DOI:
10.1039/D1RA07336B
(Paper)
RSC Adv., 2022,
12, 3856-3861
Assessing the potential biological activities of TiO2 and Cu, Ni and Cr doped TiO2 nanoparticles
Received
2nd October 2021
, Accepted 21st January 2022
First published on 31st January 2022
Abstract
Nanoparticles are like magic bullets and nanomaterials exhibit appealing properties. Their size and morphology can be switched by dopants for certain biological activities. Nanoparticles in combination with certain drugs enhance the antibiotic effects and may be valuable in combating bacterial resistance. The antimicrobial potency of nanoparticles depends upon their ability to bind to the surface of microbial cell membranes resulting in modulation of basic cell functions such as respiration. We report herein the antibacterial, antifungal and antioxidant activities of pure TiO2 and TiO2 doped with 4% Cu, Ni and Cr. The performance of pure and doped nanoparticles has been compared with reference compounds. A comparison of the antifungal activities of the samples doped with TiO2 reveals that Cu–TiO2 exhibits improved performance against A. fumigatus but lower antifungal activity against Mucor sp. and F. solani. Cu–TiO2 and Ni–TiO2 showed good antibacterial action against B. bronchiseptica, while Cr–TiO2 nanoparticles displayed better activity against S. typhimurium as compared to pure TiO2. Moreover, pristine TiO2 and Ni–TiO2 nanoparticles were found to demonstrate maximum total antioxidant capacity.
1. Introduction
Nanotechnology is an emerging technology in the modern world providing a wide variety of applications in chemistry, biology, physics, materials and health sciences. A literature survey reveals that physico-chemical and biological properties of nanoparticles depend upon their size and shape.1–4 Nanoparticles exert their antimicrobial action by binding to the surface of microbial cell membranes and altering their basic cellular functions.5 Moreover, nanoparticles have been reported to penetrate the microbial cells and interact with nitrogen, sulfur and phosphorus of biomolecules.6 The size and morphology of nanoparticles have been found to exhibit noticeable potential for certain biological activities.7–10 In fact nanoparticles are employed in combination with certain drugs to enhance the antibiotic effects and to combat bacterial resistance.6
Semiconductor oxide nanoparticles are widely synthesized by chemical as well as green routes for application in biological sciences owing to their potential as antibacterial, antifungal and antioxidant materials.11–16 A recent article about the antibacterial activity of TiO2 doped ZnO composite material has reported that TiO2 doping in ZnO increased the activity index.17 Chitosan coated biologically synthesized TiO2 nanoparticles displayed good antibacterial activities against pandrug resistant (PDR) E. coli strain with the prominent zone of inhibition of 23 mm.18 In 2019, Islam et al., reported the biological activities of gold nanoparticles functionalized with Salix alba synthesized via a green route which displayed good antifungal activity and also showed appreciable antinociceptive and muscle relaxant properties.19 Ahamed et al., prepared copper oxide nanoparticles by precipitation method and found these materials to demonstrate excellent antimicrobial potential against various bacterial strains.20 Similarly Ag nanoparticles obtained through chemical and greener means have been found to demonstrate strong antimicrobial action.12,16,21–25 Metal nanoparticles such as those of palladium have been considered as ecofriendly materials owing to their antifungal potential.26
TiO2 nanoparticles are recognized as promising materials due to their biocompatibility, environment friendliness, stability and nontoxic nature. They exhibit strong antimicrobial and anticancer activities. It has been shown that the biological activities of TiO2 nanoparticles can be significantly improved with dopants and thus doping can be employed to tune their antimicrobial properties.27,28 In the present research, we have evaluated the antibacterial, antifungal and antioxidant potential of undoped TiO2 and doped titania with 4% Cu, Ni and Cr synthesized by a chemical route. We found that the doped TiO2 displayed appreciable antimicrobial properties compared to many previously reported studies on nanoparticles for biological activities. The excellent antimicrobial potential of doped nanoparticles suggests their potential for practical applications as alternative or combinational drugs.
2. Materials and methods
TiO2 and doped TiO2 nanoparticles were synthesized via a sol gel method using titanium(IV)-iso-propoxide Ti[OCH(CH3)2]4 precursor as described earlier.29 Copper, chromium and nickel acetate were employed as precursors for doping titania with 4% Cu, Ni and Cr. Analytical grade 1-propanol and deionized water were used as media in the synthesis procedure. Characterization of nanoparticles prepared by the same method has been reported in our previous work.30 The statistical analysis of antibacterial and antifungal activities was performed by using SPSS Pearson's product moment correlation coefficient method. All experiments for biological activities were performed in triplicate.
