DOI:
10.1039/D2RA00298A
(Paper)
RSC Adv., 2022,
12, 8394-8403
Initiating a high-temperature zinc ion battery through a triazolium-based ionic liquid†
Received
15th January 2022
, Accepted 2nd March 2022
First published on 16th March 2022
Abstract
Triazolium-based ionic liquids (T1, T2 and T3) with or without terminal hydroxyl groups were prepared via Cu(I) catalysed azide–alkyne click chemistry and their properties were investigated using various technologies. The hydroxyl groups obviously affected their physicochemical properties, where with a decrease in the number of hydroxyl groups, their stability and conductivity were enhanced. T1, T2 and T3 showed relatively high thermal stability, and their electrochemical stability windows (ESWs) were 4.76, 4.11 and 3.52 V, respectively. T1S-20 was obtained via the addition of zinc trifluoromethanesulfonic acid (Zn(CF3SO3)2) and lithium bis(trifluoromethanesulfonyl)imide (LiTFSI) to T1, displaying conductivity and ESW values of 1.55 × 10−3 S cm−1 and 6.36 V at 30 °C, respectively. Subsequently, a Zn/Li3V2(PO4)3 battery was assembled using T1S-20 as the electrolyte and its performances at 30 °C and 80 °C were investigated. The battery showed a capacity of 81 mA h g−1 at 30 °C, and its capacity retention rate was 89% after 50 cycles. After increasing the temperature to 80 °C, its initial capacity increased to 111 mA h g−1 with a capacity retention rate of 93.6% after 100 cycles, which was much higher than that of the aqueous electrolyte (WS-20)-based zinc ion battery (71.8%). Simultaneously, the T1S-20 electrolyte-based battery exhibited a good charge/discharge efficiency, and its Coulomb efficiency was 99%. Consequently, the T1S-20 electrolyte displayed a better performance in the Zn/Li3V2(PO4)3 battery than that with the aqueous electrolyte, especially at high temperature.
Introduction
Zinc ion batteries (ZIBs) have been widely investigated due to their high theoretical capacity, cyclic stability, energy density and rate performance.1–3 Besides, they can be easily assembled in air and are safer and more environmentally friendly than lithium-ion batteries, especially with the emergence of aqueous ZIBs.4,5 However, water-induced side reactions hinder their further development, such as hydrogen/oxygen evolution reactions, dissolution of cathode materials, and narrow electrochemical stability window (ESW). In addition, the growth of dendrites needs to be suppressed.6,7 Although high-performance cathodes have been developed to overcome the above-mentioned problems,8–10 the electrolyte also directly affects the performance of ZIBs.11 Concentrated salt and other additives (organic solvents and ionic liquids)12–15 were incorporated in aqueous electrolytes by tuning the solvation sheath of Zn2+ to promote the plating/stripping and migration of Zn2+.16 However, the water-induced side reactions are inevitable, and thus the development of new electrolytes without water, such as organic electrolytes and solid (or quasi-solid) electrolytes, is very important.17
Ionic liquids (ILs), which are obtained via the quaternization of molecules containing nitrogen, phosphorus, sulfur atoms, etc., possess the properties of low vapor pressure, appropriate conductivity, wide ESW and non-flammable nature, and hence can be considered safer than conventional organic electrolytes and better electrode interfacial contacts than solid electrolytes. However, the relatively poor stability of common ILs at high temperature limits their application.18 Among them, imidazolium ILs are the most widely investigated,19 where an improvement in their stability is usually realized via C-2 substitution but their functionalization is relative tedious.20 Triazolium ILs possess similar chemical structures to that of imidazolium ILs but show higher stability and wider ESW,20–22 and the emergence of azide–alkyne click chemistry allows the convenient and flexible functionalization of triazolium. To date, triazolium ILs have been studied as conductive units in polyelectrolytes,23,24 but seldomly reported as IL electrolytes for electrochemical energy storage devices. As is known, the main shortcoming of ILs is their high viscosity, which limits their conductivity and hinders the diffusion of ions, and thus some strategies have been developed to overcome this, such as the addition of water or conventional organic electrolytes. Increasing the temperature provides another effective strategy for improving the conductivity and accelerating the migration of ions. Meanwhile, high-temperature ZIBs have rarely been reported, with the focus on conductive iongel or organohydrogel electrolyte.25–27 In this contribution, triazolium-based ILs, 1, 2, and 3, with different amounts of hydroxyl groups were synthesized via Cu(I)-catalysed azide–alkyne click chemistry, where we anticipated that the presence of oxygen atoms could enhance the flexibility of their side chain due to the fact that the ether group possesses a higher degree of conformational freedom than alkyl chains.28 This is beneficial for dissolving metal ions and improving the conductivity,29 similar to alkali-metal salt dissolved in polyether, and the influence of their molecular structure on their properties were investigated to evaluate their application in ZIBs, especially at high temperature.
