DOI:
10.1039/D1RA07835F
(Paper)
RSC Adv., 2022,
12, 4924-4930
K-doped Li2ZnTi3O8/C as an efficient anode material with high performance for Li-ion batteries
Received
23rd October 2021
, Accepted 4th January 2022
First published on 9th February 2022
Abstract
Li2ZnTi3O8/C and Li1.9K0.1ZnTi3O8/C were successfully synthesized using the sol–gel method. Doping K apparently yielded a wider tunnel, helpful for increasing the rate of transport of lithium ions, and furthermore yielded excellent electrochemical properties. The first discharge capacity for Li1.9K0.1ZnTi3O8/C was 352.9 mA h g−1 at a current density of 200 mA g−1. Li1.9K0.1ZnTi3O8/C also performed stably, retaining a capacity of 323.7 mA h g−1 at the 100th cycle, indicative of its excellent cycling properties. In the rate performance test, Li1.9K0.1ZnTi3O8/C showed at the first cycle a high discharge capacity of 379.5 mA h g−1 for a current density of 50 mA g−1 and a capacity of 258.9 mA h g−1 at 1000 mA g−1. The results indicated that K-doping should be considered a useful method for improving electrochemical performances.
1 Introduction
Lithium-ion batteries (LIBs) are effective batteries because of their high specific energy levels, small volumes, long cycling lifetimes and high working voltages,1–3 and have been used in diverse applications. The anode material, as one of the important components of the LIB, directly affects the electrochemical performance of the battery. Cubic Li2ZnTi3O8 (LZTO) has been investigated as a potential anode material due to its lack of toxicity, high safety, low cost, relatively high theoretical capacity and low discharge voltage plateau of approximately 0.5 V (vs. Li/Li+).4–6 But its poor electronic conductivity has restricted its practical applications.7–10 Therefore, finding methods to improve the electronic and ionic conductivities of LZTO is extremely important.
To overcome such defects, methods such as carbon coating, improving the preparation process, and doping diverse ions have proved effective.11–22 Carbon materials have great advantages, such as low price, high natural abundance, excellent conductivity, low amounts required for using them to modify other materials, stable physical and chemical properties, etc., which could not only endow the matrix materials with high conductivity, but also reduce the amounts of side reactions between electrode materials and electrolyte. Therefore, carbon inclusion is considered a convenient modification method to improve the electrical conductivity of electrode materials.20,23,24 Producing small, i.e., nano-sized, particles of lithium ions can promote the increase of charge–discharge time, and is also conducive to the electrolyte infiltration of the material.25 The ionic conductivity and electronic conductivity of LZTO can be improved by doping it with ions, and the location and amount of doping affects the electrochemical properties of electrode materials.11,26–28 The ions currently used for doping lithium zinc titanate mainly include Na+,29 Ag+,30 Al3+,31 Ce4+,10 and V5+.32
Na+ is considered a promising doping element due to its high abundance and environmental friendliness. Chen et al.29 has synthesized Na+-doped Li2ZnTi3O8 using the solid state method. Their experiments showed the capacity of Li1.95Na0.05ZnTi3O8 reaching as high as 267.3 mA h g−1 at the 50th cycle when using a current density of 0.1 A g−1. Of the materials they tested, it was shown to display the highest reversible capacity, mainly due to the diameter of Na+ being greater than that of Li+, thus enlarging the diffusion channel of Li+.
Tang et al.31 used the solid phase method to add Al2O3 into Li2ZnTi3O8 to realize the doping with Al3+. Their experimental results showed capacity retentions of 99.8%, 91.6%, 86.6% and 93.4% obtained for Li2ZnTi2.9Al0.1O8 at the 100th cycle for current densities of 0.5 A g−1, 1.0 A g−1, 2.0 A g−1 and 3.0 A g−1, respectively. Therefore, the doping of Al3+ was found to significantly improve the capacity and rate performance of Li2ZnTi3O8.
Yi et al.32 used V2O5 as a raw material to synthesize V5+-doped Li2ZnTi3O8 by carrying out a simple solid-phase method in one step. V5+ was found to replace some of the Li+ ions and in this way improve the diffusion rate of lithium ions. The reversible capacities of Li1.95V0.05ZnTi3O8 at rates of 0.2, 1.0, 2.0 and 5.0C were measured to be, respectively, 213.3, 171.2, 132.5 and 84.7 mA h g−1 values higher than those measured for pure Li2ZnTi3O8 (184.5, 129.5, 107.3 and 24 mA h g−1). Qie et al.33 studied the influence of Cu2+ doping on the electrochemical properties of Li2ZnTi3O8 and found that Li1.95Zn0.9Cu0.1Ti3O8 displayed the best electrochemical performance.