2.1. Biological activities protocols
The potential of the material against bacterial strains was evaluated according to literature reported procedure.31 Samples to be tested (5 μL of 4 mg per mL DMSO; 100 μg per disc) were pervaded on 6 mm discs of sterile filter paper put on seeded nutrient agar plate Cefixime-USP (20 μg per disc) and DMSO impregnated discs were employed as +ve and −ve controls, respectively.31,32 Clear zones of inhibition were determined after 24 hours of incubation time. Fungal strains were bought from fungal culture bank of Pakistan and antifungal activity was determined according to procedures described earlier.31 100 μL of harvested spores of each type were placed on plates containing 25 mL sterilized Sabouraud dextrose agar (SDA). 5 μL of each sample to be tested (4 mg per mL DMSO; 100 μg per disc) and standard drug (50 μg per disc) were pervaded in filter paper discs. Samples were incubated for 24–48 h at 28 °C. Afterwards, clear inhibition zones were measured employing a vernier caliper.
Total antioxidant activity of extracts was evaluated by method with a little modification as described elsewhere.33 A 4 mg per mL of each sample was dissolved in DMSO solvent. A 100 μL volume of each sample solution was then combined with 900 μL of solutions prepared using H2SO4 (0.6 M), NH4MoO4 (4 mM) and Na3PO4 (28 mM). Incubation of the reaction mixtures was done for about 90 min at 95 °C, followed by cooling to room temperature. Then, the absorbance was determined at the wavelength of 695 nm.32 A 100 μL of DMSO was used as a standard. Moreover, ascorbic acid was employed as positive standard for calibration curve with a concentration of 4 mg mL−1. The total antioxidant capacity (TAC) was indicated in μg ascorbic acid equivalent (μg AAE) per mg dry weight.
The reduction potential was investigated according to procedure described earlier.33 A 4 mg per mL of each sample was dissolved in DMSO solvent. A 100 μL volume of each sample solution was then combined with 200 μL of 0.2 M phosphate buffer and 250 μL of 1% w/v potassium ferricyanide. Incubation of the mixtures was done for 20 min at a temperature of 50 °C. Following this a 200 μL of 10% w/v trichloroacetic acid was added to the mixture for acidification. The resulting mixtures were centrifuged at the rate of 3000 rpm for about 10 min. The 150 μL of the supernatant layer was mixed with 50 μL of 0.1% w/v FeCl3 solution and optical density was measured at 630 nm using microplate reader.32 The results were expressed as μg AAE per mg dry weight using ascorbic acid as positive standard.
For estimating free radical scavenging potential, we used 2,2-diphenyl-1-picryl hydrazyl (DPPH). The mixture of 10 μL of extract (4 mg per mL) and 190 μL of DPPH (0.004% w/v) in methanol was incubated for 1 h in the dark. The optical density was similarly determined at the wavelength of 515 nm through microplate reader. Samples to be evaluated were first tested at a concentration of 200 μg per mL and those exhibiting good quenching activity (i.e. ≥50%) were tested at smaller concentration (200, 66.6, 22.2, 7.41 μg per mL) to find IC50 values. Following formula was employed to calculate the percent inhibition:
% Inhibition of the sample = % of scavenging activity = (1 − Abs/Abc) × 100. |
where, the absorbance of the DPPH solution along with sample is represented by Ab
s and absorbance of the negative standard (
i.e., reagent and solvent) is represented by Ab
c. IC
50 was evaluated by using Table curve software 2D version 4.
