DOI:
10.1039/D1RA08579D
(Paper)
RSC Adv., 2022,
12, 785-789
Pd-catalyzed [3 + 2] cycloaddition of cyclic ketimines and trimethylenemethanes toward N-fused pyrrolidines bearing a quaternary carbon†
Received
23rd November 2021
, Accepted 17th December 2021
First published on 24th December 2021
Abstract
A Pd-catalyzed [3 + 2] cycloaddition of N-sulfonyl cyclic ketimines and trimethylenemethanes (TMM) was developed that afforded N-fused pyrrolidines bearing a quaternary carbon. Under mild reaction conditions, structurally diverse N-sulfonyl cyclic imines, including sulfamate-fused aldimines, aryl- or styryl-substituted sulfamate-derived ketimines, and N-sulfonyl cyclic ketimines, were tolerated as reactants, affording N-fused pyrrolidines with high efficiency.
Introduction
Heterocycles bearing quaternary carbon stereocenters are found in a wide range of biologically active natural compounds and pharmaceuticals.1 In particular, alkaloids containing N-fused pyrrolidines with quaternary carbons have been observed to exhibit a wide range of biological properties (Fig. 1), such as anti-tumoral and anti-inflammatory activities, as well as the ability to potently reverse the multidrug resistance of cancers.2 Given the pronounced bioactivities of marketed drugs comprising the pyrrolidine scaffold,3 over the past three decades or so, many synthetic methods have been developed to access pyrrolidines.4 However, few reports have been published on the synthesis of N-fused pyrrolidine bearing quaternary carbons.5 One of the reasons that renders the construction of quaternary carbons a challenging task in organic synthesis is their congested nature.6 Considering the specific bioactivities of quaternary carbon centers in heterocycles, there is clearly an unmet need for convenient and efficient methods for the synthesis of quaternary carbon-containing pyrrolidines.
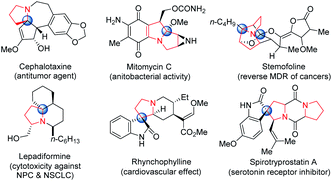 |
| Fig. 1 Representative alkaloids containing N-fused pyrrolidines bearing quaternary carbons. | |
Transition metal-catalyzed dipolar cycloadditions have proven to be efficient and practical approaches to the synthesis of valuable cyclic compounds.7 In particular, the recently developed approach whereby sulfamate-fused imines are used in cycloaddition reactions appears to be an extremely promising strategy for the synthesis of N-fused heterocycles. The sulfamate moiety is an important structural motif in pharmacology due to its potent bioactivities, such as anticancer, antibiotic, and antiviral properties; moreover, this moiety serves as a reactive intermediate that can be readily converted to other useful heterocycles.8 Accordingly, significant efforts have been devoted to developing cycloadditions of sulfamate-derived aldimines coupled with various types of dipoles, affording sulfamate-functionalized heterocycles characterized by diverse ring sizes.9 For the preparation of sulfamate-fused pyrrolidines, the Pd-catalyzed [3 + 2] cycloaddition between vinylcyclopropanes and sulfamate-derived aldimines has been developed, which demonstrated high efficiency under mild reaction conditions (Scheme 1a).10 An asymmetric strategy based on the organocatalytic Mannich/Aza-Michael cascade reaction using cis δ-formyl α,β-unsaturated ketones with sulfamate-derived aldimines has also been reported (Scheme 1b).11 In stark contrast to the cycloadditions of sulfamate-derived aldimines, only recently have a few examples of catalytic cycloadditions of sulfamate-derived ketimines generating quaternary carbons in nitrogen-containing heterocycles been reported, and they involved a limited number of ketimines, such as cyclic N-sulfonyl trifluoromethylated ketimines12 and styryl-substituted ketimines.13 The variety of examples of such reactions possibly descends from the inherent lability and inactivity of ketimine derivatives.14 In the present study, we established a Pd-catalyzed [3 + 2] cycloaddition affording sulfamate-fused pyrrolidines bearing a quaternary carbon, starting from commercially available trimethylenemethane (TMM) and sulfamate-derived ketimines serving as prochiral sp2-carbons (Scheme 1c).