K is similar to Li in chemical properties and is a promising doping atom. However, to the best of our knowledge, it has not been used to dope Li2ZnTi3O8. Therefore, the electrochemical reaction of Li1.9K0.1ZnTi3O8/C was studied using the sol–gel method in the current work, and the results showed its excellent electrochemical performance, demonstrating that K-doping should in general be considered a useful method for improving electrochemical performances.
2 Materials and methods
Li1.9K0.1ZnTi3O8/C (0.02 mol) was synthesized using the sol–gel method. To do so, 4.7922 g TiO2 (99.8%, Shanghai Maclin Biochemical Technology Co., Ltd), 4.39 g (CH3COO)2Zn·2H2O (AR, Chengdu Cologne Chemical Reagent Co., Ltd), 2.5076 g (CH3COO)Li (99.9%, Shanghai Maclin Biochemical Technology Co., Ltd), 4 g polyvinylpyrrolidone K30 (PVP K30) (AR, Chengdu Cologne Chemical Reagent Co., Ltd), and 0.1491 g KCl (AR, Chengdu Cologne Chemical Reagent Co., Ltd) were added into a sample of anhydrous ethanol. Then the resulting mixture was dried at 80 °C for 4 h, and the dried material was heated at 750 °C for 15 minutes in a microwave sintering furnace to achieve the final Li1.9K0.1ZnTi3O8/C electrode material. For purposes of comparison, Li2ZnTi3O8/C was prepared using the same protocol except that KCl was not included.
X-ray diffraction (XRD) patterns were acquired using a Brook AXS's D2 PHASER, with a 2θ range of 10–80°. Scanning electron microscopy (SEM) images were acquired using a TESCAN VEGA3 apparatus. X-ray photoelectron spectroscopy (XPS) was carried out using K-Kα radiation as the excitation source. Data for high-resolution transmission electron microscopy (HR-TEM) and TEM-EDX mapping were collected using an FEI Talos F200X apparatus. Raman spectra were recorded using a DXR Raman spectrometer (Thermo Scientific) at room temperature with a 780 nm excitation line using an Ar+ laser.
The electrochemical performance of Li1.9K0.1ZnTi3O8/C was assessed with lithium metal as the counter electrode. Li1.9K0.1ZnTi3O8/C, Super-P and sodium carboxymethylcellulose (CMC) with a weight ratio of 80
:
10
:
10 were added into water, and the resulting electrode slurry was spread on a copper current collector and dried at 80 °C for 8 h in a vacuum oven. The working electrode was prepared by following the above steps. CR2032 coin cells were prepared in an Ar-filled glovebox. All electrochemical properties were characterized between 0.05 and 3.0 V at 25 °C, and the EIS data were collected at frequencies ranging from 0.1 Hz to 100 kHz.
3 Results and discussion
Fig. 1(A) shows the XRD patterns of the materials investigated. All of the samples were determined using these patterns to have formed the spinel LZTO structure, indicating that the low dosage of K+ did not alter the structure. As shown in Table 1, the cell parameters of Li2ZnTi3O8/C and Li1.9K0.1ZnTi3O8/C were determined to be, respectively, a = 8.4112 and 8.5032 Å with the greater cell length for Li1.9K0.1ZnTi3O8/C due to the introduction of K+ into the crystal structure resulting in a replacement of some of the Li+ ions (ionic radius 0.076 Å) with the larger K+ ions (ionic radius 1.38 Å).34,35 This difference was perhaps associated with a wider tunnel for Li1.9K0.1ZnTi3O8/C, a feature apt to increase the speed of Li+ transport and further improve the electrochemical properties of the resulting electrode material.36 Fig. 1(B) shows the XRD patterns of Li1.9K0.1ZnTi3O8/C before and after 100 cycles. In these patterns, the diffraction peaks were essentially identical, demonstrating that the structure of Li1.9K0.1ZnTi3O8/C was very well maintained after 100 cycles.
