DOI:
10.1039/D1RA08116K
(Paper)
RSC Adv., 2022,
12, 777-784
Fluorescent probe for the detection of hypochlorous acid in water samples and cell models†
Received
5th November 2021
, Accepted 15th December 2021
First published on 24th December 2021
Abstract
Hypochlorous acid (HClO) is a special kind of reactive oxygen species, which plays an important role in resisting pathogen invasion and maintaining cell redox balance and other physiological processes. In addition, HClO is commonly used in daily life as a bleaching and disinfectant agent. Its excessive use can also lead to death of water animals and serious respiratory and skin diseases in humans. Therefore, it is of great significance to develop a quick and convenient tool for detecting HClO in the environment and organisms. In this paper, we utilize the specific reaction of HClO with dimethylthiocarbamate to develop a novel naphthalene derivative fluorescent probe (BNA-HClO), it was designed and synthesized by using 6-(2-benzothiazolyl)-2-naphthol as the fluorophore and N,N-dimethylthiocarbamate as the recognition group. BNA-HClO shows large fluorescence enhancement (374-fold), high sensitivity (a detection limit of 37.56 nM), rapid response (<30 s), strong anti-interference ability and good specificity in vitro. Based on the outstanding in vitro sensing capability of BNA-HClO, it has been successfully used to detect spiked HClO in tap water, medical wastewater and fetal bovine serum with good recovery. BNA-HClO has also been successfully used as a portable test strip for the in situ semi-quantitative detection of HClO in tap water solutions. In addition, BNA-HClO can successfully enable the detection and imaging of exogenous and endogenous HClO in living cells. This work provides a simple and effective tool for the detection and imaging of HClO in environmental and biological systems, and provides some theoretical guidance for future exploration of biological and pathological studies related to HClO.
1 Introduction
Hypochlorous acid (HClO) is a special reactive oxygen species (ROS), which is produced by myeloperoxidase (MPO)-mediated peroxidation of chloride ions and is playing an important role in killing pathogens.1 HClO plays an important role in the human immune system. The normal concentration of hypochlorous acid in the human body is generally within the range of 200 μM.2 When the HClO concentration in the human body dynamically fluctuates within the normal value, it can be directly used as a part of the immune defense process, which will destroy invading bacteria and pathogens. Meanwhile, the high oxidation ability of HClO makes it a double-edged sword. For example, an abnormal level HClO in organisms will cause a series of diseases, including cardiovascular disease and rheumatoid arthritis, as well as atherosclerosis and cancer.3,4 Meanwhile, HClO that is produced by the chlorination of water (Cl2 + H2O → HClO) is also widely used as an antimicrobial agent and disinfectant for water treatment. Unfortunately, excess HClO can result in serious harm to the environment and human health. It was reported that the use of chlorine-containing disinfectants for disinfection can increase the mortality of zebrafish embryos and increase the risk of bladder cancer in the human body.5–7 In addition, according to the World Health Organization (WHO), the allowable upper limit of HClO in tap water is 8.6 μmol L−1. Therefore, considering the potential hazard of HClO towards environment and humans, it is of great significance to develop a fast and effective method to quantify HClO in the environmental and biology.
The strategies for detecting HClO including colorimetric,8 electrochemical,9 chemiluminescence,10 fluorescent probe.11–27 Among these methods, fluorescence probe has attracted much attention owing to their various advantages over other technologies, such as simple operation, good selectivity, high sensitivity, fast response, low-cost, noninvasive detection and high spatial and temporal resolution.28–36 In the past few years, a number of HClO fluorescent probes have been reported (see Table S1†), but only a few of them can be directly used for the real-time detection of HClO in real water samples. The main reasons are attributed to the following two points. For example, turbidity interferes more with the detection of HClO in domestic water, and while competitive interference from other active substances is greater in the biological microenvironment. On the other hand, HClO itself has high reactivity and short life. The above difficulties have greatly increased the research on the development of probes for the detection of HClO in environmental and biological systems.
Interestingly, HClO can oxidize sulfide-type amino acids, and this characteristic of HClO is different from other ROS.37 Inspired by these characteristic, we develop a new type of fluorescent probe (BNA-HClO) that can be directly used for the quantitative detection of HClO in water samples and made into portable test paper to be used for highly selective monitoring HClO. The BNA-HClO could be easily synthesized through a one step reaction between 6-(2-benzothiazoly)-2-naphthalenol (the fluorophore) and dimethylcarbamothioic chloride (the HClO responsive site and the fluorescence quencher). The BNA-HClO has demonstrated high selectivity, excellent specificity, rapid response and significant fluorescence color change by investigating the analysis and detection performance of the probe in an aqueous solution. Notably, the BNA-HClO has been successfully applied to the detection of HClO in real water samples from remote areas (Ningyuan County Environmental Monitoring Station, Yongzhou, Hunan Province) as well as in biological fetal calf serum. The BNA-HClO has also been successfully prepared into a convenient test paper for highly selective quantitative monitoring of HClO in tap water. Even more importantly, the probe BNA-HClO allows direct in situ tracking of exogenous and endogenous HClO in HeLa cells.