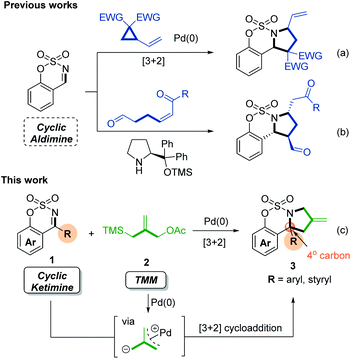 |
| Scheme 1 Synthetic approaches implemented to access sulfamate-fused pyrrolidines. | |
Since Trost first reported the use of TMMs in Pd-catalyzed cycloadditions, a variety of [3 + n] cycloadditions reacting with dipolarophiles, such as activated alkenes, aldehydes, ketones, and acyclic imines, have been developed.15 In the presence of Pd(0) species, a TMM can form a zwitterionic Pd complex, which can be considered a C-nucleophilic/C-electrophilic 1,3-dipole intermediate. Accordingly, the utilization of TMMs in Pd catalysis has emerged as a reliable synthetic strategy for providing three-carbon synthons for the preparation of various carbocycles and heterocycles. Surprisingly, despite the great importance of the sulfamate moiety and recent advances in the catalytic cycloadditions of sulfamate-derived imines,8,9 the Pd-catalyzed cycloaddition of sulfamate-derived cyclic imines achieved employing TMMs as reactive 1,3-dipoles has never been reported. Herein, we describe an efficient Pd-catalyzed [3 + 2] cycloaddition of a TMM with both sulfamate-derived cyclic aldimines and ketimines conducted under mild reaction conditions.
Results and discussion
Initially, to test the feasibility of the [3 + 2] cycloaddition of sulfamate-derived cyclic imines and a TMM, the reaction of the cyclic aldimine 1a with 1.5 equiv. of TMM 2 was attempted in the presence of a Pd catalyst. On the basis of previously published studies,15,16 we chose tris(dibenzylideneacetone)dipalladium(0) (Pd2(dba)3) as the palladium source, and we screened the effects of various ligands on the catalytic reaction conducted in toluene at 60 °C (see the ESI for details†). We observed that the reaction efficiency was largely dependent on the identity of the ligand. Among the screened phosphine and phosphoramidite ligands, phosphoramidite L1 was found to be the most effective for this Pd-catalyzed [3 + 2] cycloaddition reaction; by employing it, the desired product 3a was afforded in 97% isolated yield. The use of other Pd(II) catalysts, such as Pd(OAc)2 and PdCp(η3-allyl), and solvents was also investigated in the presence of L1 at 60 °C, but none of these catalysts and solvents afforded yields higher than that obtained using Pd2(dba)3 in toluene. Moreover, reducing the equiv. of 2 or decreasing the reaction temperature were observed to be associated with decreases in the yield of 3a (see the ESI for details†).
With the optimized reaction conditions in hand, we first examined the Pd-catalyzed [3 + 2] cycloaddition reactions of various cyclic aldimines 1 and 2 to investigate the scope of the reaction (Table 1). Sulfamate-derived cyclic aldimines 1a–g bearing electron-donating (–Me, –OMe) and electron-withdrawing (–Cl, –F) groups in different positions of the arene were well tolerated in this catalytic system, producing sulfamate-fused pyrrolidines 3a–g in 82–97% yields.