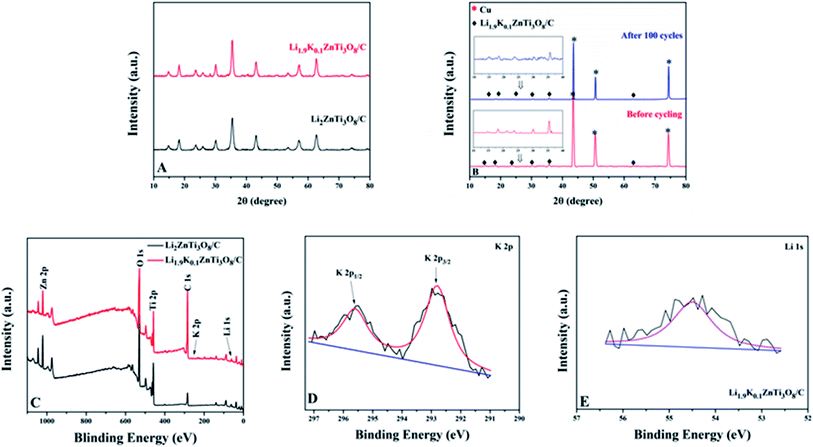 |
| Fig. 1 (A) XRD patterns of Li2ZnTi3O8/C and Li1.9K0.1ZnTi3O8/C. (B) XRD patterns of Li1.9K0.1ZnTi3O8/C before and after 100 cycles. (C) XPS survey spectra of Li2ZnTi3O8/C and Li1.9K0.1ZnTi3O8/C. (D) Li1.9K0.1ZnTi3O8/C XPS spectrum in the K 2p region. (E) Li2ZnTi3O8/C and Li1.9K0.1ZnTi3O8/C XPS spectra in the Li 1s region. | |
Table 1 Crystal lattice parameter values for Li2ZnTi3O8/C and Li1.9K0.1ZnTi3O8/C
Samples |
Lattice parameters |
a (Å) |
V (Å3) |
Li1.9K0.1ZnTi3O8/C |
8.5032 |
614.8189 |
Li2ZnTi3O8/C |
8.4112 |
595.0780 |
More information was obtained by taking XPS measurements. Fig. 1(C) shows the XPS survey spectrum of Li1.9K0.1ZnTi3O8/C; peaks corresponding to Li 1s, O 1s, C 1s, Zn 2p, Ti 2p, and K 2p were identified in this spectrum. Peaks observed at about 292.78 and 295.58 eV (Fig. 1(D)) appeared to correspond well to K 2p3/2 and K 2p1/2,37 verifying the presence of K+ in the Li1.9K0.1ZnTi3O8/C sample tested. The peak observed at about 54.48 eV in the spectrum of the Li1.9K0.1ZnTi3O8/C sample (Fig. 1(E)) can be attributed to Li 1s.37
The SEM images (Fig. 2(A) and (B)) acquired of the Li2ZnTi3O8/C and Li1.9K0.1ZnTi3O8/C samples indicated that they were well crystallized. Note that the particle morphology did not obviously change after the doping with the low dose of K+. More detailed structural information was gained from the TEM images of the Li2ZnTi3O8/C and Li1.9K0.1ZnTi3O8/C composites (Fig. 2(C) and (D)). And as seen in Fig. 2(E) and (F), no obvious difference between the lattice fringes of these composites was observed, consistent with the XRD results. The results demonstrated that the crystal structure of the composite did not change upon it being doped with small amounts of K. To further investigate the doping into Li2ZnTi3O8/C, Li1.9K0.1ZnTi3O8/C was examined using energy dispersive spectroscopy (EDS) mapping. From the EDS mappings, the elements C (Fig. 2(G)), Ti (Fig. 2(H)), Zn (Fig. 2(I)) and K (Fig. 2(J)) were seen on the surface of Li1.9K0.1ZnTi3O8/C.
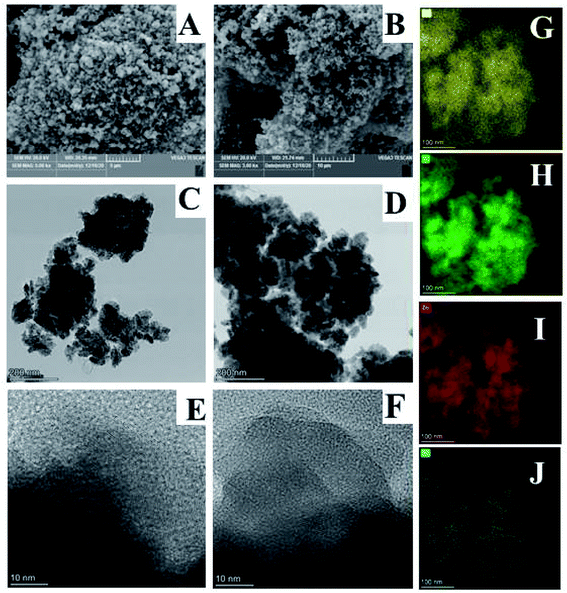 |
| Fig. 2 (A and B) SEM images of (A) Li2ZnTi3O8/C and (B) Li1.9K0.1ZnTi3O8/C. (C and D) Typical TEM images of (C) Li2ZnTi3O8/C and (D) Li1.9K0.1ZnTi3O8/C. (E and F) HRTEM images of (E) Li2ZnTi3O8/C and (F) Li1.9K0.1ZnTi3O8/C. (G–J) EDS mappings of Li1.9K0.1ZnTi3O8/C for the elements (G) C, (H) Ti, (I) Zn, and (J) K. | |
The pore size distributions determined using the Barrett–Joyner–Halenda (BJH) method are shown in Fig. 3(A) and (B) (inset). The average diameters of the pores of Li2ZnTi3O8/C and Li1.9K0.1ZnTi3O8/C were found to be 10.26 nm and 11.13 nm, respectively. From the data obtained, more pores were identified in the Li1.9K0.1ZnTi3O8/C material than in the Li2ZnTi3O8/C material, which indicated that the degree of agglomeration was reduced and that more tunnels for Li+ conduction were provided by Li1.9K0.1ZnTi3O8/C.
