1. First, the effect of solvent was investigated. According to the experimental results, we found that the THF was better for the reaction. Various organic solvents, including DMSO, DMF, facilitated the coupling reaction with good yields. However, solvents such as ethyl acetate, ethanol, and water or solvent-free condition produced low yields (Fig. 1A). An investigation of the effect of the amount of solvent demonstrated that the 0.5 ml of THF was able to improve the coupling reaction by contrast with the other solvent amount (Fig. 1B). Further, the survey of the results for the catalyst dosage presented in Fig. 1C, revealed that the reaction proceeded well with low catalyst loading of 0.15 mol%, meanwhile, more catalysts decreased the reaction performance (Fig. 1C). Finally, the optimal condition for this homocoupling reaction was established by using 0.15 mol% of TiO2–AA–Cu(II) as a catalyst in THF (0.5 ml) as solvent at room temperature for 1 h, in visible light.
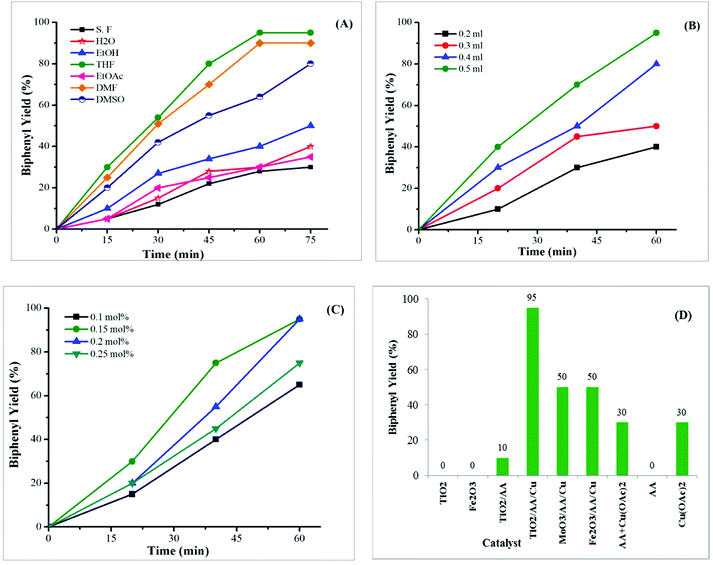 |
| Fig. 1 The screening of solvent (A), amount of solvent (B), amount of catalyst (C), and (D) comparison of various catalysts for the homocoupling reaction of phenylboronic acid (0.25 mmol) at ambient temperature in the open air and visible light condition. | |
Additionally, the ability of ascorbic acid, copper acetate, and a combination of ascorbic acid and copper acetate, as well as another metal oxide such as TiO2, Fe2O3 to promote the homocoupling of phenylboronic acid was then probed under optimized conditions (Fig. 1D). These compounds exhibited poor activity toward this reaction and the preferable catalytic performance of TiO2–AA–Cu(II) was confirmed.
Then we investigated the scope of the base-free homocoupling reaction concerning a variety of boronic acids. As shown in Table 1, these reactions, which took place under visible light (CFL light, 40 W), afforded good to excellent yields of the expected products according to the nature of boronic acid. The reaction conditions are notably compatible with a range of functional groups such as ethyl, alkoxy, aldehyde, halogen, or nitro on the aryl ring. Remarkably, all of the functional groups remain intact. Based on the results in Table 1, aryl boronic acids with electron-releasing substituents exhibited higher reactivity than their electron-poor counterparts. This was further supported by competition experiments in the presence of TiO2–AA–Cu(II) as a catalyst. For this purpose, we performed competition experiments with equivalent amounts of an electron-deficient aryl boronic acid (4-formyl phenylboronic acid) with an electron-releasing aryl boronic acid (4-methoxyphenyl boronic acid) (Scheme 2, reaction A) and an electron-neutral aryl boronic acids (Scheme 2, reaction B). As seen in Scheme 2, the homocoupling reaction of electron-deficient aryl boronic acids was significantly slower with less yield than electron releasing and electron-neutral counterparts. Also, using heteroaryl boronic acids such as thiophene-2-boronic acid and pyridine-4-ylboronic acid under optimal conditions confirmed the above observations (Table 1, entries 9 and 10). Obtained results of electronic effects of substituents showed that the aryl boronic acids with electron-releasing substituents increase the electron density at the active site during the formation of the transition state.39 To screen the steric effect, some substituted boronic acid in ortho, meta, and para position was examined using nitro-boronic acid derivatives (entries 5–7). The result showed the effect of sterically hindered ortho position caused to a lower yield than the other ones.