Table 1 Scope of the cycloaddition reaction involving sulfamate-derived cyclic aldiminea
Reaction conditions: 1 (0.1 mmol), 2 (0.15 mmol), Pd2(dba)3 (2.5 mol%), and L1 (11 mol%) in toluene (1.0 mL) at 60 °C for 12 h under argon, isolated yields after column chromatography. |
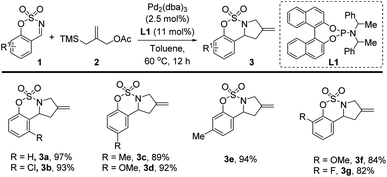 |
Encouraged by these results, we focused on the cycloadditions between cyclic ketimines 4 and TMMs to afford N-fused pyrrolidine derivatives bearing a quaternary carbon. We applied the reaction conditions optimized using aldimines 1 to the reaction of ketimine 4a with 2, and we observed the production of cyclic adduct 5a in excellent yield. Notably, the reaction could be conducted in milder catalytic conditions than those implemented in the reaction between 1 and 2, even though ketimines 4 are considered less reactive substrates than aldimines 1.14 After further optimization, the desired product 5a was obtained in 95% yield by using 1.2 equiv. of 2 at 30 °C.
Subsequently, a wide range of cyclic ketimines 4 were tested as substrates using 2 under mild reaction conditions (Table 2). The reactions of the sterically demanding ketimine 4b and ketimines 4c–g, which bear electron-donating (–Me, –tBu, –OMe) or halogen (–F, –Cl) substituents at the 6-position on the phenyl ring, proceeded efficiently to afford sulfamate-fused pyrrolidines 5b–g comprising a quaternary carbon in 83–98% yields, although in the cases of substrates 4d and 4f the amount of TMM had to be increased. The presence of a bromide substituent on the aryl ketimine was tolerated in this Pd-catalysis; in the relevant case, the corresponding product 5h was obtained in 96% yield. The ketimines containing different substituents at the 6- and 7-positions of the aryl moiety of 4 smoothly underwent the [3 + 2] cycloaddition to afford N-fused pyrrolidines 5i–m in excellent yields, up to 99%; moreover, the structure of 5i was determined by X-ray diffraction analysis of a single crystal. A range of different R2 substituents on ketimines 4 were also tested; evidence indicated that use of various para-substituted phenyls with different electronic properties afforded the corresponding products 5n–s in good-to-excellent yields. In particular, ketimines 4p and 4q, comprising the ester and cyanide groups, respectively, proved compatible with the present Pd-catalyzed [3 + 2] cycloaddition. To our delight, the described cycloaddition reaction also tolerated the α,β-unsaturated ketimine 4t, in which an electron-deficient alkene can participate in the cycloaddition to provide a cyclopentane derivative.17 Indeed, the desired product 5t was selectively obtained in good yield. The reagents compatible with this catalytic reaction were not limited to sulfamate-derived ketimines, and use of the N-sulfonyl cyclic ketimine 4u provided N-sulfonyl pyrrolidine 5u in 89% yield.
Table 2 Scope of the cycloaddition reaction involving sulfamate-derived cyclic ketiminesa
Reaction conditions: 4 (0.1 mmol), 2 (0.12 mmol), Pd2(dba)3 (2.5 mol%), and L1 (11 mol%) in toluene (1.0 mL) at 30 °C under argon, 20 h, isolated yields after column chromatography. 2 (0.15 mmol) was added. |
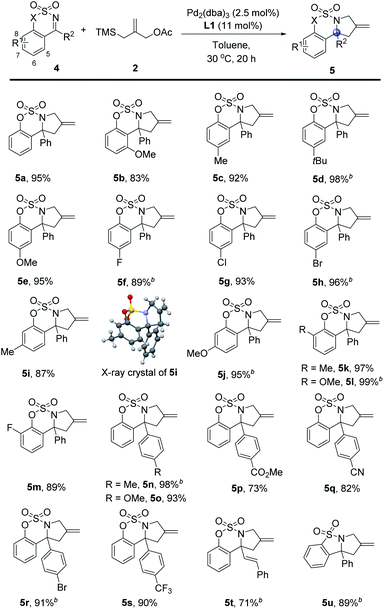 |
We then investigated the Pd-catalyzed asymmetric [3 + 2] cycloaddition of cyclic ketimine 4a and TMM 2, and the preliminary results of a ligand screening effort are reported in Scheme 2. The different phosphoramidite ligands bearing the 1,1′-bi-2-naphthol (BINOL) backbone (L1–4) and the octahydro-1,1′-2-naphthol backbone (L5–7) were employed in the reaction between 4a and 2 affording the sulfamate-fused pyrrolidine 5a comprising a stereogenic center. Unfortunately, only a 20% ee value for compound 5a was obtained when the chiral phosphoramidite L1 was employed, and only a slight increase in enantioselectivity (28% ee) was achieved conducting the cycloaddition reaction at 0 °C.