2 Materials and methods
2.1 Synthesis and characterization
Unless otherwise stated, all reagents and solvents were of analytical grade, which were obtained directly from commercial suppliers without further purification. 6-Hydroxy-2-naphthaldehyde, 2-aminothiophenol, p-toluenesulfonic acid monohydrate, and dimethylcarbamothioic chloride were all purchased directly from Sigma. The secondary distilled water was used throughout all over the experiment. The 1H NMR and 13C NMR spectra were measured by Bruker 400 M nuclear magnetic resonance spectrometer using d6-DMSO as the solvent, in which chemical shifts are in ppm and tetramethylsilane (TMS) is used as an internal standard. The mass spectra of different systems were measured on Bruker solanX 70 FT-MS. All fluorescence measurements were measured in the G9800A fluorescence spectrophotometer (Agilent, USA) at the slits of 5.0/5.0 nm. The pH was measured using a METTLER TOLEDO FE-28 pH; Fluorescence imaging of HeLa cells was obtained by Nikon confocal microscopy (Ti-E+A1 SI).
2.2 Synthesis and characterization
2.2.1. Synthesis of compound 1. 6-(2-Benzothiazoly)-2-naphthalenol was synthesized according to the ref. 38, which was named compound 1 in this paper. 1H NMR (500 MHz, d6-DMSO) δ (ppm): 8.55 (s, 1H), 8.15 (d, J = 7.9 Hz, 1H), 8.12–8.04 (m, 2H), 8.01 (d, J = 8.8 Hz, 1H), 7.85 (d, J = 8.6 Hz, 1H), 7.48 (d, J = 7.6 Hz, 1H), 7.21 (s, 1H), 7.13 (d, J = 8.0 Hz, 1H), 4.67 (s, 1H). 13C NMR (126 MHz, d6-DMSO) δ (ppm): 168.21, 157.65, 154.17, 138.26, 136.65, 134.83, 131.21, 128.58, 127.84, 127.59, 127.08, 125.98, 124.66, 123.08, 122.76, 120.28, 109.41. MS(EI) m/z, C17H11NOS calcd 277.0, found [C17H11NOS]+ 277.0 (Fig. S1–S3†).
2.2.2. Synthesis of probe BNA-HClO. As show in Fig. 1, N,N-diisopropylethylamine (520 mg, 4 mmol) was added into compound 1 (277 mg, 1 mmol) dissolved in 20 mL dry dichloromethane and then the mixture was stirred in ice bath for 30 min to cooled to 0 °C for under N2 atmosphere. Dimethylcarbamothioic chloride (309 mg, 2 mmol) was added to 5 mL dry dichloromethane. Then, dimethylcarbamothioic chloride in dry dichloromethane was added into the above admixture drop by drop. After stirring for 6 h in at 0 °C for under N2 atmosphere. After the completion of the reaction (monitored by TLC), the mixture was washed in turn by 50 mL water and saturated brine and dried over anhydrous Na2SO4. Finally, the solvent was removed by rotary evaporation, and the residue was subsequently purified with column chromatography by petroleum ether/ethyl acetate = 15
:
1 (v/v) to afford 165 mg BNA-HClO as white solid with 50.0% yields. 1H NMR (400 MHz, d6-DMSO), δ (ppm): 8.73 (s, 1H), 8.25 (d, J = 8.5 Hz, 1H), 8.19 (d, J = 8.2 Hz, 2H), 8.11 (d, J = 6.3 Hz, 1H), 7.72 (s, 2H), 7.58 (t, J = 7.6 Hz, 1H), 7.50 (t, J = 7.5 Hz, 1H), 7.39 (d, J = 8.8 Hz, 1H), 3.33 (s, 6H). 13C NMR (101 MHz, d6-DMSO) δ (ppm): 186.69, 167.66, 154.10, 153.27, 135.11, 135.05, 132.01, 130.33, 129.16, 127.65, 127.21, 126.10, 124.99, 124.54, 123.35, 122.87, 119.97, 47.68. MS(EI) m/z, C20H16N2OS2 calcd 364.0 found [C20H16N2OS2 + H]+ 365.0(Fig. S4–S6†).
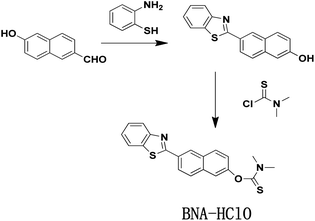 |
| Fig. 1 The synthesis of fluorescent probe BNA-HClO. | |
2.3 Spectroscopic analysis
The BNA-HClO was dissolved in DMF to form a (1.0 mM) stock solution and store at 4 °C. According to the reported method. NaClO stock solution was prepared for immediate use, which was used as the source of HClO. Other species, such as KCl, NaCl, CaCl2, FeCl3, Na2SO3, MgCl2, NaNO3, Hcy, GSH, H2O2, 1O2, and HNO were all dissolved in double distilled water and configured to the required concentration for selective and competitive experiments. Unless specifically stated. A stock solution of the probe BNA-HClO was diluted to 10 μM with 10.0 mM phosphate buffer saline (PBS/C2H5OH = 1
:
1, v/v, pH = 7.4), and the various concentrations of HClO (0–200 μM) was added, the resulting mixture was equilibrated for 1 min at room temperature and then the fluorescence intensity was measured. The excitation wave-length was set at 384 nm, the emission spectra were scanned from 450 nm to 600 nm, and both excitation and emission slit widths were set at 5 nm. The fluorescence spectra were collected at a wavelength of 510 nm, the data of these experiments were reported as the mean ± standard deviation of triplicate experiments. Other optical experiments were similar.