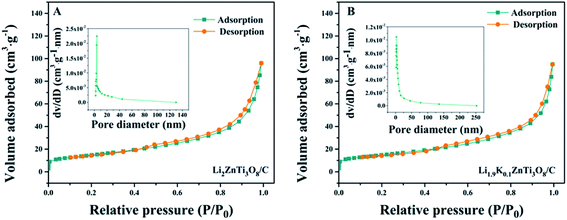 |
| Fig. 3 Nitrogen adsorption–desorption isotherms of (A) Li2ZnTi3O8/C and (B) Li1.9K0.1ZnTi3O8/C with the corresponding pore diameter distributions in the insets. | |
To verify the presence of carbon in the synthesized Li2ZnTi3O8/C and Li1.9K0.1ZnTi3O8/C samples, Raman spectra were acquired, as shown in Fig. 4. From the spectra of Li2ZnTi3O8/C and Li1.9K0.1ZnTi3O8/C, the D band (at ca.1330 cm−1) and G band (at ca.1560 cm−1) were identified, demonstrating the presence of carbon in the Li2ZnTi3O8/C and Li1.9K0.1ZnTi3O8/C samples.38
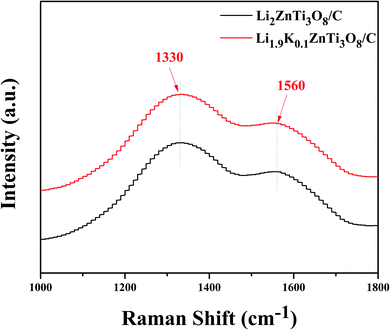 |
| Fig. 4 Raman spectra of Li2ZnTi3O8/C and Li1.9K0.1ZnTi3O8/C. | |
The electrochemical reaction mechanisms for Li2ZnTi3O8/C and Li1.9K0.1ZnTi3O8/C were investigated using cyclic voltammetry (CV). These tests were performed using a scan rate of 0.1 mV s−1 and potentials between 0.05 V and 3 V (Fig. 5 (A) and (B)). The anodic peak potential (φPa), cathodic peak potential (φPc) and the difference between them (ΔφP) are shown in Table 2. Li2ZnTi3O8/C and Li1.9K0.1ZnTi3O8/C showed cathodic and anodic peaks at potentials between 1 V and 2 V, which we interpreted as involving the participation of the reactions of the Ti4+/Ti3+ redox couple, and indicated that applying a low dose of K-doping did not change the Li2ZnTi3O8/C electrochemical process.39 As shown in Table 2, the difference between the potentials of the oxidation and reduction peaks was 151 mV for the Li2ZnTi3O8/C sample, but considerably less, at 97 mV, for the Li1.9K0.1ZnTi3O8/C sample. Compared with Li2ZnTi3O8/C, Li1.9K0.1ZnTi3O8/C has been shown to display higher lithium-ion diffusion rates and lower polarization.40 Therefore, the reversibility and cycling abilities of the electrode materials were enhanced by the low-dose doping of Li2ZnTi3O8/C.