3. Results and discussion
3.1. Biological activities
The synthesized nanoparticles were examined for the evaluation of their antifungal, antibacterial and antioxidant potential. The antifungal activities of TiO2 and doped TiO2 analyzed against A. fumigatus, Mucor sp. and F. solani in comparison with the standard drug terbinafine can be seen in Fig. 1. Although the synthesized compounds demonstrate lower activity than the reference drug used against the fungal strains, but a comparison of the activities of the doped samples with pristine TiO2 reveals improved performance of Cu–TiO2 against A. fumigatus but a lower activity against Mucor sp. and F. solani as depicted from the zone of inhibition values of 18, 15 and 10 mm, respectively. A recent article indicate that TiO2 nanoparticles synthesized via green route exhibit no activity against A. fumigatus at concentrations of 10, 20 and 30 mg mL−1.34 In another report, biologically synthesized ZrO2 nanoparticles displayed a zone of inhibition of 34 ± 1 mm at a concentration of 15 mg mL−1.35 Similarly 15% Zr doped CeO2 nanoparticles employed against A. fumigatus have been reported to have a maximum zone of inhibition of 10 mm.36
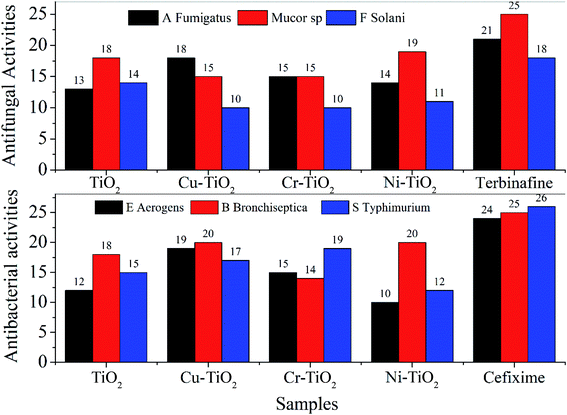 |
| Fig. 1 Comparative analysis of antifungal and antibacterial activities of TiO2 and doped TiO2 nanoparticles with reference drugs. | |
Ag nanoparticles are widely investigated for antimicrobial action. According to the recent literature Ag nanoparticles show no significant activity towards Mucor sp.37 Cu, Ni and Cr doped TiO2 indicated reduced activity against F. solani than that of the pure TiO2 nanoparticles. Moreover, literature survey reveals few reports using metal nanoparticles as antifungal agents against F. solani.38 One report manifested the reduction of the growth of F. solani to 1.42% (as compared to 93% of the control), at a concentration of 100 mg mL−1 for TiO2 nanoparticles prepared via a greener route.39 Direct comparison is difficult to make due to different experimental conditions and concentrations of the samples.
Antibacterial activities were also determined for TiO2 and doped TiO2 nanoparticles and compared with the reference drug cefixime. The result of antibacterial activity against E. aerogens, B. bronchiseptica and S. typhimurium has been displayed in Fig. 1. Although TiO2 and doped TiO2 displayed lower antibacterial action than that of the reference drug, but demonstrated enhanced performance compared to many reported studies employing nanoparticles as antibacterial agents. For instance, a study of the antibacterial action of chemically synthesized TiO2 encapsulated into zeolite and SiO2–TiO2 composite nanoparticles displayed no activity against E. aerogenes40 compared to our data of 12, 19, 15 and 10 mm for TiO2, Cu–TiO2, Cr–TiO2 and Ni–TiO2, respectively. Recent literature also showed that the Ag nanoparticles possess zone of inhibition of 7.1,41 24 (ref. 42) and 11 mm43 against E. aerogens at various concentrations of Ag nanoparticles. Similarly, MnO nanoparticles prepared via a green route displayed 9 mm zone of inhibition44 against B. bronchiseptica. Antibacterial action of nanoparticles against S. typhimurium has been reported for Ag,45 Se,46 Au47 and ZnO48 employing other methods and sample concentrations. Cu nanoparticles capped with 1% gum arabic49 displayed a higher zone of inhibition of 27 mm. Phosphorous and fluorine co-doped TiO2 are also reported against S. typhimurium (approximately 106 CFU mL−1) under day light.50 However, comparison of the results cannot be made with literature data due to different conditions of measurements and concentrations of the samples.
We further evaluated the antioxidant activities of the pure TiO2 as well as doped TiO2. Total antioxidant capacity (TAC) values were found to be highest for Ni–TiO2 followed by pristine TiO2 nanoparticles (see Fig. 2 & Table 1) whereas; minimum TAC values were obtained for Cu–TiO2 nanoparticles. Literature survey on the cytotoxic effects of TiO2 shows no significant cytotoxic effects on lung epithelial cells at a concentration of 0.1 mg mL−1 titania.51 Another article on the cytotoxicity studies of TiO2 and doped TiO2 on human cells demonstrates high oxidative stress mediated cytotoxicity on lung epithelial cells at a concentration of 2-200 μg mL−1 (ref. 52) which can be greatly reduced by the antioxidant N-acetyl cysteine.53 Mg and Cu co-doped TiO2 display high antibacterial potential against E. coli and S. aureus with exceptional cytocompatibility.54 The in vitro release kinetic study of co-doped TiO2 reveals that Cu ions at a concentration greater than 9 mg L−1 is cytotoxic to MC3T3-E1 cells, however maximum single day release is 2.29 mg L−1.54 A recent study has reported the cytotoxicity of Ni (1%) and Pt (0.2%) doped TiO2 in ARP-19 cells and dermal cells of balb/c mice where the ARP-9 cells have been found more susceptible to Pt doped TiO2 and less susceptible to Ni doped TiO2.55 Hence, to establish the toxicological effects of TiO2 and doped TiO2 nanoparticles further concentration based cytotoxicity studies are required.