3 Results
3.1 Desigh strategy of probe
In this work, an ICT-based fluorescent probe named BNA-HClO was rationally designed and synthesized for the special response to HClO. The generation of “push–pull” fluorescent structure induced by the specific reaction of HClO was adopted to enhance the sensitivity. The naphthalene hydroxyl derivative was selected as a fluorophore by virtue of its high fluorescence quantum yield, effective two-photon active absorption cross-section, high photostability, concise synthesis, and easy modification which may offer a perfect platform for constructing two-photon fluorescence probe. Furthermore, it has been reported that sulfide-type amino acids can be oxidized through the initiation of an electrophilic addition of Cl+ which from the decomposition of HClO.39–41 Therefore, we selected dimethylcarbamothioic chloride with a strong intromolecular charge transfer effect (ICT) as the HClO reactive group and the fluorescence quencher. As a consequence, based on the oxidation of HClO, N,N-dimethylthiocarbamate was removed, releasing the D–π–A structure of naphthalene derivatives as well as the fluorescence recovering (Fig. 2). Therefore, probe BNA-HClO can recognize HClO selectively.
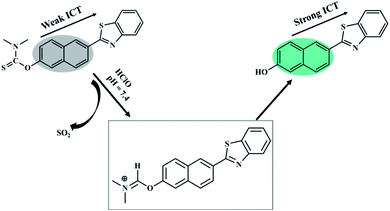 |
| Fig. 2 Possible recognition mechanism of fluorescent probe for HClO. | |
3.2 Fluorescence response of probe BNA-HClO toward HClO
In order to inquire the ability of BNA-HClO to qualitatively and quantitatively detect HClO, we first tested the UV-vis spectra response BNA-HClO toward HClO. As shown in Fig. S7,† BNA-HClO exhibited an maximum absorption peak at a wavelength (λabs) of 340 nm (ε = 1972 M−1 cm−1), upon addition of HClO to probe BNA-HClO solution, the maximum absorption spectrum of probe BNA-HClO was slightly red-shifted to 384 nm (ε = 2825 M−1 cm−1). However, the change between the maximum absorption wavelength of probe BNA-HClO and HClO before and after response was not obvious. Therefore, the following studies were focus on the fluorescence method. The probe BNA-HClO was investigated for fluorescence detection of HClO in PBS/C2H5OH (1
:
1, v/v, pH = 7.4), due to the inhibiting of intramolecular charge transfer (ICT) process between fluorophore and quencher. The probe BNA-HClO was essentially non-fluorescent in the absence of HClO (ф = 0.10). Nevertheless, the fluorescence intensity of probe BNA-HClO significantly increased at 510 nm (ф = 0.57) as the HClO concentration increased from 0 to 200 μM (Fig. 3a). When the HClO concentration reached 200 μM, the fluorescence intensity of probe BNA-HClO reached a plateau, which was 374 folds the blank value. As shown in Fig. 3b, the fluorescence emission of probe BNA-HClO has a good linear relationship with the HClO for concentrations of HClO ranging from 0 to 20 μM. The regression equation was F/F0 = 7.5351 [HClO] + 0.4978, with R2 = 0.9909. Moreover, the detection limit of probe BNA-HClO for HClO was estimated to be as low as 37.54 nM according to LOD = 3δ/k, where σ is means of the standard deviation of the blank measures, and k is a numerical slope factor between the fluorescent intensity and the concentrations of HClO. These results showed that probe BNA-HClO could be used to measure HClO quantitatively in a wide linear range.
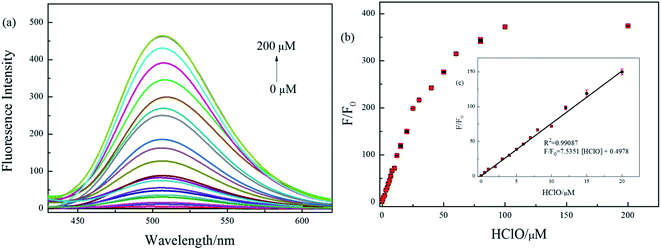 |
| Fig. 3 (a) Fluorescence emission spectra of the probe (10 μM) in the presence of different concentrations of HClO (0–200 μM). (b) The calibration curve of the sensing system, inset exhibits the linear response at low concentration of HClO (0–20 μM). F and F0 represent the fluorescence intensity of the presence or absence of HClO. PBS buffer solution system (10 mM, pH 7.4, C2H5OH/PBS = 1 : 1, v/v), λex = 384 nm, λem = 450–600 nm. | |
3.3 Time – dependence in the detection process of HClO
HClO has the characteristics of high reactivity and short life. Whether BNA-HClO responds quickly to HClO is the key point to determine whether the probe can accurately detect HClO. Therefore, we also tested the reaction time of probe BNA-HClO and HClO under physiological conditions. As shown in Fig. 4, with HClO (100 μM) added to BNA-HClO (10 μM), the probe immediately shows strong fluorescence emission at 510 nm within 30 s, which shows that probe BNA-HClO responded to HClO fast and sensitively. The fluorescence intensity of probe BNA-HClO with HClO remained stable for the next 240 s, indicating that the fluorescence stability of probe BNA-HClO was perfect. In contrast, the probe BNA-HClO without HClO did not show any obvious fluorescence change within 240 s.