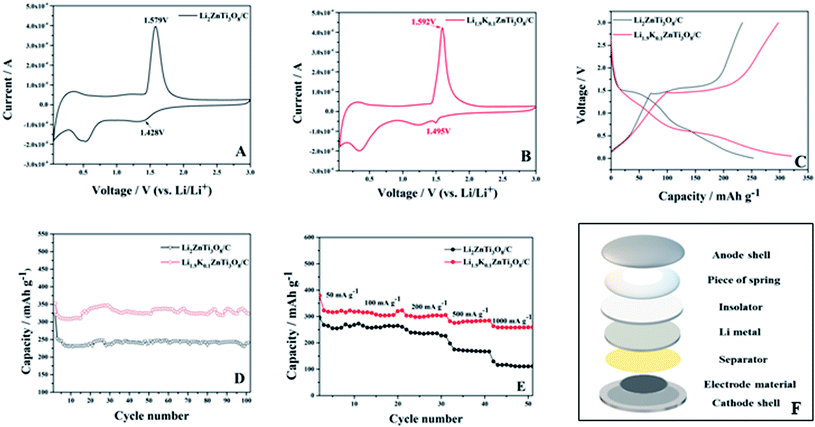 |
| Fig. 5 (A and B) CV curves of (A) Li2ZnTi3O8/C and (B) Li1.9K0.1ZnTi3O8/C from 0.05 V to 3.0 V. (C) Initial charge–discharge curves of Li2ZnTi3O8/C and Li1.9K0.1ZnTi3O8/C. (D) Cycling capacity of Li2ZnTi3O8/C and Li1.9K0.1ZnTi3O8/C for 100 cycles. (E) Rate performances of Li2ZnTi3O8/C and Li1.9K0.1ZnTi3O8/C at different current densities. (F) The composition of the CR2032 button battery. | |
Table 2 Electrochemical parameters of investigated samples, including the difference between the potentials of the anodic and cathodic peaks from CV
Sample |
φPa (V) |
φPc (V) |
ΔφP (mV) |
Li1.9K0.1ZnTi3O8/C |
1.592 |
1.495 |
97 |
Li2ZnTi3O8/C |
1.579 |
1.428 |
151 |
The CV curves for the first cycle at 200 mA g−1 for potentials of 0.05–3 V are shown in Fig. 5(C). Here, the discharge capacity of Li1.9K0.1ZnTi3O8/C was higher than that of Li2ZnTi3O8/C at the 1st cycle. The voltage of the discharge platform was 0.57 V (vs. Li+/Li), the voltage of charging platform was 1.48 V (vs. Li+/Li), and the voltage difference between charge and discharge platform was the smallest, indicating that the polarization of the synthesized material was small during Li+ disintercalation.41
The cycling properties of Li2ZnTi3O8/C and Li1.9K0.1ZnTi3O8/C were characterized at 200 mA g−1 (Fig. 5(D)). At the 100th cycle, 75.06% and 91.73% (323.7 mA h g−1) of the initial capacities were retained for Li2ZnTi3O8/C and Li1.9K0.1ZnTi3O8/C, respectively. Chen et al.29 synthesized Li1.95Na0.05ZnTi3O8, and it reached 267.3 mA h g−1 at the 50th cycle at 0.1 A g−1, indicating a better cycling performance resulting from K+ doping than from Na+ doping. Therefore, Li1.9K0.1ZnTi3O8/C was indicated to demonstrate a better capacity retention and cycling performance than Li2ZnTi3O8/C, which could be attributed to the low-dose K+ doping enlarging the Li+ transport tunnels, and hence increasing the rates of Li+ transport and electron transport.36
The rate properties of Li2ZnTi3O8/C and Li1.9K0.1ZnTi3O8/C are compared in Fig. 5(E). Li1.9K0.1ZnTi3O8/C delivered a maximum discharge capacity of 379.5 mA h g−1 at the 1st cycle for a current density of 50 mA g−1, and discharge capacities of 322.9, 305.5, 284.5, and 258.9 mA h g−1 for 100, 200, 500, and 1000 mA g−1, respectively, whereas Li2ZnTi3O8/C delivered capacities of 260.6, 226.2, 166.9, and 111.4 mA h g−1 at these current densities, respectively. Li1.9K0.1ZnTi3O8/C showed better rate properties than did Li2ZnTi3O8/C, which may be attributed to (1) doping K possibly having enhanced the electronic conductivity and yielding a good rate performance for Li1.9K0.1ZnTi3O8/C, and (2) the increased cell volume after low-dose K doping possibly increasing the rates of Li+ transport and electron transport.42,43
EIS measurements for the samples were taken as shown in Fig. 6. The corresponding plots were composed of a semicircle at high frequency and an inclined line at the low-frequency region, indicating the charge transfer resistance and Li+ diffusion process within the electrodes, respectively.44,45 As shown in Fig. 6, the charge-transfer resistance of the Li1.9K0.1ZnTi3O8/C was found to be lower than that of Li2ZnTi3O8/C, demonstrating low-dose K+ doping to be a useful method for enhancing the electronic conductivity.46,47 In addition, according to the data in the low-frequency regions, the Li+ diffusion rate of the Li1.9K0.1ZnTi3O8/C sample was slightly higher than that of the Li2ZnTi3O8/C sample.