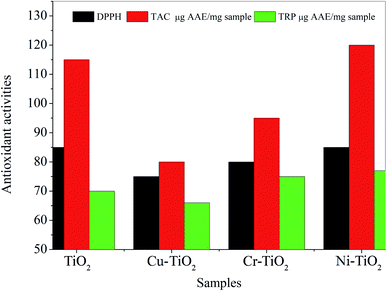 |
| Fig. 2 Comparative analysis of total antioxidant capacity (TAC) and total reducing power (TRP) of TiO2 and doped TiO2 nanoparticles using bar graph. | |
Table 1 Summary of the antifungal, antibacterial and antioxidant activities of TiO2 and doped TiO2 nanoparticles prepared by sol–gel method
Activity |
TiO2 |
4% Cu doped TiO2 |
4% Cr doped TiO2 |
4% Ni doped TiO2 |
Ref. drugs |
Antifungal |
|
Terbinafine |
A. fumigatus |
13 ± 1.91 |
18 ± 1.81 |
15 ± 1.23 |
14 ± 1.14 |
21 ± 1.46 |
Mucor sp. |
18 ± 1.49 |
15 ± 1.41 |
15 ± 1.34 |
19 ± 1.27 |
25 ± 0.26 |
F. solani |
14 ± 1.32 |
10 ± 0.19 |
10 ± 0.149 |
11 ± 0.707 |
18 ± 0.808 |
Antibacterial |
|
Cefixime |
E. aerogenes |
12 ± 1.51 |
19 ± 1.477 |
15 ± 1.473 |
10 ± 1.41 |
24 ± 1.11 |
B. bronchiseptica |
18 ± 1.81 |
20 ± 1.23 |
14 ± 1.14 |
20 ± 1.46 |
25 ± 1.44 |
S. typhimurium |
15 ± 1.41 |
17 ± 1.34 |
19 ± 1.27 |
12 ± 1.21 |
26 ± 1.12 |
Antioxidant |
|
DPPH |
85 |
75 |
80 |
85 |
— |
TAC μg AAE per mg sample |
115 ± 1.11 |
80 ± 0.21 |
95 ± 0.21 |
120 ± 0.21 |
— |
TRP μg AAE per mg sample |
70 ± 0.77 |
66 ± 0.75 |
75 ± 1.41 |
77 ± 0.88 |
— |
Total reducing power (TRP) was highest for Ni–TiO2 followed by Cr–TiO2 and TiO2 nanoparticles. The values of TAC and TRP coincided well with percent inhibition of DPPH as well (see Table 1).
3.1.1. Statistical analysis. Statistical analysis of antibacterial and antifungal activities was performed by using SPSS, where TiO2, Cu–TiO2, Cr–TiO2 and Ni–TiO2 were taken as variables to find out the correlation of antifungal/antibacterial activities between the reference drug and the nanoparticles.The correlation data between nanoparticles and the reference drug for antifungal activities as presented in the Table 2, is positive and moderate to strong (r = 0.807, r = 0.552, r = 0.822, r = 0.998). Data show the strongest correlation of reference drug with Ni–TiO2, Cr–TiO2 and TiO2 as seen from the correlation value of 0.998, 0.822 and 0.807, respectively. Although the antifungal activities against all the fungal strains are not much significant for Ni–TiO2, however the statistical analysis is representing the overall correlation of doped samples against all three strains with reference drug and is complementing our results of antifungal activities. In addition to the correlation of reference drug with doped samples, the correlation of TiO2 with doped samples can be seen from statistical data listed in Table 2. TiO2 has non-significant weak negative correlation with Cu (r = −0.047, p = 0.97) which indicates less correlation between TiO2 and Cu–TiO2 antifungal activities as manifested in our values. Similarly, moderate positive correlation with Cr–TiO2 (r = 0.327, p = 0.788), strong positive correlation of Ni–TiO2 (r = 0.842, p = 0.363) with pristine TiO2 is in accordance with our results. Correlations are all non-significant i.e., the p values are greater than 0.05 due to small sample size (n = 3). The large p values do not manifest invalid results rather it is a consequence of small data set.56
Table 2 Correlation coefficients of antifungal activities of nanoparticles obtained by statistical analysis
|
TiO2 |
Cu–TiO2 |
Cr–TiO2 |
Ni–TiO2 |
Terbinafine |
TiO2 |
1 |
|
|
|
|
Cu–TiO2 |
−0.047 (0.970) |
1 |
|
|
|
Cr–TiO2 |
0.327 (0.788) |
0.929 (0.242) |
1 |
|
|
Ni–TiO2 |
0.807 (0.402) |
0.5 (0.667) |
0.786 (0.425) |
1 |
|
Terbinafine |
0.807 (0.402) |
0.552 (0.628) |
0.822 (0.386) |
0.998 (0.039) |
1 |