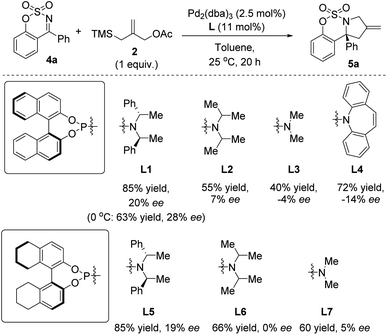 |
| Scheme 2 Results of the investigation on the feasibility of the asymmetric [3 + 2] cycloaddition. | |
Next, to demonstrate that the reaction is a practical protocol for the synthesis of pyrrolidine derivatives, a gram-scale reaction between ketimine 4a and 2 was performed. To our delight, the reaction proceeded smoothly, delivering the corresponding pyrrolidine 5a in 97% yield without the loss of reactivity (Scheme 3a). To probe the utility of the synthesized pyrrolidine 5a, which contains the versatile exocyclic double bond, organic transformations aimed at producing pyrrolidines bearing two stereogenic centers were attempted. The exocyclic double bond in 5a could be readily converted to the methyl substituted pyrrolidine 6a in 85% yield with 3
:
1 diastereomeric ratio by hydrogenation (Scheme 3b). Bromination of 5a with Br2 (3 equiv.) in CHCl3 afforded the vicinal dibromides product 6b18 containing two quaternary carbons in 91% yield (Scheme 3c).
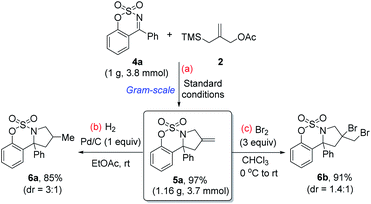 |
| Scheme 3 Gram-scale synthesis of compound 5a and its transformation. | |
Conclusions
In summary, we have developed a facile method for the synthesis of N-fused pyrrolidines via Pd-catalyzed [3 + 2] cycloaddition of N-sulfonyl imines and a commercially available TMM. The reaction proceeded efficiently under mild conditions, and afforded N-fused pyrrolidines, which are important skeletons of biologically active compounds. Notably, a wide range of ketimines (e.g., sulfamate-derived ketimines bearing aryl and styryl substituents and N-sulfonyl cyclic ketimine) were tolerated as substrates, providing N-sulfonyl pyrrolidines comprising quaternary carbon centers in good-to-excellent yields. To demonstrate the scalability and the utility of the synthesized product, sulfamated-fused pyrrolidine with exocyclic double bond derived from TMM, a gram-scale reaction and synthetic transformations were performed.
Experimental
General procedure for the synthesis of compound 3
To a flame-dried Schlenk tube, Pd2(dba)3 (2.3 mg, 2.5 mol%) and L1 (6.0 mg, 11 mol%) were added in a glove box; subsequently, aldimine 1 (0.1 mmol), 2-((trimethylsilyl)methyl)allyl acetate 2 (0.15 mmol), and toluene (1.0 mL) were added under argon atmosphere. The reaction mixture was stirred at 60 °C for 12 h. After completion of the reaction, the solvent was removed by evaporation, and the product was isolated by silica gel column chromatography using an appropriate eluent. The product yields were determined by 1H NMR analysis.