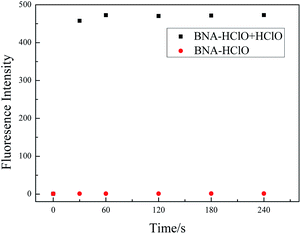 |
| Fig. 4 The time-dependent fluorescence spectra of probe BNA-HClO responses to HClO. Data were acquired in 10.0 mM PBS/C2H5OH (1 : 1, v/v, pH 7.4). Fluorescence signals with λex = 384 nm, λem = 510 nm. | |
3.4 Effect of pH
We also investigated the effect of pH to probe BNA-HClO. As shown in Fig. 5, the probe itself had no influence on the fluorescence by the pH of mediums within the range from 5.0 to 9.0. In the presence of HClO (100 μM), the fluorescence intensity of probe BNA-HClO gradually increased during the pH raising (5.0–7.4). However, the fluorescence intensity obviously decreased in the pH = 8.0–9.0 for which the reaction was inhibited, but the signal-to-noise ratio is relatively obvious. The main reason may be that the reaction between the added HClO specificity and probe BNA-HClO leads to the restoration of the push–pull electronic structure of probe BNA-HClO, so the fluorescence intensity of probe BNA-HClO increases significantly. However, the alkaline reaction system will inhibit the reaction and weaken the fluorescence intensity of the system.36 However, under physiological conditions (pH = 7.4), the fluorescence signal to back ratio enhancement of the probe BNA-HClO is significantly higher up to 374-fold. Thus, the probe BNA-HClO has the potential to detect HClO in a biological environment.
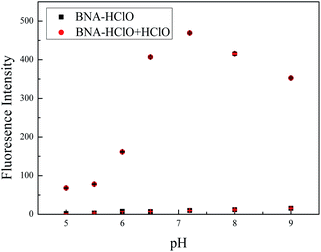 |
| Fig. 5 The effect of different pH on the fluorescence intensity of the probe BNA-HClO (10.0 μM) toward HClO (100.0 μM); data were acquired in 10.0 mM PBS/C2H5OH (1 : 1, v/v, pH 7.4). Fluorescence signals with λex = 384 nm, λem = 510 nm. | |
3.5 Selectivity and competition experiments
For an excellent probe, a high selectivity is required. To do this, various analytes including HClO (100 μM) and other potential interfering substances such as (1 mM) KCl, NaCl, CaCl2, FeCl3, Na2SO3, MgCl2, NaNO3, Hcy, GSH, H2O2, 1O2, HNO were added to the probe BNA-HClO solution. As shown in Fig. 6, only addition of HClO induced the fluorescence intensity of probe BNA-HClO solution increased significantly at 510 nm while other analytes induced negligible changes, which indicates that probe BNA-HClO has high selectivity for HClO over other potential interfering substances. To further assess its utility as a HClO selective fluorescent probe, its fluorescence spectrum response to HClO in the presence of other species mentioned above was also tested. As shown in Fig. 7, the fluorescence response of probe BNA-HClO to HClO in the presence of other potential interfering substances (1 mM KCl, NaCl, CaCl2, FeCl3, Na2SO3, MgCl2, NaNO3, Hcy, GSH, H2O2, 1O2, HNO) was studied, and no obvious interference was detected. All these results indicate that probe BNA-HClO is selective for HClO and promising for detection of HClO in complex environmental conditions.
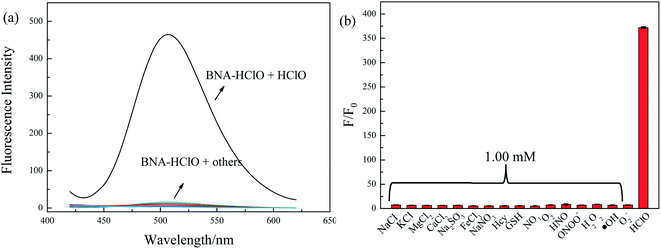 |
| Fig. 6 Selective investigation of probe BNA-HClO. (a) Fluorescence emission spectra of BNA-HClO in response to different substances, (b) F/F0 of BNA-HClO in response to different substances. | |
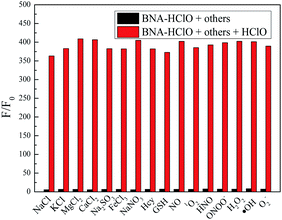 |
| Fig. 7 Investigation of anti-jamming ability of probe BNA-HClO. | |
3.6 Proposed detection mechanism of probe
In order to assess the sensing mechanism of BNA-HClO toward HClO, the reaction system was verified by HPLC and MS spectra methods. The HPLC analysis showed that the reaction mixture produced a fluorescence compound, which has the same retention time to compound 1. Comparing the three pictures in Fig. S8a,† it could be seen that after probe BNA-HClO and HClO reacted for 1 min, the signal of compound 1 was significantly increased, indicating that the reaction of probe BNA-HClO and HClO did produce compound 1. At the same time, the product was characterized by ESI-MS spectrometry. As shown in Fig. S8b,† a new peak appeared at 278.95 (m/z) in the spectrum, suggesting that the transformation from probe BNA-HClO to compound 1 ([M + H]+m/z calcd 278.06) was induced by HClO. The response mechanism of probe BNA-HClO verified by the two experimental results at the same time indicated that the enhanced fluorescence of probe BNA-HClO was indeed due to sulfide-type amino acids can be oxidized through the initiation of an electrophilic addition of Cl+ which from the decomposition of HClO, restoring the D–π–A structure of the naphthalene derivative and the fluorescence.