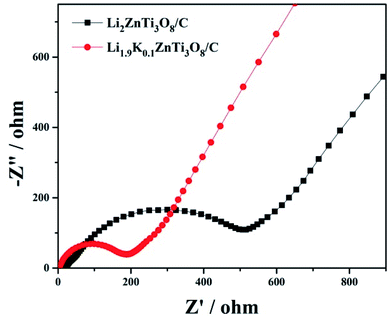 |
| Fig. 6 EIS results for Li2ZnTi3O8/C and Li1.9K0.1ZnTi3O8/C. | |
4 Conclusions
In this study, an XRD analysis indicated no change in the structure of Li2ZnTi3O8/C resulting from introducing into it a low dose of K. In addition, a low dose of K doped into Li2ZnTi3O8/C was good for enhancing the electronic and ionic conductivity levels, which could improve the electrochemical performance. The initial discharge capacity was 352.9 mA h g−1 (Li1.9K0.1ZnTi3O8/C) at 200 mA g−1. The sample performed stably, with a capacity of 323.7 mA h g−1 retained for Li1.9K0.1ZnTi3O8/C after 100 cycles. In the rate test, Li1.9K0.1ZnTi3O8/C showed a high discharge capacity of 379.5 mA h g−1 at the 1st cycle for a current density of 50 mA g−1 and a capacity of 258.9 mA h g−1 for 1000 mA g−1. Of the various materials tested, Li1.9K0.1ZnTi3O8/C exhibited the best rate properties and excellent cycling stability. Therefore, K-doping should be considered a useful method for improving electrochemical performances.
Funding
This work was financially supported by the Demonstration Project of Scientific and Technological Achievements Transfer and Transformation of Sichuan Province (2021ZHCG0040).
Author contributions
X.-G. Zeng and J. Peng contributed to the conception and design of the study. H.-F. Zhu and K. Xia organized the database. J. Peng and J. Gong wrote the first draft of the manuscript. X.-G. Zeng revised the whole manuscript.
Conflicts of interest
The authors declare that the research was conducted in the absence of any commercial or financial relationships that could be construed as a potential conflict of interest.
References
- T. Liu, H. Tang and J. Liu, et al., Improved electrochemical performance of Li2ZnTi3O8 using carbon materials as loose and porous agent, Electrochim. Acta, 2018, 259, 28–35 CrossRef CAS.
- T. Liu, H. Tang and L. Zan, et al., Comparative study of Li2ZnTi3O8 anode material with good high rate capacities prepared by solid state, molten salt and sol–gel methods, J. Electroanal. Chem., 2016, 771, 10–16 CrossRef CAS.
- G. Li, X. Chen and Y. F. Liu, et al., One-time sintering process to synthesize ZrO2-coated LiMn2O4 materials for lithium-ion batteries, RSC Adv., 2018, 8, 16753–16761 RSC.
- C. An, C. Li and H. Tang, et al., Binder-free flexible Li2ZnTi3O8@MWCNTs stereoscopic network as lightweight and superior rate performance anode for lithium-ion batteries, J. Alloys Compd., 2020, 816, 152580 CrossRef CAS.
- C. Chen, Z. Li and B. Xu, Surface modification of Li2ZnTi3O8 with the C&N layer for lithium-ion batteries, Mater. Chem. Phys., 2020, 245 Search PubMed.
- N. Firdous, N. Arshad and S. B. Simonsen, et al., Advanced electrochemical investigations of niobium modified Li2ZnTi3O8 lithium ion battery anode materials, J. Power Sources, 2020, 462, 228186 CrossRef CAS.
- C. Chen, C. Ai and Y. He, et al., High performance Li2ZnTi3O8 coated with N-doped carbon as an anode material for lithium-ion batteries, J. Alloys Compd., 2017, 705, 438–444 CrossRef CAS.
- C. Chen, C. Ai and X. Liu, Ti(III) self-doped Li2ZnTi3O8 as a superior anode material for Li-ion batteries, Electrochim. Acta, 2018, 265, 448–454 CrossRef CAS.
- C. Chen, C. Ai and X. Liu, et al., High performance Li2ZnTi3O8@C anode material fabricated by a facile method without an additional carbon source, J. Alloys Compd., 2017, 698, 692–698 CrossRef CAS.
- C. Chen, C. Ai and X. Liu, et al., Advanced electrochemical properties of Ce-modified Li2ZnTi3O8 anode material for lithium-ion batteries, Electrochim. Acta, 2017, 227, 285–293 CrossRef CAS.
- Y. J. Bai, C. Gong and Y. X. Qi, et al., Excellent long-term cycling stability of La-doped Li4Ti5O12 anode material at high current rates, J. Mater. Chem., 2012, 22, 19054 RSC.