Table 1 Synthesis of biaryls in the presence of TiO2–AA–Cu(II)a
Aryl boronic |
Arylboronic acid |
Product |
Yieldb (%) |
Reaction condition: phenylboronic acid (0.25 mmol), catalyst (0.15 mol%), THF (0.5 ml) after 1 h in visible light (CFL light, 40 W). Isolated yield. The products were identified by comparison with authentic samples retention times of GC analysis and NMR spectra (ESI).39,41,47 |
1 |
 |
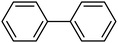 |
95 |
2 |
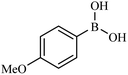 |
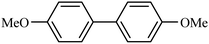 |
97 |
3 |
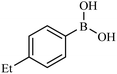 |
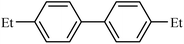 |
84 |
4 |
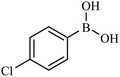 |
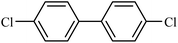 |
93 |
5 |
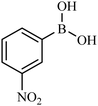 |
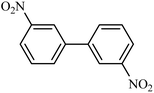 |
59 |
6 |
 |
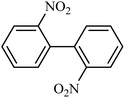 |
25 |
7 |
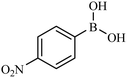 |
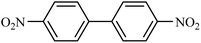 |
65 |
8 |
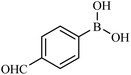 |
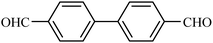 |
71 |
9 |
 |
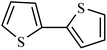 |
52 |
10 |
 |
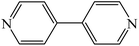 |
20 |
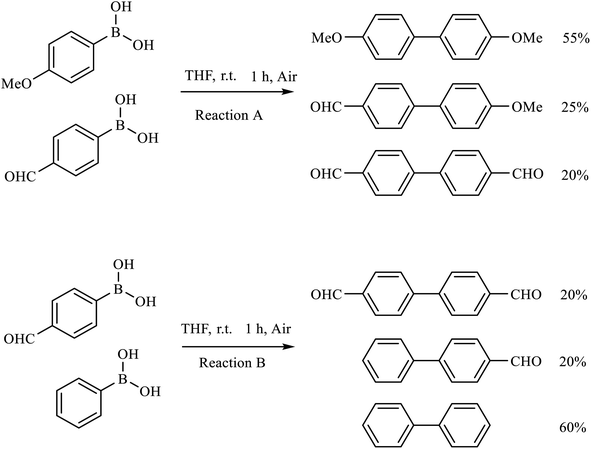 |
| Scheme 2 Competition experiments. The yield is based on GC analysis. | |
3.2. Photocatalytic activity
To screen the photocatalytic behavior of catalyst, the reaction of phenylboronic acid in optimum condition was examined by different sources of light. Fig. 2 shows the relative contributions of light and thermal processes to the conversion efficiencies in homocoupling reactions of aryl boronic acids (0.25 mmol) in the presence of TiO2–AA–Cu(II) nanohybrid (0.15 mol%). Survey the result exhibited without visible light irradiation, the yield of biaryl is 20%. It should be noted that the conversion of the reaction in the dark is regarded as the contribution of the thermal effect. We calculated the contributions of light irradiation to the conversion efficiency by subtracting the conversion of the reaction in the dark from the whole conversion observed when the system was irradiated with conditions identical. When the light sources were UV light (λ = 200–290 nm, 15 W), LED (λ = 505 nm, 12 W), sunlight (18 W), actinic BL lamp (λ = 366–400 nm, 15 W) and CFL lamps (compact fluorescent lamp, λ = 400–650 nm, 40 W), the light contributions for homocoupling of aryl boronic acid investigated (Fig. 2).
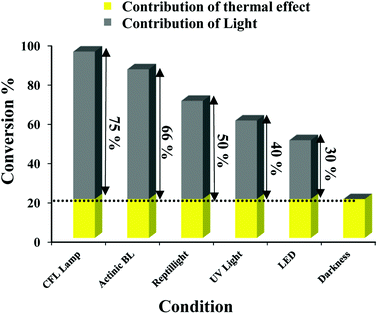 |
| Fig. 2 Dependence of the catalytic activity of the TiO2–AA–Cu(II) nanohybrid for the Homocoupling of aryl boronic acid on the irradiation wavelength. The numbers with percentages show the contribution of the light irradiation effect. Reaction conditions: aryl boronic acid (0.25 mmol), catalyst (0.15 mol%), THF (0.5 ml) after 1 h. | |
We can see that the CFL lamp has the greater contribution of irradiation to the overall conversion rate.