Experimental
Materials
Triethylene glycol monomethyl ether, propargyl bromide, NaH (60%), iodomethane (CH3I), triethylene glycol, L-ascorbic acid (Vc) and CuSO4·5H2O were purchased from Shanghai Adamas Reagent Company. Lithium bis(trifluoromethanesulfonyl)imide (LiTFSI) and zinc trifluoromethanesulfonate (Zn(CF3SO3)2) were purchased from Shanghai Energy Chemical Co., LTD. Li3V2(PO4)3, compound 5, and compound 8 were prepared according to the literature.30–32
Characterization
NMR spectra were recorded on a Bruker DPX500 spectrometer using tetramethyl silane as an internal standard in CDCl3 or DMSO-d6. Thermal gravimetric analysis (TGA) was performed using a Mettler-Toledo TGA 2 instrument under an argon flow at a heating rate of 10 °C min−1 from 30 °C to 800 °C. Differential scanning calorimetry (DSC) was performed on a Netzsch 204F1 in a nitrogen atmosphere. An indium standard was used for the temperature and enthalpy calibrations. The sample was first heated from 25 °C to 150 °C and held at this temperature for 3 min to eliminate the thermal history, and then cooled to −90 °C and heated again from −90 °C to 100 °C, at a heating or cooling rate of 10 °C min−1. Conductivity was measured according to our previous method33 via electrochemical impedance spectroscopy (EIS) on a CHI 760E electrochemical workstation (Shanghai Chenhua Equipment, China) with an AC amplitude of 10 mV in the frequency range of 0.01 Hz to 100 kHz, and the conductivity ‘σ’ was calculated using the formula σ = D/(SR), where ‘D’ is the thickness of the sample and ‘S’ is the area of the sample.
The assembly of CR2016-type coin cells was as follows: a slurry consisting of Li3V2(PO4)3 (70 wt%), acetylene black (20 wt%), polyvinylidene fluoride (10 wt%), and N-methylpyrrolidone was pasted on stainless steel mesh, then dried at 80 °C for 12 h, and finally cut into round pieces as cathodes. The mass loading of Li3V2(PO4)3 was approximately 2 mg per piece. CR2016-type coin cells were assembled in an open atmosphere by sandwiching a Whatman filter paper soaked with electrolyte between the prepared Li3V2(PO4)3 cathode and zinc foil anode.
The ESWs of the ILs were measured on a CHI760E electrochemical workstation via linear sweep voltammetry (LSV) at a scanning rate of 1 mV s−1 in different voltage ranges by sandwiching the electrolyte between a stainless steel sheet cathode and zinc foil anode. Cyclic voltammetry (CV) measurement of the CR2016-type coin cells was carried out on a CHI760E electrochemical workstation at a scanning rate of 0.1 mV s−1. The charge/discharge performance of the CR2016-type coin cells was tested on a Neware battery test system (Shenzhen Neware Electronics Co., Ltd). The cycle stability of the ZIBs at the rate of 0.2 A g−1 and their rate performance at different current densities (0.2, 0.3, 0.5, 1, and 1.5 A g−1) were tested in the voltage range of 0.7–1.7 V. Scanning electron microscopy (SEM, TESCAN MIRA4) was used to observe the morphological characteristics of the Zn foil anode.
Synthetic procedures
Synthesis of compound 1. To a 500 mL dry three-neck bottle flask, anhydrous THF (100 mL) and NaH (6 g, 150 mmol) were added under nitrogen at 0 °C, and then triethylene glycol monomethyl ether (16.4 g, 100 mmol) in anhydrous THF (50 mL) was added dropwise. After stirring for 1 h, the mixture was placed in ice bath and propargyl bromide (14.2 g, 120 mmol) in anhydrous THF (50 mL) was added slowly, and then warmed to room temperature and stirred for 24 h. Subsequently, the mixture was dropped into 100 mL of ice water and extracted with ethyl acetate, then washed with saturated brine, and the organic phase was dried over anhydrous MgSO4 and filtered. After removal of the solvent, the crude product was purified via column chromatography (petroleum ether: ethyl acetate = 4
:
1) to yield compound 1 as a light-yellow oil (18 g, yield 89.11%).