Similarly for antibacterial activities TiO2 has non-significant positive correlation with Cu–TiO2 (r = 0.327, p = 0.788), weak negative correlation with Cr–TiO2 (r = −0.189, p = 0.879), strong positive correlation with Ni–TiO2 (r = 0.945, p = 0.212) (see Table 3). These complement the antibacterial activities given in Table 1. The negative values correspond to the pattern (increasing or decreasing) with the reference. Again, non-significant p values are due to small data set. Cefixime has non-significant positive correlation with TiO2 (r = 0.500, p = 0.667), good negative correlation with Cu–TiO2 (r = −655, p = 0.546), good positive correlation with Cr–TiO2 (r = 0.756, p = 0.454) and weak positive correlation with Ni–TiO2 (r = 0.189, p = 0.879).
Table 3 Correlation coefficients of antibacterial activities of nanoparticles obtained by statistical analysis
|
TiO2 |
Cu–TiO2 |
Cr–TiO2 |
Ni–TiO2 |
Cefixime |
TiO2 |
1 |
|
|
|
|
Cu–TiO2 |
0.327 (0.788) |
1 |
|
|
|
Cr–TiO2 |
−0.189 (0.879) |
−0.990 (0.091) |
1 |
|
|
Ni–TiO2 |
0.945 (0.212) |
0.619 (0.575) |
−0.500 (0.667) |
1 |
|
Cefixime |
0.500 (0.667) |
−0.655 (0.546) |
0.756 (0.454) |
0.189 (0.879) |
1 |
4. Mechanism of action of nanoparticles
The nanoparticles are known to have antimicrobial action (a) due to binding with the cell membrane and thus altering their basic cell functions such as respiration and (b) by penetration of the nanoparticles inside the cell and interaction with sulfur and phosphorous containing compounds, particularly DNA. The cell walls of microbes are composed of metal binding ligands which can reduce the metal ions by donating electrons and thus allowing metals to penetrate inside the cell.57 The accumulated metal ions can further penetrate into the cell components and damage the cell depending upon the cell's resistant for that metal. The severity of cell damage depends on various factors such as composition of cell wall, the sorption site, and the chemical interaction of the metal ions. Thus titanium ions can enter into the cell membrane and bind with DNA via direct bonding58 thus disrupting their normal function. This in turn prevents the cell replication and ultimately results in the death of the microorganisms.59 Doping of TiO2 resulted in the substitution of Ti4+ ions by the dopant metal (i.e. Cu2+, Cu+1, Ni2+, Ni3+ or Cr3+ in our case) depicted by XPS. We have seen that Cu, Cr and Ni doped TiO2 have shown better antifungal and antibacterial activities in general as compared to the undoped TiO2 and similarly reported data for other nanoparticles, indicating stronger interaction and sorption of Cu, Cr and Ni doped TiO2 into the microbial cells.
5. Conclusions
TiO2 nanoparticles were synthesized and doped with 4% Cu, Ni and Cr via sol–gel chemical method and evaluated for their antibacterial, antifungal and antioxidant potential. A comparison of the activities of the doped samples with pristine TiO2 reveals better performance of Cu–TiO2 against A. fumigatus but lower antifungal activity against Mucor sp. and F. solani. The antibacterial action of TiO2 and doped TiO2 was found lower than the reference drug but better than many reported studies employing nanoparticles as antibacterial agents. Statistical analysis provided an overall comparison of antibacterial/antifungal activities of doped samples with pristine TiO2 as well as reference drugs. Strong to moderate correlations were predicted from data of antimicrobial activities, however, correlations were non-significant (i.e., p > 0.05) due to small sample size. The total antioxidant capacity (TAC) values were found to be the highest for pristine TiO2 and Ni–TiO2 nanoparticles. Cu, Ni and Cr led to alteration of the antimicrobial action of TiO2 nanoparticles due to stronger chemical interaction and sorption of these metals into the microbial cells that led to cell death. The results of the biological assay demonstrated promising potential of doped TiO2 as alternative or combinational drug for practical applications.