The results exhibit a light dependence of TiO2–AA–Cu(II) nanohybrid for homocoupling of aryl boronic acid. We then explored the dependence of the catalytic activity on the irradiation wavelength by a 40 W CFL lamp. The result is shown in Fig. 3. Without any filters, the irradiation of the light with wavelengths ranging from 400 to 800 nm gives a biaryl yield of 95%. The yield declines to 65%, 52%, and 37% when the wavelength range of the irradiation is 450–800, 550–800, and 600–800 nm, respectively. The contribution of 400–450 nm light accounts for about 40% ((75–45)/75 × 100%) in the total light-induced yield. Also, the light in the wavelength range of 450–550, 550–600, and 600–800 nm, respectively accounts for 17%, 20%, and 22% of the light-induced yield. These results confirm well the UV-visible absorption spectrum of the TiO2–AA–Cu(II) catalyst (Fig. 3).
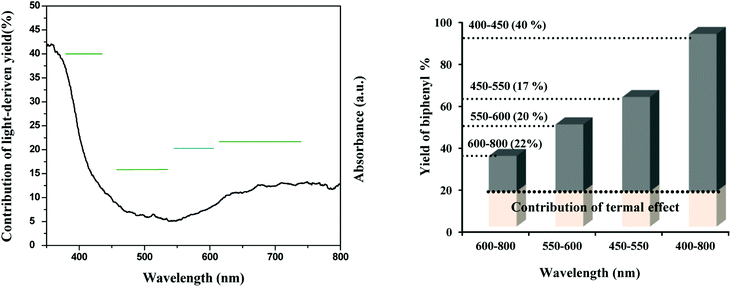 |
| Fig. 3 Dependence of the catalytic activity of TiO2–AA–Cu(II) for homocoupling of aryl boronic acid on the irradiation wavelength. | |
The photocatalytic performances of TiO2–AA–Cu(II) were also investigated by action spectra. To determine whether an observed reaction is induced by a photoinduced process or a thermocatalytic process. Indeed, action spectra represent the relationship between the wavelength of incident light and the photocatalytic rate. In this regard, the reaction rates of homocoupling of aryl boronic acid using TiO2–AA–Cu(II) under irradiation with different wavelengths were investigated. A good accordance between apparent quantum yield (AQY) with the diffuse reflectance spectrum of the TiO2–AA–Cu(II) nanohybrid was observed in homocoupling of phenylboronic acid (Fig. 4). These results convinced us that the reactions are performing in photo catalytically manner.
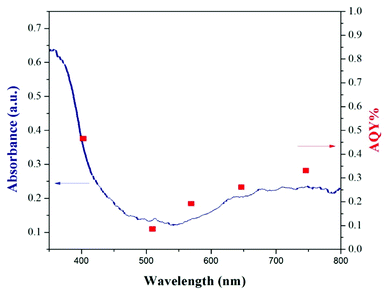 |
| Fig. 4 Action spectrum for homocoupling of aryl boronic acid using TiO2–AA–Cu(II) photocatalyst in the optimized reaction condition. The AQY versus the respective wavelengths of the reaction is plotted. AQY was calculated with the following equation: AQY (%) = [(Yvis − Ydark)/(photon number entered into the reaction vessel)] × 100, where Ylight and Ydark are the amounts of products formed under light irradiation and dark conditions, respectively. | |
3.3. Recyclability and stability of catalyst
For any heterogeneous catalyst, recyclability is the most important aspect. Hence, recoverability and reusability of the catalyst are taken into consideration where the choice to achieve environmentally sustainable and viable processes. Therefore, to test the reusability of the TiO2–AA–Cu(II) catalyst the homocoupling reaction of phenylboronic acid was investigated under the optimal reaction conditions. After completion of the reaction, the catalyst was separated from the reaction mixture by centrifugation followed by decantation with ethanol three times and dried in a vacuum oven before using for every next cycle. The catalyst proved to be reusable over six consecutive runs without significant loss of its activity (Fig. 5). Further, The ICP-AES analysis of the used catalyst after the 6th runs showed the amount of Cu in the reused catalyst is comparable with the fresh one which indicated the stability of catalyst even after recycling. The stability of the catalyst was also documented by recording the FTIR spectra of the used catalyst recovered from the model reaction (Fig. S4, ESI†). To evaluate the heterogeneity of catalyst, we performed a filtration test on model reaction. After 30 min (almost 50% conversion) the catalyst was filtered off and the filtrate was allowed to react further, but no further reaction was observed even after 4 h suggesting that the copper catalyst remains on the support during the reaction.