Synthesis of compound 2. To a 500 mL dry three-neck bottle flask, triethylene glycol monomethyl ether (24.6 g, 150 mmol) and triethylamine (34.6 mL, 250 mmol) were added at 0 °C, and then p-toluene sulfonyl chloride (23.9 g, 125 mmol) dissolved in dichloromethane (50 mL) was added dropwise and warmed to room temperature. After stirring for 24 h, the mixture was adjusted to acidic with dilute hydrochloric acid, and then washed with deionized water, saturated brine, and extracted with dichloromethane. The organic phase was dried over anhydrous MgSO4. After removal of the solvent, the crude product was obtained (37 g) and used without further purification.The crude product (37 g), acetonitrile (100 mL) and NaN3 (6.11 g, 116 mmol) were added to a 500 mL flask and stirred at 90 °C for 7 d. The reaction solution was filtered and concentrated, and then diluted with ethyl acetate (200 mL) and washed with saturated brine. The organic phase was collected and dried over anhydrous MgSO4. After removal of the solvent, the crude product was purified via column chromatography (petroleum ether: ethyl acetate = 4
:
1) to yield compound 2 as a light-yellow oil (15.2 g, 70% yield).
Synthesis of compound 3. To a solution of compound 1 (9 g, 45 mmol) and compound 2 (7.56 g, 40 mmol) in methanol (50 mL), CuSO4·5H2O (0.5 g, 2 mmol) in deionized water (0.2 mL) was added. After stirring for 30 min under nitrogen, the newly prepared sodium ascorbate solution (Vc: 0.66 g; NaHCO3: 0.33 g) was added and stirred for 24 h at room temperature. Na2S·9H2O (0.48 g, 2 mmol) was added, and after filtration, the filtrate was concentrated, diluted with dichloromethane, and dried over anhydrous MgSO4. After removal of the solvent, the crude product was purified via column chromatography (ethyl acetate: methanol = 10
:
1) to yield compound 3 as a pale-yellow oil (8.3 g, yield 70.9%).
Synthesis of compound 4. Compound 3 (5 g, 13 mmol), CH3I (2.49 mL, 40 mmol) and dichloromethane (5 mL) were added to a 50 mL reaction flask and stirred for 48 h at 45 °C until no compound 3 was detected via thin layer chromatography (TLC). The mixture was concentrated and dried under vacuum to generate compound 4 as a brown liquid (5 g, yield 73.5%).
Synthesis of T1. Compounds 4 (5 g, 10 mmol), methanol (10 mL), LiTFSI (5.7 g, 20 mmol) and deionized water (8 mL) were added to a 100 mL reaction flask and stirred at 70 °C for 3 days. The mixture was extracted with dichloromethane and washed with deionized water until the absence of AgI precipitation in the aqueous phase after the addition of AgNO3 solution. The solution was concentrated and dried under vacuum to obtain T1 as a brown oil (5.1 g, yield 86.9%).
Synthesis of compound 6. To a solution of compound 5 (4.7 g, 25 mmol) and compound 2 (5.7 g, 30 mmol) in methanol (80 mL), CuSO4·5H2O (0.313 g, 1.25 mmol) in deionized water (0.2 mL) was added. After stirring for 30 min under nitrogen, the newly prepared sodium ascorbate solution (Vc: 0.4 g; NaHCO3: 0.2 g) was added and stirred for 24 h at room temperature. Na2S·9H2O (0.3 g, 0.125 mmol) was added, and after filtration, the filtrate was concentrated, diluted with dichloromethane, and dried over anhydrous MgSO4. After removal of the solvent, the crude product was purified via column chromatography (dichloromethane: methanol = 1
:
1) to yield compound 6 as a light-yellow oil (10 g, yield 71.4%).
Synthesis of compound 7. Compound 6 (6.4 g, 17 mmol), CH3I (3.2 mL, 50 mmol) and methyl dichloride (10 mL) were added to a 50 mL reaction flask and stirred for 48 h at 45 °C until no compound 6 was detected via TLC. The mixture was concentrated and dried under vacuum to generate compound 7 as a brown oil (8.1 g, yield 92.1%).