3.7 Real sample analysis
Hypochlorous acid is an important bioactive oxygen species that plays a vital role in the human immune system; it is also widely used in the natural environment as a disinfectant and bleaching agent for tap water and medical wastewater. For example, in disinfection of tap water, medical wastewater, and daily life. HClO is used at a concentration in the range of 10−5 to 10−2 M.42 Therefore, whether the probe BNA-HClO can be directly used for the detection of HClO in actual domestic water samples and biological systems is a key point to evaluate the performance of this probe. In this experiment, tap water, medical wastewater and serum were selected as the reaction media for the study, and the practical application of the probe BNA-HClO was investigated separately. Both tap water and medical wastewater are provided by the Yongzhou Environmental Testing Station, which were filtered through filter paper (Φ = 7 cm) to remove impurities during the measurement, and the medical sewage is diluted 10 times with double distilled water before the measurement. Therefore, according to the linear regression equation (Fig. 3b), the results of the determination of HClO in water samples and the results of spiked recovery experiments was shown in Table 1: the HClO levels in fetal bovine serum, tap water, and medical wastewater were non-detect, 2.98 μM, and 93.0 μM, respectively. The test results were within the standard ranges for tap water discharge (8.6 μM)43 and medical wastewater discharge (123–190 μM).44 The recoveries of all spiked standards were in the range of 96.1–102.7%, which proved the validity and accuracy of the method for the detection of HClO. The above results indicate that the probe BNA-HClO has the potential to be used for the detection of HClO in environmental water samples and biological systems.
Table 1 Detection of HClO in actual water samples by BNA-HClO probe
Sample |
Determineda (μM) |
Added HClO (μM) |
Founda (μM) |
Recovery (%) |
Mean ± standard deviation (n = 3). |
1 |
ND |
2.00 |
2.05 ± 0.01 |
102.4 |
5.00 |
4.97 ± 0.06 |
99.4 |
10.00 |
9.61 ± 0.06 |
96.1 |
2 |
2.98 ± 0.05 |
2.00 |
5.02 ± 0.03 |
102.0 |
5.00 |
8.09 ± 0.04 |
102.3 |
10.00 |
12.90 ± 0.09 |
98.0 |
3 |
9.30 ± 0.07 |
2.00 |
11.36 ± 0.02 |
102.6 |
5.00 |
14.44 ± 0.04 |
102.7 |
10.00 |
19.90 ± 0.08 |
96.6 |
3.8 Paper test strips
The above experimental results all prove the outstanding sensitivity and excellent selectivity of BNA-HClO for the detection of HClO. Therefore, we have prepared a portable test paper that can be directly used for the determination of HClO in the environment, and explored the specificity and qualitative and quantitative analysis performance of the test paper for HClO. The filter paper was first cut into circular pieces of paper with a diameter of 15 cm, and then inserted into the probe solution with a concentration of 10 μM for 30 minutes, and finally dried naturally. Firstly, when the aqueous solutions containing 100 μM HClO and 1 mM KCl, NaCl, CaCl2, FeCl3, Na2SO3, MgCl2, NaNO3, Hcy, GSH were dropwise added onto the test strips at room temperature respectively, and the fluorescence change of the test paper under UV lamp (365 nm) by naked eyes was recorded. As shown in Fig. 8a, only when HClO is added to the test paper, the test paper exhibits strong green fluorescence under UV lamp (365 nm). Secondly, the test paper is used to detect different concentrations of HClO. As shown in Fig. 8b, the fluorescent color of the test paper changes from blue to green under UV lamp (365 nm), and the fluorescence is continuously enhanced. In addition, the dried test paper is directly immersed in pretreated tap water. The color of the test paper is compared with the standard color card (Fig. 8b), which met the standards of the required concentrations in tap water. These results indicated that the test paper is not only convenient to carry, sensitive to identification, capable of semi-quantitative in situ identification of HClO, and has important significance for monitoring the HClO in tap water or other environmental water samples.
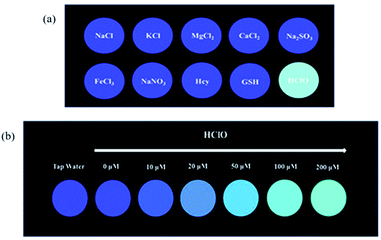 |
| Fig. 8 (a) Fluorescence images of test paper soaked with probe BNA-HClO to detection interfering substances (1 mmol L−1) and HClO (100 μmol L−1) under 365 nm, (b) fluorescence images of test paper soaked with probe BNA-HClO to detection HClO (0–200 μmol L−1) in water under 365 nm. | |
3.9 Cytotoxicity studies
To ensure the safety of probe BNA-HClO for bioimaging studies, the toxicity of probe BNA-HClO and compound 1 on HeLa cells were first assessed by the CCK-8 method. As seen in Fig. 9, when (0–12 μM) probe BNA-HClO was co-incubated with cells for 48 h, HeLa cell viability decreased from 102.50% to 94.24%. The survival rate was higher than 90%, meanwhile, when (0–12 μM) compound 1 was co-incubated with HeLa cells for 48 h, the HeLa cell viability decreased from 103.60–92.35%, and the cell survival rate was also higher than 90%. And as seen in Fig. S9,† the higher concentrations of the probe BNA-HClO (15–30 μM) also have good cellular compatibility. The above experimental results shown that the probe BNA-HClO and compound 1 did not exhibit significant cytotoxicity to HeLa cells within the experimentally investigated concentration range, indicating that the probe BNA-HClO has good cytocompatibility and can be directly used for the detection and imaging studies of HClO in biological systems.