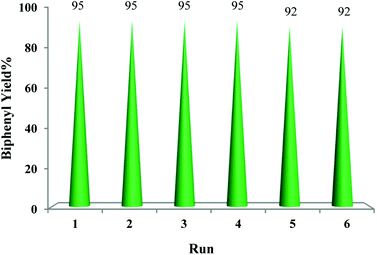 |
| Fig. 5 Recycling of the catalytic system for homocoupling reaction of phenylboronic acid using TiO2–AA–Cu(II) nanocatalyst. | |
Table 2 shows the merit of this operational protocol in comparison with those previously reported methods, in terms of catalyst loading, yield, and especially conditions used in the synthesis of biaryls using homocoupling of phenylboronic acid as a model substrate. Therefore, the title methodology is environmentally benign because of using an active bio-relevant catalyst (vitamin C), ambient temperature, air-condition, and finally green reaction media which qualify all requirements of an efficient catalytic system for applied objectives.
Table 2 Comparison of the oxidative activity of TiO2–AA–Cu(II) nanohybrid with other Cu based catalysts for the synthesis of biphenyl product using phenylboronic acid
Entry |
Catalyst |
Catalyst |
Conditions |
Time (h) |
Yield (%) |
Ref. |
Molecular sieve. β-Cyclodextrin. Fluorapatite. [{(phen)Cu(μ-OH)}2Cl2]. 1,4-Benzenedicarboxylic acid. 2,2′-Dipyridyl. |
1 |
TiO2–AA–Cu(II) |
0.15 mol% |
THF, r.t, air |
1 |
95 |
This work |
2 |
CuSO4-MSa |
80 mg |
DMF, 50 °C, air |
1 |
80 |
36 |
3 |
Cu(OAc)2 |
9 mg |
DMF, 100 °C, O2 |
3 |
72 |
37 |
4 |
CuCl |
1.5 mg |
MeOH, r.t |
3 |
72 |
47 |
5 |
Fe3O4–Cu2–β-CDb |
0.01 mmol |
DMF, 70 °C, air |
3 |
90 |
48 |
6 |
CuFAPc |
25 mg |
MeOH, r.t |
2 |
92 |
49 |
7 |
Phenanthrolined |
1.6 mg |
2-Propanol, 28 °C, air |
2 |
89 |
50 |
8 |
Cu(BDC)eMOF |
12 mg |
DMF, r.t, air |
16 |
97 |
51 |
9 |
[CuI(bpy)]2f |
5 mol% |
2-Propanol, K2CO3, r.t |
6 |
72 |
39 |
10 |
CuII-TRIPTA |
30 mg |
MeOH, 60 °C, air |
5 |
95 |
40 |
4. Conclusion
We have developed a mild, simple, and efficient protocol for the synthesis of symmetrical biaryl compounds through a homocoupling reaction of aryl boronic acids using TiO2–AA–Cu(II) catalyst through visible light. Good to excellent yields of the homocoupled product was obtained at ambient temperature in air and visible light condition. It is noteworthy, so far only a few reports have appeared in which copper species act as catalysts for the synthesis of biaryls from the homocoupling of arylboronic acids, particularly through photocatalytic manners. The as-prepared nanobio photocatalyst is efficient, easily recoverable, and reusable six times without losing its activity. Some advantages such as a green catalytic system, vitamin C as a natural source, high catalytic activity, use of readily available, inexpensive copper, easy separation, and excellent recyclability are salient features of this method. Our results provide a reliable method for configuring copper-complex containing vitamins and more opportunities for the discovery of simple and environmentally compatible catalysts in the general domain of heterogeneous photocatalytic applications in organic processes.
Conflicts of interest
The authors declare no conflict of interest.