Author contributions
Dr Shamsa Munir synthesized and characterized the nanoparticles, Dr Faiza Asghar analyzed the data. Dr Saira Tabassum carried out the biological activities of the samples. Dr Faryal Younis performed the statistical analysis of the results. Dr Afzal Shah and Dr Sher Bahadar Khan helped in writing of the manuscript.
Conflicts of interest
The authors declare no conflict of interest.
Acknowledgements
Prof. Dr Sher Bahadar Khan graciously acknowledges the Deanship of Scientific Research (DSR) at King Abdul Aziz University, Jeddah, Saudi Arabia for project under grant No. (KEP-32-130-42). Dr Shamsa Munir gratefully acknowledges the Higher Education Commission of Pakistan for project number 21-2499/SRGP/R&D/HEC/2019 and Quaid-i-Azam University Islamabad, Pakistan for providing laboratory facilities.
References
- V. Yadav, Adv. Electron. Elec. Eng., 2013, 3, 771–778 Search PubMed.
- N. Beyth, Y. Houri-Haddad, A. Domb, W. Khan and R. Hazan, Evidence-Based Complementary and Alternative Medicine, 2015, 2015 Search PubMed.
- N. Yudasari, P. A. Wiguna, W. Handayani, M. M. Suliyanti and C. Imawan, Appl. Phys. A: Mater. Sci. Process., 2021, 127, 1–11 CrossRef PubMed.
- A. S. Vijayanandan and R. M. Balakrishnan, Appl. Phys. A: Mater. Sci. Process., 2020, 126, 1–13 CrossRef.
- P. Mazur, I. Skiba-Kurek, P. Mrowiec, E. Karczewska and R. Drożdż, Int. J. Nanomed., 2020, 15, 3551 CrossRef CAS PubMed.
- E. Sánchez-López, D. Gomes, G. Esteruelas, L. Bonilla, A. L. Lopez-Machado, R. Galindo, A. Cano, M. Espina, M. Ettcheto and A. Camins, Nanomaterials, 2020, 10, 292 CrossRef PubMed.
- S. Pal, Y. K. Tak and J. M. Song, Appl. Environ. Microbiol., 2007, 73, 1712–1720 CrossRef CAS PubMed.
- A. H. Hammadi, S. A. Habeeb, L. F. Al-Jibouri and F. H. Hussien, Syst. Rev. Pharm., 2020, 11, 431–439 CAS.
- Y. Huo, Y. X. Han, P. Singh, J. P. Kang, J. Y. Pu, C. H. Piao and D. C. Yang, Appl. Phys. A: Mater. Sci. Process., 2021, 127, 1–10 CrossRef.
- C. Anuradha and P. Raji, Appl. Phys. A: Mater. Sci. Process., 2021, 127, 1–9 CrossRef.
- A. J. Haider, R. H. AL-Anbari, G. R. Kadhim and C. T. Salame, Energy Procedia, 2017, 119, 332–345 CrossRef CAS.
- A. Thirumurugan, S. Ramachandran, N. A. Tomy, G. Jiflin and G. Rajagomathi, Korean J. Chem. Eng., 2012, 29, 1761–1765 CrossRef CAS.
- M.-K. Yeo and M. Kang, Korean J. Chem. Eng., 2009, 26, 711–718 CrossRef CAS.
- M. Ahmadi, P. Amiri and N. Amiri, Korean J. Chem. Eng., 2015, 32, 1327–1332 CrossRef CAS.
- S. Rajeswaran, S. S. Thirugnanasambandan, S. R. Subramaniyan, S. Kandasamy and R. Vilwanathan, Appl. Phys. A: Mater. Sci. Process., 2019, 125, 105 CrossRef.
- G. Zhang, F. Zhang, H. Morikawa and Y. Chen, Appl. Phys. A: Mater. Sci. Process., 2014, 114, 1277–1283 CrossRef CAS.
- A. Menazea and N. S. Awwad, J. Mater. Res. Technol., 2020, 9, 9434–9441 CrossRef CAS.