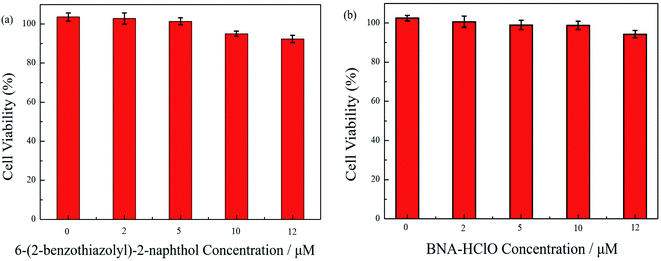 |
| Fig. 9 Cytotoxicity of compound 1 (a) and probe BNA-HClO (b). | |
3.10 Cell imaging
The results of in vitro experiments and cytotoxicity assays demonstrated the good sensitivity, specificity, and cytocompatibility of the probe BNA-HClO in detecting HClO. Therefore, the imaging of exogenous and endogenous HClO in HeLa cells with the probe BNA-HClO was investigated by fluorescence confocal microscopy. In the detection of exogenous HClO in live cells, it can be seen from Fig. 10a–c that only the probe BNA-HClO (10 μM) and HeLa cells were incubated for 30 min, and the probe BNA-HClO had no obvious fluorescence in the cells under the excitation light of 405 nm. However, when HeLa cells were incubated with the probe BNA-HClO (10 μM) and continued with the addition of exogenous HCIO (40 μM) for 30 min, the green fluorescence of HeLa cells increased significantly (Fig. 10d–f). The above experimental results indicate that the probe BNA-HClO has good cytocompatibility and membrane permeability and has the potential to detect exogenous HClO in living cells. In order to further explore the potential of probe BNA-HClO to detect HClO in biological environment, this experiment further applied probe BNA-HClO to detect endogenous HClO in living cells. HeLa cells were first pretreated with LPS(μg mL−1) and PMA (μg mL−1) stimulation to produce endogenous HClO, a clear green fluorescence enhancement was observed in the HeLa cells after continued incubation with the probe BNA-HClO (10 μM) (Fig. 10g–i). Meanwhile, to confirm that the fluorescence signal was mainly caused by endogenous HClO produced by LPS and PMA stimulation, this experiment used ABAH as an inhibitor of MPO enzyme activity to reduce the production of endogenous HClO by cells. As shown in Fig. 10j–l, HeLa cells were first incubated with ABAH and then incubated with LPS, PMA and the probe BNA-HClO in sequence, the results were compared with Fig. 10g–i, the cell fluorescence intensity was significantly weakened, which proved that the change of the probe fluorescence signal was caused by the intracellular production of endogenous HClO. The above experimental results indicate that the probe BNA-HClO can also be used for imaging and analysis of endogenous HClO in living cells.
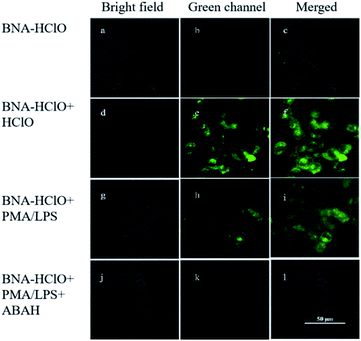 |
| Fig. 10 Confocal fluorescence imaging of the probe BNA-HClO with exogenous and endogenous HClO in HeLa cells. (a–c) The imaging of HeLa cells treated with BNA-HClO (10 μM) for 30 min; (d–f) The imaging of HeLa cells treated with 10.0 μM BNA-HClO for 30 min and then treated with 40 μM HClO for 30 min; (g–i) The imaging of HeLa cells were incubated with LPS (1.0 μg mL−1) for 12 h, and then were incubated with PMA (1.0 μg mL−1) for 1 h, and finally probe BNA-HClO (10 μM) was added incubate for 30 min; (j–l) HeLa cells were first incubated with ABAH (200 μM) for 3 h, then with LPS (1.0 μg mL−1) for 12 h, and incubate with PMA (1.0 μg mL−1) for 1 h, and finally incubate with BNA-HClO (10 μM) for 30 min. The fluorescence intensities were collected at the green channel (490–550) upon excitation at 800 nm. Scale bar: 50 μm. | |
4 Conclusion
In this paper, the probe BNA-HClO was successfully synthesized based on the ICT mechanism using N,N–dimethylthiocarbamate bond as the recognition group and fluorescence quenching group. The probe BNA-HClO has the advantages of rapid response, high sensitivity, good selectivity, strong anti-interference ability, the detection limit of the probe BNA-HClO is 37.5 nM, which is much lower than the threshold limit set by the National Standardization Administration. What's more, the probe BNA-HClO can be directly used for the detection of HClO in tap water, medical wastewater and biological serum systems. The probe BNA-HClO was further successfully made into a portable test strip, which has the ability of semi-quantitative analytical detection. In addition, the probe BNA-HClO was successfully used for the imaging analysis of exogenous and endogenous HClO in living cells. In conclusion, the probe BNA-HClO provides a favorable tool for the study of HClO at the practical application level.