Acknowledgements
Support for this work by the Research Council of the University of Birjand and ‘‘Iran National Science Foundation’’ (Grant No. 96013225) is highly appreciated.
References
- R. S. Varma, Green Chem., 2014, 16, 2027–2041 RSC.
- S. Trakhtenberg and J. C. Warner, Chem. Rev., 2007, 107, 2174–2182 CrossRef CAS PubMed.
- B. P. Mason, K. E. Price, J. L. Steinbacher, A. R. Bogdan and D. T. McQuade, Chem. Rev., 2007, 107, 2300–2318 CrossRef CAS PubMed.
- K. M. Buettner, J. M. Collins and A. M. Valentine, Inorg. Chem., 2012, 51, 11030–11039 CrossRef CAS PubMed.
- P. Brandão and S. Guieu, in Molecular Nutrition, Elsevier, 2020, pp. 33–49 Search PubMed.
- A. K. Renfrew, Metallomics, 2014, 6, 1324–1335 CrossRef CAS PubMed.
- K. Komeyama, R. Ohata, S. Kiguchi and I. Osaka, Chem. Commun., 2017, 53, 6401–6404 RSC.
- B. Zümreoglu-Karan, Coord. Chem. Rev., 2006, 250, 2295–2307 CrossRef.
- M. Martinek, M. Korf and J. Srogl, Chem. Commun., 2010, 46, 4387–4389 RSC.
- M. S. Refat, J. Mol. Struct., 2010, 969, 163–171 CrossRef CAS.
- A. R. Hajipour, M. Check and Z. Khorsandi, Appl. Organomet. Chem., 2017, 31, e3769 CrossRef.
- B. Y. Beker, T. Bakır, I. Sönmezoğlu, F. Imer and R. Apak, Chem. Phys. Lipids, 2011, 164, 732–739 CrossRef CAS PubMed.
- Y.-D. Park, Y.-J. Lyou, H.-S. Hahn, M.-J. Hahn and J.-M. Yang, J. Biomol. Struct. Dyn., 2006, 24, 131–138 CrossRef CAS PubMed.
- S. N. S. Vasconcelos, J. S. Reis, I. M. de Oliveira, M. N. Balfour and H. A. Stefani, Tetrahedron, 2019, 75, 1865–1959 CrossRef CAS.
- D. A. Horton, G. T. Bourne and M. L. Smythe, Chem. Rev., 2003, 103, 893–930 CrossRef CAS PubMed.
- G. Bringmann, T. Gulder, T. A. M. Gulder and M. Breuning, Chem. Rev., 2011, 111, 563–639 CrossRef CAS PubMed.
- G. Wu, H.-F. Guo, K. Gao, Y.-N. Liu, K. F. Bastow, S. L. Morris-Natschke, K.-H. Lee and L. Xie, Bioorg. Med. Chem. Lett., 2008, 18, 5272–5276 CrossRef CAS PubMed.
- F. V. Singh, R. Vatsyayan, U. Roy and A. Goel, Bioorg. Med. Chem. Lett., 2006, 16, 2734–2737 CrossRef CAS PubMed.
- Y. Uozumi and Y. Nakai, Org. Lett., 2002, 4, 2997–3000 CrossRef CAS PubMed.
- R. Lebeuf, J. Dunet, R. Beniazza, D. Ibrahim, G. Bose, M. Berlande, F. Robert and Y. Landais, J. Org. Chem., 2009, 74, 6469–6478 CrossRef CAS PubMed.
- H.-C. Zhang, W.-S. Huang and L. Pu, J. Org. Chem., 2001, 66, 481–487 CrossRef CAS PubMed.
- M. Tamura and H. Fujihara, J. Am. Chem. Soc., 2003, 125, 15742–15743 CrossRef CAS PubMed.
- R. Letrun, M. Koch, M. L. Dekhtyar, V. V Kurdyukov, A. I. Tolmachev, W. Rettig and E. Vauthey, J. Phys. Chem. A, 2013, 117, 13112–13126 CrossRef CAS PubMed.
- G. Zheng, M. Tian, Y. Xu, X. Chen and X. Li, Org. Chem. Front., 2018, 5, 998–1002 RSC.
- A. F. Lee, P. J. Ellis, I. J. S. Fairlamb and K. Wilson, Dalton Trans., 2010, 39, 10473–10482 RSC.