- N. Zafar, B. Uzair, M. B. K. Niazi, S. Sajjad, G. Samin, M. J. Arshed and S. Rafiq, Adv. Polym. Technol., 2020, 2020, 8456024 CrossRef.
- N. U. Islam, K. Jalil, M. Shahid, A. Rauf, N. Muhammad, A. Khan, M. R. Shah and M. A. Khan, Arabian J. Chem., 2019, 12, 2914–2925 CrossRef.
- M. Ahamed, H. A. Alhadlaq, M. Khan, P. Karuppiah and N. A. Al-Dhabi, J. Nanomater., 2014, 2014, 637858 Search PubMed.
- A. A. Mostafa, S. R. Sayed, E. N. Solkamy, M. Khan, M. R. Shaik, A. Al-Warthan and S. F. Adil, J. Nanomater., 2015, 2015, 789178 Search PubMed.
- A. Roy, O. Bulut, S. Some, A. K. Mandal and M. D. Yilmaz, RSC Adv., 2019, 9, 2673–2702 RSC.
- B. K. Salunke, J. Shin, S. S. Sawant, B. Alkotaini, S. Lee and B. S. Kim, Korean J. Chem. Eng., 2014, 31, 2035–2040 CrossRef CAS.
- S. M. Shaban and D.-H. Kim, Korean J. Chem. Eng., 2020, 37, 1008–1019 CrossRef CAS.
- B. K. Salunke, E. Sathiyamoorthi, T. K. Tran and B. S. Kim, Korean J. Chem. Eng., 2017, 34, 943–951 CrossRef CAS.
- F. J. Osonga, S. Kalra, R. M. Miller, D. Isika and O. A. Sadik, RSC Adv., 2020, 10, 5894–5904 RSC.
- C. Gomez-Polo, S. Larumbe, A. Gil, D. Muñoz, L. R. Fernández, L. F. Barquín, A. García-Prieto, M. Fdez-Gubieda and A. Muela, Surf. Interfaces, 2021, 22, 100867 CrossRef CAS.
- K. Mukherjee, K. Acharya, A. Biswas and N. R. Jana, ACS Appl. Nano Mater., 2020, 3, 2016–2025 CrossRef CAS.
- S. Munir, S. M. Shah and H. Hussain, Mater. Des., 2016, 92, 64–72 CrossRef CAS.
- S. Munir, S. M. Shah and H. Hussain, Mater. Des., 2016, 92, 64–72 CrossRef CAS.
- A. Ihsan-Ul-Haq, I. Ahmed, I. Hussain, M. Jamil and B. Mirza, Pak. J. Bot., 2012, 44, 1487–1490 Search PubMed.
- H. Zafar, A. Ali, J. S. Ali, I. U. Haq and M. Zia, Front. Plant Sci., 2016, 7, 535 Search PubMed.
- S. Tabassum, M. Ahmed, B. Mirza, M. Naeem, M. Zia, Z. K. Shanwari and G. M. Khan, BMC Complementary Altern. Med., 2017, 17, 1–13 CrossRef PubMed.
- W. Ahmad, K. K. Jaiswal and S. Soni, Inorg. Nano-Met. Chem., 2020, 50, 1032–1038 CrossRef CAS.
- N. C. Joshi, N. Chaudhary and N. Rai, Bionanoscience, 2021, 11, 497–505 CrossRef.
- R. Bakkiyaraj, M. Balakrishnan, G. Bharath and N. Ponpandian, J. Alloys Compd., 2017, 724, 555–564 CrossRef CAS.
- T. Khan, A. Yasmin and H. E. Townley, Colloids Surf., B, 2020, 194, 111156 CrossRef CAS PubMed.
- A. Prasad, L. Williams, J. Garvasis, K. Shamsheera, S. M. Basheer, M. Kuruvilla and A. Joseph, J. Mol. Liq., 2021, 115805 CrossRef CAS.
- S. A. Monclou-Salcedo, S. N. Correa-Torres, M. I. Kopytko, C. Santoyo-Muñóz, D. M. Vesga-Guzmán, R. Castellares- Lozano, M. López-Amaris, A. D. Saavedra-Mancera and A. P. Herrera-Barros, Int. J. Agric. Nat. Resour., 2020, 47, 126–133 Search PubMed.
- L. R. Kalankesh, S. Rodríguez-Couto, M. A. Zazouli, Y. D. Shahamat, R. A. Dianati and M. Arghiani, J. Microbiol. Methods, 2019, 167, 105736 CrossRef PubMed.