General procedure for the synthesis of compound 5
To a flame-dried Schlenk tube, Pd2(dba)3 (2.3 mg, 2.5 mol%) and L1 (6.0 mg, 11 mol%) were added in a glove box; subsequently, ketimine 4 (0.1 mmol), 2-((trimethylsilyl)methyl)allyl acetate 2 (0.12 mmol), and toluene (1.0 mL) were added under argon atmosphere. The reaction mixture was stirred at 30 °C for 20 h. After completion of the reaction, the solvent was removed by evaporation, and the product was isolated by silica gel column chromatography using an appropriate eluent. The product yields were determined by 1H NMR analysis.
Conflicts of interest
There are no conflicts to declare.
Acknowledgements
This work was financially supported by National Research Foundation (NRF-2020R1F1A1068452, NRF-2020R1A4A2002831) and Korea Basic Science Institute (KBSI) National Research Facilities & Equipment Center (NFEC) grant funded by the Korea government (Ministry of Education) (No. 2019R1A6C1010042).
Notes and references
-
(a) Z.-P. Wang, Q. Wu, J. Jiang, Z.-R. Li, X.-J. Peng, P.-L. Shao and Y. He, Org. Chem. Front., 2018, 5, 36–40 RSC;
(b) X. Wu and H. Ji, J. Org. Chem., 2018, 83, 4650–4656 CrossRef CAS PubMed.
-
(a) S. M. Weinreb, Chem. Rev., 2006, 106, 2531–2549 CrossRef CAS PubMed;
(b) S. E. Wolkenberg and D. L. Boger, Chem. Rev., 2002, 102, 2477–2496 CrossRef CAS PubMed;
(c) X.-Z. Huang, L.-H. Gao and P.-Q. Huang, Nat. Commun., 2020, 11, 5314 CrossRef CAS PubMed;
(d) J. Xu, L.-D. Shao, X. Shi, J. Ren, C. Xia and Q.-S. Zhao, RSC Adv., 2016, 6, 63131–63135 RSC;
(e) H. Abe, S. Aoyagi and C. Kibayashi, Tetrahedron Lett., 2000, 41, 1205–1208 CrossRef CAS;
(f) S. G. Pyne, A. Jatisatienr, P. Mungkornasawakul, A. T. Ung, P. Limtrakul, T. Sastraruji, K. Sastraruji, S. Chaiyong, S. Umsumarng, M. C. Baird, X. D. Dau and R. A. Ramli, Nat. Prod. Commun., 2017, 12, 1365–1369 CrossRef;
(g) P. Kumari, W. Liu, C.-J. Wang, J. Dai, M.-X. Wang, Q.-Q. Yang, Y.-H. Deng and Z. Shao, Chin. J. Chem., 2020, 38, 151–157 CrossRef CAS.
-
(a) L. Tian, G.-Q. Xu, Y.-H. Li, Y.-M. Liang and P.-F. Xu, Chem. Commun., 2014, 50, 2428–2430 RSC;
(b) E. Vitaku, D. T. Smith and J. T. Njardarson, J. Med. Chem., 2014, 57, 10257–10274 CrossRef CAS PubMed;
(c) C.-V. T. Vo and J. W. Bode, J. Org. Chem., 2014, 79, 2809–2815 CrossRef CAS PubMed;
(d) D. Wang, W. Liu, Y. Hong and X. Tong, Org. Lett., 2018, 20, 5002–5005 CrossRef CAS PubMed;
(e) D. Habel, D. S. Nair, Z. Kallingathodi, C. Mohan, S. M. Pillai, R. R. Nair, G. Thomas, S. Haleema, C. Gopinath, R. V. Abdul, M. Fritz, A. R. Puente, J. L. Johnson, P. L. Polavarapu and I. Ibnusaud, J. Nat. Prod., 2020, 83, 2178–2190 CrossRef CAS PubMed;
(f) G. L. Petri, M. V. Raimondi, V. Spano, R. Holl, P. Barraja and A. Montalbano, Top. Curr. Chem., 2021, 379, 34 CrossRef PubMed.