- J. Tsuji, Palladium Reagents and Catalysts: New Perspectives for the 21st Century, John Wiley & Sons, 2006 Search PubMed.
- J. R. Falck, S. Mohapatra, M. Bondlela and S. K. Venkataraman, Tetrahedron Lett., 2002, 43, 8149–8151 CrossRef CAS.
- A. R. Kapdi, G. Dhangar, J. L. Serrano, J. A. De Haro, P. Lozano and I. J. S. Fairlamb, RSC Adv., 2014, 4, 55305–55312 RSC.
- R. Luque, B. Baruwati and R. S. Varma, Green Chem., 2010, 12, 1540–1543 RSC.
- K. Mitsudo, T. Shiraga, D. Kagen, D. Shi, J. Y. Becker and H. Tanaka, Tetrahedron, 2009, 65, 8384–8388 CrossRef CAS.
- B. Mu, T. Li, Z. Fu and Y. Wu, Catal. Commun., 2009, 10, 1497–1501 CrossRef CAS.
- H. Lakmini, I. Ciofini, A. Jutand, C. Amatore and C. Adamo, J. Phys. Chem. A, 2008, 112, 12896–12903 CrossRef CAS PubMed.
- C. Amatore, C. Cammoun and A. Jutand, Eur. J. Org. Chem., 2008, 2008, 4567–4570 CrossRef.
- M. P. Sk, C. K. Jana and A. Chattopadhyay, Chem. Commun., 2013, 49, 8235–8237 RSC.
- T. Vogler and A. Studer, Adv. Synth. Catal., 2008, 350, 1963–1967 CrossRef CAS.
- B. Kaboudin, T. Haruki and T. Yokomatsu, Synthesis, 2011, 2011, 91–96 CrossRef.
- A. S. Demir, Ö. Reis and M. Emrullahoglu, J. Org. Chem., 2003, 68, 10130–10134 CrossRef CAS PubMed.
- H. Mizuno, H. Sakurai, T. Amaya and T. Hirao, Chem. Commun., 2006, 5042–5044 RSC.
- B.-F. Long, G.-F. Qin, Q. Huang, T. Xiong, Y. Mi, F.-L. Hu and X.-H. Yin, J. Iran. Chem. Soc., 2019, 16, 2639–2646 CrossRef CAS.
- S. K. Das, B. K. Chandra, R. A. Molla, M. Sengupta, S. M. Islam, A. Majee and A. Bhaumik, Mol. Catal., 2020, 480, 110650 CrossRef CAS.
- N. Hussain, P. Gogoi, V. K. Azhaganand, M. V Shelke and M. R. Das, Catal. Sci. Technol., 2015, 5, 1251–1260 RSC.
- C. Yuan, L. Zheng and Y. Zhao, Molecules, 2019, 24, 3678 CrossRef PubMed.
- F. Feizpour, M. Jafarpour and A. Rezaeifard, Catal. Lett., 2018, 148, 30–40 CrossRef CAS.
- M. Jafarpour, A. Rezaeifard and F. Feizpour, ChemistrySelect, 2017, 2, 2901–2909 CrossRef CAS.
- F. Feizpour, M. Jafarpour and A. Rezaeifard, Catal. Lett., 2019, 149, 1595–1610 CrossRef CAS.
- N. Pourmorteza, M. Jafarpour, F. Feizpour and A. Rezaeifard, RSC Adv., 2020, 10, 12053–12059 RSC.
- G. Cheng and M. Luo, Eur. J. Org. Chem., 2011, 2011, 2519–2523 CrossRef.
- B. Kaboudin, R. Mostafalu and T. Yokomatsu, Green Chem., 2013, 15, 2266–2274 RSC.
- S. A. R. Mulla, S. S. Chavan, M. Y. Pathan, S. M. Inamdar and T. M. Y. Shaikh, RSC Adv., 2015, 5, 24675–24680 RSC.
- N. Kirai and Y. Yamamoto, Eur. J. Org. Chem., 2009, 2009, 1864–1867 CrossRef.
- P. Puthiaraj, P. Suresh and K. Pitchumani, Green Chem., 2014, 16, 2865–2875 RSC.
Footnote |
† Electronic supplementary information (ESI) available. See DOI: 10.1039/d1ra07252h |
|
This journal is © The Royal Society of Chemistry 2022 |
Click here to see how this site uses Cookies. View our privacy policy here.