- Y. R. Jhan and J. G. Duh, Electrochemical performance and low discharge cut-off voltage behavior of ruthenium doped Li4Ti5O12 with improved energy density, Electrochim. Acta, 2012, 63, 9–15 CrossRef CAS.
- C. Y. Lin, Y. R. Jhan and J. G. Duh, Improved capacity and rate capability of Ru-doped and carbon-coated Li4Ti5O12 anode material, J. Alloys Compd., 2011, 509, 6965–6968 CrossRef CAS.
- V. Mani, K. Nathiya and N. Kalaiselvi, Role of carbon content in qualifying Li3V2(PO4)3/C as a high capacity anode for high rate lithium battery applications, RSC Adv., 2014, 4, 17091–17096 RSC.
- Y. Qi, Y. Huang and D. Jia, et al., Preparation and characterization of novel spinel Li4Ti5O12−xBrx anode materials, Electrochim. Acta, 2009, 54, 4772–4776 CrossRef CAS.
- A. Y. Shenouda and K. R. Murali, Electrochemical properties of doped lithium titanate compounds and their performance in lithium rechargeable batteries, J. Power Sources, 2008, 176, 332–339 CrossRef CAS.
- B. Tian, H. Xiang and L. Zhang, et al., Niobium doped lithium titanate as a high rate anode material for Li-ion batteries, Electrochim. Acta, 2010, 55, 5453–5458 CrossRef CAS.
- S. Wang, L. Wang and Z. Meng, et al., Li2ZnTi3O8/graphene nanocomposite as a high-performance anode material for lithium-ion batteries, RSC Adv., 2018, 8, 31628–31632 RSC.
- H. Wu, S. Chang and X. Liu, et al., Sr-doped Li4Ti5O12 as the anode material for lithium-ion batteries, Solid State Ionics, 2013, 232, 13–18 CrossRef CAS.
- Y. Xu, Z. Hong and L. Xia, et al., One step sol–gel synthesis of Li2ZnTi3O8/C nanocomposite with enhanced lithium-ion storage properties, Electrochim. Acta, 2013, 88, 74–78 CrossRef CAS.
- Z. Yu, X. Zhang and G. Yang, et al., High rate capability and long-term cyclability of Li4Ti4.9V0.1O12 as anode material in lithium ion battery, Electrochim. Acta, 2011, 56, 8611–8617 CrossRef CAS.
- B. Zhang, Z. D. Huang and S. W. Oh, et al., Improved rate capability of carbon coated Li3.9Sn0.1Ti5O12 porous electrodes for Li-ion batteries, J. Power Sources, 2011, 196, 10692–10697 CrossRef CAS.
- H. Tang and Z. Tang, Effect of different carbon sources on electrochemical properties of Li2ZnTi3O8/C anode material in lithium-ion batteries, J. Alloys Compd., 2014, 613, 267–274 CrossRef CAS.
- L. Zeng, C. Zheng and L. Xia, et al., Ordered mesoporous TiO2–C nanocomposite as an anode material for long-term performance lithium-ion batteries, J. Mater. Chem. A, 2013, 1, 4293 RSC.
- L. Wang, L. Wu and Z. Li, et al., Synthesis and electrochemical properties of Li2ZnTi3O8 fibers as an anode material for lithium-ion batteries, Electrochim. Acta, 2011, 56, 5343–5346 CrossRef CAS.
- W. Fang, X. Cheng and P. Zuo, et al., A facile strategy to prepare nano-crystalline Li4Ti5O12/C anode material via polyvinyl alcohol as carbon source for high-rate rechargeable Li-ion batteries, Electrochim. Acta, 2013, 93, 173–178 CrossRef CAS.
- Y. Li, L. Wang and K. Zhang, et al., An encapsulation of phosphorus doped carbon over LiFePO4 prepared under vacuum condition for lithium-ion batteries, Vacuum, 2021, 184 Search PubMed.
- C. Lin, M. O. Lai and H. Zhou, et al., Mesoporous Li4Ti5O(12−x)/C submicrospheres with comprehensively improved electrochemical performances for high-power lithium-ion batteries, Phys. Chem. Chem. Phys., 2014, 16, 24874–24883 RSC.
- W. Chen, Z. Zhou and R. Wang, et al., High performance Na-doped lithium zinc titanate as anode material for Li-ion batteries, RSC Adv., 2015, 5, 49890–49898 RSC.
- H. Tang, Z. Tang and C. Du, et al., Ag-doped Li2ZnTi3O8 as a high rate anode material for rechargeable lithium-ion batteries, Electrochim. Acta, 2014, 120, 187–192 CrossRef CAS.