-
(a) L. E. Overman, Acc. Chem. Res., 1992, 25, 352–359 CrossRef CAS;
(b) I. Coldham and R. Hufton, Chem. Rev., 2005, 105, 2765–2810 CrossRef CAS PubMed;
(c) P. Compain, Adv. Synth. Catal., 2007, 349, 1829–1846 CrossRef CAS;
(d) A. L. Cardoso and T. M. V. D. Pinho e Melo, Eur. J. Org. Chem., 2012, 2012, 6479–6501 CrossRef CAS;
(e) D. M. Schultz and J. P. Wolfe, Synthesis, 2012, 44, 351–361 CrossRef CAS PubMed;
(f) J. L. Jeffrey and R. Sarpong, Chem. Sci., 2013, 4, 4092–4106 RSC;
(g) I. Kumar, RSC Adv., 2014, 4, 16397–16408 RSC;
(h) S. W. M. Crossley and R. A. Shenvi, Chem. Rev., 2015, 115, 9465–9531 CrossRef CAS PubMed;
(i) A. K. Mailyan, J. A. Eickhoff, A. S. Minakova, Z. Gu, P. Lu and A. Zakarian, Chem. Rev., 2016, 116, 4441–4557 CrossRef CAS PubMed;
(j) B. L. Pagenkopf and N. Vemula, Eur. J. Org. Chem., 2017, 2017, 2561–2567 CrossRef CAS;
(k) J. Robertson and K. Stevens, Nat. Prod. Rep., 2017, 34, 62–89 RSC.
-
(a) N. V. Shymanska and J. G. Pierce, Org. Lett., 2017, 19, 2961–2964 CrossRef CAS PubMed;
(b) B. M. Trost, T. M. Lam and M. A. Herbage, J. Am. Chem. Soc., 2013, 135, 2459–2461 CrossRef CAS PubMed;
(c) A. J. Boddy, D. P. Affron, C. J. Cordier, E. L. Rivers, A. C. Spivey and J. A. Bull, Angew. Chem., Int. Ed., 2019, 58, 1458–1462 CrossRef CAS PubMed;
(d) L. R. E. Pantaine, J. A. Milligan, J. K. Matsui, C. B. Kelly and G. A. Molander, Org. Lett., 2019, 21, 2317–2321 CrossRef CAS PubMed.
- C. J. Douglas and L. E. Overman, Proc. Natl. Acad. Sci. U. S. A., 2004, 101, 5363–5367 CrossRef CAS PubMed.
-
(a) J. Adrio and J. C. Carretero, Chem. Commun., 2011, 47, 6784–6794 RSC;
(b) K. V. Gothelf and K. A. Jørgensen, Chem. Rev., 1998, 98, 863–909 CrossRef CAS PubMed.
- J.-Y. Winum, A. Scozzafava, J.-L. Montero and C. T. Supuran, Med. Res. Rev., 2005, 25, 186–228 CrossRef CAS PubMed.
- L. Zhang, H. Yu, Z. Yang, H. Liu, Z. Li, J. Guo, Y. Xiao and H. Guo, Org. Biomol. Chem., 2013, 11, 8235–8240 RSC.
- K. Spielmann, E. Tosi, A. Lebrun, G. Niel, A. v. d. Lee, R. M. d. Figueiredo and J.-M. Campagne, Tetrahedron, 2018, 74, 6497–6511 CrossRef CAS.
- H. Kim, Y. Kim and S.-G. Kim, J. Org. Chem., 2017, 82, 8179–8185 CrossRef CAS PubMed.
- Z.-Z. Zhang, Y. Zhang, H.-X. Duan, Z.-F. Deng and Y.-Q. Wang, Chem. Commun., 2020, 56, 1553–1556 RSC.
- X. Gao, D. Zhu, F. Jiang, J. Liao, W. Wang, Y. Wu, L. Zheng and H. Guo, Org. Biomol. Chem., 2021, 19, 4877–4881 RSC.