- E. Bernardo-Mazariegos, B. Valdez-Salas, D. González-Mendoza, A. Abdelmoteleb, O. T. Camacho, C. C. Duran and F. Gutiérrez-Miceli, Rev. Argent. Microbiol., 2019, 51, 103–109 Search PubMed.
- A. V. A. Mariadoss, V. Ramachandran, V. Shalini, B. Agilan, J. H. Franklin, K. Sanjay, Y. G. Alaa, M. A.-A. Tawfiq and D. Ernest, Microb. Pathog., 2019, 135, 103609 CrossRef CAS PubMed.
- A. Ashraf, S. Zafar, K. Zahid, M. S. Shah, K. A. Al-Ghanim, F. Al-Misned and S. Mahboob, J. Infect. Public Health, 2019, 12, 275–281 CrossRef PubMed.
- S. A. Khan, S. Shahid, B. Shahid, U. Fatima and S. A. Abbasi, Biomolecules, 2020, 10, 785 CrossRef CAS PubMed.
- S. Sinsinwar, M. K. Sarkar, K. R. Suriya, P. Nithyanand and V. Vadivel, Microb. Pathog., 2018, 124, 30–37 CrossRef CAS PubMed.
- A. Rangrazi, H. Bagheri, K. Ghazvini, A. Boruziniat and M. Darroudi, Mater. Res. Express, 2020, 6, 1250h1253 Search PubMed.
- B. Lee and D. G. Lee, J. Appl. Microbiol., 2019, 127, 701–712 CrossRef CAS PubMed.
- R. C. d. Souza, L. U. Haberbeck, H. G. Riella, D. H. Ribeiro and B. A. Carciofi, Braz. J. Chem. Eng., 2019, 36, 885–893 CrossRef.
- P. Chawla, N. Kumar, A. Bains, S. B. Dhull, M. Kumar, R. Kaushik and S. Punia, Int. J. Biol. Macromol., 2020, 146, 232–242 CrossRef CAS PubMed.
- G. Schneider, B. Schweitzer, A. Steinbach, B. Z. Pertics, A. Cox and L. Kőrösi, Foods, 2021, 10, 1786 CrossRef CAS PubMed.
- S. Wadhwa, C. Rea, P. O'Hare, A. Mathur, S. Roy, P. Dunlop, J. Byrne, G. Burke, B. Meenan and J. McLaughlin, J. Hazard. Mater., 2011, 191, 56–61 CrossRef CAS PubMed.
- K. Kaviyarasu, N. Geetha, K. Kanimozhi, C. M. Magdalane, S. Sivaranjani, A. Ayeshamariam, J. Kennedy and M. Maaza, Mater. Sci. Eng., C, 2017, 74, 325–333 CrossRef CAS PubMed.
- J. Ahmad, M. Siddiqui, M. Akhtar, H. Alhadlaq, A. Alshamsan, S. Khan, R. Wahab, A. Al-Khedhairy, A. Al-Salim and J. Musarrat, Hum. Exp. Toxicol., 2018, 37, 496–507 CrossRef CAS PubMed.
- B. Wang, Z. Wu, S. Wang, S. Wang, Q. Niu, Y. Wu, F. Jia, A. Bian, L. Xie and H. Qiao, Mater. Sci. Eng., C, 2021, 128, 112322 CrossRef CAS PubMed.
- S.-E. Jin, E.-J. Kim, H. Kim, H. Kim, W. Hwang and S. W. Hong, Mater. Sci. Eng., C, 2020, 115, 110843 CrossRef CAS PubMed.
- S. Greenland, S. J. Senn, K. J. Rothman, J. B. Carlin, C. Poole, S. N. Goodman and D. G. Altman, Eur. J. Epidemiol., 2016, 31, 337–350 CrossRef PubMed.
- B. Igiri, S. Okoduwa, G. O. Idoko, E. P. Akabuogu, A. O. Adeyi and I. K. Ejiogu, J. Toxicol., 2018, 2018, 2568038 Search PubMed.
- N. Asefifeyzabadi, P. K. Das, A. H. Onorimuo, G. Durocher and M. H. Shamsi, RSC Adv., 2021, 11, 28332–28341 RSC.
- I. X. Yin, J. Zhang, I. S. Zhao, M. L. Mei, Q. Li and C. H. Chu, Int. J. Nanomed., 2020, 15, 2555 CrossRef CAS PubMed.
|
This journal is © The Royal Society of Chemistry 2022 |
Click here to see how this site uses Cookies. View our privacy policy here.