- H. Tang, J. Zhu and Z. Tang, et al., Al-doped Li2ZnTi3O8 as an effective anode material for lithium-ion batteries with good rate capabilities, J. Electroanal. Chem., 2014, 731, 60–66 CrossRef CAS.
- T. F. Yi, J. Z. Wu and J. Yuan, et al., Rapid Lithiation and Delithiation Property of V-Doped Li2ZnTi3O8 as Anode Material for Lithium-Ion Battery, ACS Sustainable Chem. Eng., 2015, 3, 3062–3069 CrossRef CAS.
- F. Qie and Z. Tang, Cu-doped Li2ZnTi3O8 anode material with improved electrochemical performance for lithium-ion batteries, Mater. Express, 2014, 4, 221–227 CrossRef CAS.
- Z. Zhang, R. Xun and L. Wang, et al., Construction of pseudocapacitive Li2-xLaxZnTi3O8 anode for fast and super-stable lithium storage, Ceram. Int., 2021, 47, 662–669 CrossRef CAS.
- Z. Liu, Z. Zhang and Y. Liu, et al., Facile and scalable fabrication of K+-doped Li1.2Ni0.2Co0.08Mn0.52O2 cathode with ultra high capacity and enhanced cycling stability for lithium ion batteries, Solid State Ionics, 2019, 332, 47–54 CrossRef CAS.
- Z. Tai, W. Zhu and M. Shi, et al., Improving electrochemical performances of Lithium-rich oxide by cooperatively doping Cr and coating Li3PO4 as cathode material for Lithium-ion batteries, J. Colloid Interface Sci., 2020, 576, 468–475 CrossRef CAS PubMed.
- H. Yang, X. H. Wang and Y. X. Qi, et al., Improving the Electrochemical Performance of Li2ZnTi3O8 by Surface KCl Modification, ACS Sustainable Chem. Eng., 2017, 5, 6099–6106 CrossRef CAS.
- J. L. Qin, H. L. Zhu and N. Lun, et al., Li2ZnTi3O8/C anode with high initial Coulombic efficiency, long cyclic life and outstanding rate properties enabled by fulvic acid, Carbon, 2020, 163, 297–307 CrossRef CAS.
- W. Chen, H. Liang and L. Shao, et al., Observation of the structural changes of sol-gel formed Li2MnTi3O8 during electrochemical reaction by in situ and ex situ studies, Electrochim. Acta, 2015, 152, 187–194 CrossRef CAS.
- S. Wang, Y. Bi and L. Wang, et al., Mo-doped Li2ZnTi3O8@graphene as a high performance anode material for lithium-ion batteries, Electrochim. Acta, 2019, 301, 319–324 CrossRef CAS.
- P. Zhang, X. Li and Y. Hua, et al., Enhanced performance and anchoring polysulfide mechanism of carbon aerogel/sulfur material with Cr doping and pore tuning for Li–S batteries, Electrochim. Acta, 2018, 282, 499–509 CrossRef CAS.
- Y. Nie, W. Xiao and C. Miao, et al., Boosting the electrochemical performance of LiNi0.8Co0.15Al0.05O2 cathode materials in situ modified with Li1.3Al0.3Ti1.7(PO4)3 fast ion conductor for lithium-ion batteries, Electrochim. Acta, 2020, 353 Search PubMed.
- Y. Chen, Y. Zhao and X. An, et al., Preparation and electrochemical performance studies on Cr-doped Li3V2(PO4)3 as cathode materials for lithium-ion batteries, Electrochim. Acta, 2009, 54, 5844–5850 CrossRef CAS.
- Y. Q. Tang, X. Liu and X. B. Huang, et al., Synthesis and electrochemical properties of Li2FeSiO4/C/Ag composite as a cathode material for Li-ion battery, J. Cent. South Univ., 2019, 26, 1443–1448 CrossRef CAS.
- W. Long, X. Wang and S. Yang, et al., Electrochemical properties of Li4Ti5−2xNixMnxO12 compounds synthesized by sol–gel process, Mater. Chem. Phys., 2011, 131, 431–435 CrossRef CAS.
- Z. Li, Y. Cui and J. Wu, et al., Synthesis and electrochemical properties of lithium zinc titanate as an anode material for lithium ion batteries via microwave method, RSC Adv., 2016, 6, 39209–39215 RSC.
- Z. Kou, C. Miao and P. Mei, et al., Enhancing the cycling stability of all-solid-state lithium-ion batteries assembled with Li1.3Al0.3Ti1.7(PO4)3 solid electrolytes prepared from precursor solutions with appropriate pH values, Ceram. Int., 2020, 46, 9629–9636 CrossRef CAS.
|
This journal is © The Royal Society of Chemistry 2022 |
Click here to see how this site uses Cookies. View our privacy policy here.