-
(a) V. A. Sukach, N. M. Golovach, V. V. Pirozhenko, E. B. Rusanow and M. V. Vovk, Tetrahedron: Asymmetry, 2008, 19, 761–764 CrossRef CAS;
(b) C. Homma, M. Yamanaka, T. Kano and K. Maruoka, Tetrahedron, 2021, 91, 132225 CrossRef CAS;
(c) G. Lupidi, A. Palmieri and M. Petrini, Adv. Synth. Catal., 2021, 363, 3655–3692 CrossRef CAS;
(d) J. Kim, M. Shin and S. H. Cho, ACS Catal., 2019, 9, 8503–8508 CrossRef CAS;
(e) C. Xu, C. Reep, J. Jarvis, B. Naumann, B. Captain and N. Takenaka, Catalyst, 2021, 11, 712 CrossRef CAS PubMed;
(f) S. Saaby, K. Nakama, M. A. Lie, R. G. Hazell and K. A. Jørgensen, Chem.–Eur. J., 2003, 9, 6145–6154 CrossRef CAS PubMed;
(g) M. Shimizu, J. Kikuchi, A. Kondoh and M. Terada, Chem. Sci., 2018, 9, 5747–5757 RSC;
(h) N. Goswami, T. Bhattacharya and D. Maiti, Nat. Rev. Chem., 2021, 5, 646–659 CrossRef CAS;
(i) H. Jang, F. Romiti, S. Torker and A. H. Hoveyda, Nat. Chem., 2017, 9, 1269–1275 CrossRef CAS PubMed.
-
(a) B. M. Trost and D. M. T. Chan, J. Am. Chem. Soc., 1979, 101, 6432–6433 CrossRef CAS;
(b) B. M. Trost and G. Mata, Acc. Chem. Res., 2020, 53, 1293–1305 CrossRef CAS PubMed;
(c) B. M. Trost, S. M. Silverman and J. P. Stambuli, J. Am. Chem. Soc., 2007, 129, 12398–12399 CrossRef CAS PubMed.
-
(a) H.-I. Ahn, J.-U. Park, Z. Xuan and J. H. Kim, Org. Biomol. Chem., 2020, 18, 9826–9830 RSC;
(b) J.-U. Park, H.-I. Ahn, H. J. Cho, Z. Xuan and J. H. Kim, Adv. Synth. Catal., 2020, 362, 1836–1840 CrossRef CAS.
-
(a) Y. Lin, Q. Wang, Y. Wu, C. Wang, H. Jia, C. Zhang, J. Huang and H. Guo, RSC Adv., 2018, 8, 40789–40803 Search PubMed;
(b) H. Lin, H. Yang, Q. Gong, S. Luo, J. Gu, X. Cao, B. Mao, Y. Ge and C. Yuan, RSC Adv., 2021, 11, 20118–20122 RSC;
(c) Q. Zhou, B. Chen, X.-B. Huang, Y.-L. Zeng, W.-D. Chu, L. He and Q.-Z. Liu, Org. Chem. Front., 2019, 6, 1891–1894 RSC;
(d) L. Yu, Y. Cheng, F. Qi, R. Li and P. Li, Org. Chem. Front., 2017, 4, 1336–1340 RSC;
(e) Y. Wu, Y. Liu, W. Yang, H. Liu, L. Zhou, Z. Sun and H. Guo, Adv. Synth. Catal., 2016, 358, 3517–3521 CrossRef CAS;
(f) X.-L. He, Y.-C. Xiao, W. Du and Y.-C. Chen, Chem.–Eur. J., 2015, 21, 3443–3448 CrossRef CAS PubMed.
- I. Saikia, A. J. Borah and P. Phukan, Chem. Rev., 2016, 116, 6837–7042 CrossRef CAS PubMed.
Footnotes |
† Electronic supplementary information (ESI) available: Experimental details, reaction optimization, characterization data, crystallographic data of 5i (CCDC 2114538), and 1H and 13C NMR spectra of all products. CCDC 2114538. For ESI and crystallographic data in CIF or other electronic format see DOI: 10.1039/d1ra08579d |
‡ The authors contributed equally to this work. |
|
This journal is © The Royal Society of Chemistry 2022 |
Click here to see how this site uses Cookies. View our privacy policy here.