Informed consent statement
Informed consent was obtained from all subjects involved in the study.
Author contributions
Conceptualization, D. Q. L. and M. Z.; methodology, M. Z. and D. Q. L; validation, M. Z. and K. Y. G; investigation, W. D. H., M. Z and K. Y. G; resources, D. Q. L. and M. L.; data curation, W. D. H., M. Z. and K. Y. G; writing—original draft preparation, M. Z. and W. D. H.; writing—review and editing, W. D. H, L. W. X and D. Q. L; supervision, D. Q. L. All authors have read and agreed to the published version of the manuscript.
Conflicts of interest
There are no conflicts to declare.
Acknowledgements
This work was funded by the National Natural Science Foundation for Young Scientists of China (Grant 22004132), Natural Science Foundation for Young Scientists of Hunan Province (Grant 2020JJ5963), Provincial Key Research and Development Plan in Hunan, China (2020NK2019). Scientific Innovation Fund for Post-graduates of Central South University of Forestry and Technology, China (CX202102076).
References
- Z. M. Prokopowicz, F. Arce, R. Biedroń, C. L. Chiang, M. Ciszek, D. R. Katz, M. Nowakowska, S. Zapotoczny, J. Marcinkiewicz and B. M. Chain, J. Immunol., 2010, 184, 824–835 CrossRef CAS PubMed.
- S. Hammerschmidt, N. Büchler and H. Wahn, Chest, 2002, 121, 573 CrossRef CAS PubMed.
- S. M. Wu and S. V. Pizzo, Arch. Biochem. Biophys., 2001, 391(1), 119 CrossRef CAS PubMed.
- D. I. Pattison and M. J. Davies, Biochemistry, 2006, 45, 8152 CrossRef CAS PubMed.
- M. L. Kent, C. Buchner, C. Barton and R. L. Tanguay, Dis. Aquat. Org., 2014, 107(3), 235–240 CrossRef CAS PubMed.
- L. E. Beane Freeman, K. P. Cantor, D. Baris, J. R. Nuckols, A. Johnson, J. S. Colt, M. Schwenn, M. H. Ward, J. H. Lubin, R. Waddell, G. M. Hosain, C. Paul, R. McCoy, L. E. Moore, A. T. Huang, N. Rothman, M. R. Karagas and D. T. Silverman, Environ. Health Perspect., 2017, 125(6), 067010 CrossRef PubMed.
- S. E. Hrudey, L. C. Backer, A. R. Humpage, S. W. Krasner, D. S. Michaud, L. E. Moore, P. C. Singer and B. D. Stanford, J. Toxicol. Environ. Health, Part B, 2015, 18(5), 213 CAS.
- J. Zhang, X. Wang and X. Yang, Analyst, 2012, 137, 2806 RSC.
- S. Thiagarajan, Z. Y. Wu and S. M. Chen, J. Electroanal. Chem., 2011, 661(2), 322–328 CrossRef CAS.
- G. J. Mao, Y. Y. Wang, W. P. Dong, H. M. Meng, Q. Q. Wang, X. F. Luo, Y. Li and G. Zhang, Spectrochim. Acta, Part A, 2021, 249, 119326 CrossRef CAS PubMed.
- L. He, H. Q. Xiong, B. H. Wang, Y. Zhang, J. P. Wang, H. Y. Zhang, H. P. Li, Z. G. Yang and X. Z. Song, Anal. Chem., 2020, 92, 11029 CrossRef CAS PubMed.
- Y. Jiang, G. Zheng, Q. Duan, L. Yang, J. Zhang, H. Zhang, J. He, H. Sun and D. Ho, Chem. Commun., 2018, 54(57), 7967–7970 RSC.
- X. L. Jin, Y. F. Jin, W. X. Chen, P. Chui and Z. H. Yang, Sens. Actuators, B, 2016, 232, 300–305 CrossRef CAS.
- Y. W. Jun, S. Sarkar, S. Singha, Y. J. Reo, H. R. Kim, J. J. Kim, Y. T. Chang and K. H. Ahn, Chem. Commun., 2017, 28, 10800 RSC.
- X. Y. Li, L. Wu, Z. Y. Zhao, C. Y. Liu and B. C. Zhu, RSC Adv., 2019, 9, 4659–4664 RSC.
- C. Y. Liu, P. Jia, L. Wu, Z. L. Li, H. C. Zhu, Z. K. Wang, S. Deng, W. Shu, X. Zhang, Y. M. Yu and B. C. Zhu, Sens. Actuators, B, 2019, 297, 126731 CrossRef CAS.
- L. F. Pang, Y. M. Zhou, W. L. Gao, H. H. Song, X. Wang and Y. Wang, RSC Adv., 2016, 6, 105795 RSC.
- L. Shi, H. J. Yu, X. Q. Zeng, S. Yang, S. Z. Gong, H. Xiang, K. Zhang and G. Shao, New J. Chem., 2020, 44, 6232 RSC.
- X. Wang, Y. Zhou, C. Xu, H. Song, L. Li, J. Zhang and M. Guo, Spectrochim. Acta, Part A, 2018, 203, 415 CrossRef CAS PubMed.
- L. L. Wu, Q. Y. Yang and L. Y. Liu, Chem. Commun., 2018, 54(61), 8522–8525 RSC.
- Y. Zhang, X. Q. Zhang, H. C. Yang, L. Yu, Y. J. Xu, A. Sharma, P. Yin, X. Y. Li, J. S. Kim and Y. Sun, Chem. Soc. Rev., 2021, 50, 11227 RSC.
- X. Yang, Q. H. Ou, K. Qian, J. R. Yang, Z. X. Bai, W. Y. Yang, Y. M. Shi and G. Liu, Coordin. Chem. Rev., 2021, 443, 214017 CrossRef.
- P. Z. Wang, H. C. Yang, C. Liu, M. Q. Qiu, X. Ma, Z. Q. Mao, Y. Sun and Z. H. Liu, Chin. Chem. Lett., 2021, 32, 168 CrossRef CAS.
- F. Ding, Z. Chen, W. Y. Kim, A. Sharma, C. L. Li, Q. Y. Ouyang, H. Zhu, G. F. Yang, Y. Sun and J. S. Kim, Chem. Sci., 2019, 10, 7023 RSC.
- K. Wang, D. Xi and C. Liu, et al., Chin. Chem. Lett., 2020, 31(11), 2955–2959 CrossRef CAS.
- J. Ouyang, Y. F. Li, W. L. Jiang, S. Y. He, H. W. Liu and C. Y. Li, Anal. Chem., 2019, 91, 1056–1063 CrossRef CAS PubMed.
- W. J. Huang, H. C Yang, Z. X. Hu, Y. F. Fan, X. F. Guan, W. Q. Feng, Z. H. Liu and Y. Sun, Adv. Healthcare. Mater., 2021, 10, 2101003 CrossRef CAS PubMed.
- L. He, H. Q. Xiong and B. H. Wang, Anal. Chem., 2020, 16, 11029–11034 CrossRef PubMed.
- Y. Jiang, G. S. Zheng and Q. Y. Duan, Chem. Commun., 2018, 54(57), 7967–7970 RSC.
- X. L. Jin, Y. F. Jia and W. X. Chen, Sens. Actuators, B, 2016, 232, 300–305 CrossRef CAS.
- Y. W. Jun, S. Sarkar and S. Singha, Chem. Commum., 2017, 53(78), 10800–10803 RSC.
- X. Y. Li, L. Wu and Z. Y. Hao, RSC Adv., 2019, 9(8), 4659–4664 RSC.
- C. Y. Liu, P. Jia and L. Wu, Sens. Actuators, B, 2019, 297, 126731 CrossRef CAS.
- L. F. Pang, Y. M. Zhou and W. L. Gao, RSC Adv., 2016, 6, 105795–105800 RSC.
- L. Shi, H. J. Yu and X. Q. Zeng, New J. Chem., 2020, 44, 6232–6237 RSC.
- X. Wang, Y. M. Zhou and C. G. Xu, Spectrochim. Acta, Part A, 2018, 203, 415–420 CrossRef CAS PubMed.
- L. L. Wu, Q. Y. Yang and L. Y. Liu, Chem. Commun., 2018, 54(61), 8522–8525 RSC.
- D. Q. Lu, L. Y. Zhou, R. W. Wang, X. B. Zhang, L. He, J. Zhang, X. X. Hu and W. H. Tan, Sens. Actuators, B, 2017, 250, 259 CrossRef CAS.
- X. Y. Li, L. Wu, Z. Y. Zhao, C. Y. Liu and B. C. Zhu, RSC Adv., 2019, 9, 4659 RSC.
- B. C. Zhu, L. Wu, M. Zhang, Z. Y. Zhao, Z. K. Wang, Q. X. Duan, P. Jia, L. Li, H. C. Zhu and C. Y. Liu, Sens. Actuators, B, 2018, 267, 589 CrossRef CAS.
- B. C. Zhu, P. Li, W. Shu, X. Wang, C. Y. Liu, Y. Wang, Z. K. Wang, Y. W. Wang and B. Tang, Anal. Chem., 2016, 88, 12532 CrossRef CAS PubMed.
- G. J. Mao, T. T. Wei, X. X. Wang, S. S. Huan, D. Q. Lu, J. Zhang, X. B. Zhang, W. H. Tan, G. L. Shen and R. Q. Yu, Anal. Chem., 2013, 85, 7875 CrossRef CAS PubMed.
- Standardization Administration of the People's Republic of China (SAC) has set the drinking water sanitary standard (GB5749-2006), http://www.sac.gov.cn/ Search PubMed.
- Standardization Administration of the People's Republic of China (SAC) has set Discharge standards of water pollutants of medical organization (GB18466-2016), http://www.sac.gov.cn/ Search PubMed.
Footnote |
† Electronic supplementary information (ESI) available. See DOI: 10.1039/d1ra08116k |
|
This journal is © The Royal Society of Chemistry 2022 |
Click here to see how this site uses Cookies. View our privacy policy here.