Synthesis of T2. Compounds 7 (8 g, 15 mmol), methanol (20 mL), LiTFSI (9 g, 30 mmol) and deionized water (15 mL) were added to a 100 mL flask stirred at 70 °C for 3 days. The mixture was extracted with dichloromethane and washed with deionized water until the absence of AgI precipitation in the aqueous phase with the addition of AgNO3 solution. The solution was concentrated and dried under vacuum to obtain T2 as a brown oil (6 g, yield 74.1%).
Synthesis of compound 9. To a solution of compound 8 (4.38 g, 25 mmol) and compound 5 (5.64 g, 30 mmol) in methanol (80 mL), CuSO4·5H2O (0.313 g, 1.25 mmol) in deionized water (0.2 mL) was added. After stirring for 30 min under nitrogen, the newly prepared sodium ascorbate solution (Vc: 0.4 g; NaHCO3: 0.2 g) was added and stirred for 24 h at room temperature. Na2S·9H2O (0.3 g, 1.25 mmol) was added, and after filtration, the filtrate was concentrated, diluted with dichloromethane, and dried over anhydrous MgSO4. After removal of the solvent, the crude product was purified using column chromatography (dichloromethane: methanol = 1
:
1) to yield compound 6 as a light-yellow oil (7 g, 77.1%).
Synthesis of compound 10. Compound 9 (2.68 g, 8 mmol), CH3I (2.84 mL, 20 mmol) and methyl dichloride (10 mL) were added to a 50 mL reaction flask and stirred for 48 h at 45 °C until no compound 10 was detected via TLC. The mixture was concentrated and dried under vacuum to generate compound 10 as a brown liquid (3.5 g, yield 87.5%).
Synthesis of T3. Compound 10 (3 g, 6 mmol), methanol (10 mL), LiTFSI (3.4 g, 12 mmol) and deionized water (8 mL) were added to a 100 mL reaction flask and stirred at 70 °C for 3 days. The mixture was extracted with dichloromethane and washed with deionized water until the absence of AgI precipitation in the aqueous phase with the addition of AgNO3 solution. The solution was concentrated and dried under vacuum to obtain T3 as a brown oil (3 g, yield 89.6%).
Results and discussion
Synthesis and characterization of ILs
As shown in Scheme 1, triazoles 3, 6, and 9 with and without –OH groups were synthesized via Cu(I)-catalysed click chemistry using organic azides and alkynes, followed by alkylated and anion metathesis reaction, generating the target triazoliums ILs T1, T2 and T3, and their NMR spectra can be seen in Fig. S1–S12.† The peaks in their 1H NMR spectra at 7.77 ppm (Fig. S3†), 7.85 ppm (Fig. S7†) and 7.91 ppm (Fig. S10†) demonstrate the formation of triazole groups, respectively. After the quaternization of the triazole groups with CH3I, the corresponding peaks shifted to 9.02 ppm (Fig. S4†), 9.03 ppm (Fig. S8†) and 9.18 ppm (Fig. S11†), respectively. Besides, the new peaks at 4.35 ppm (Fig. S4†), 4.38 ppm (Fig. S8†) and 4.40 ppm (Fig. S11†) are ascribed to the methyl protons attached to the N atom on the triazolium ring, as depicted in Scheme 1, which is in agreement with the literatures.34,35 Furthermore, the peak area ratio of the triazolium protons to the methyl protons connected to the triazolium ring is 3
:
1. Thus, all the above-mentioned results confirm the successful quaternization. Usually, ILs with the TFSI− anion possess higher electrochemical stability and weaker interaction intensity with cations, which is beneficial for electrochemical applications.36 Subsequently, the I− anion was exchanged with the TFSI− anion, and the chemical shift of the triazolium protons shifted high-field relative to the corresponding triazolium iodide ILs (Fig. S5, S9 and S12,† respectively), showing that the anion exchange reactions proceeded completely. Additionally, the 13C NMR spectra of the ILs (123–115 ppm, Fig. S5, S9 and S12,† respectively) indicate the successful introduction of the TFSI− anion.
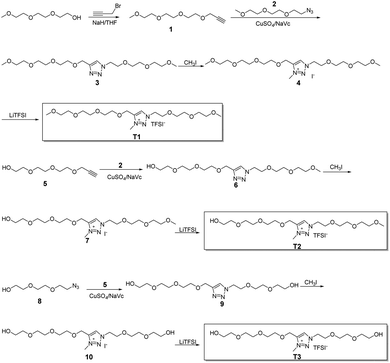 |
| Scheme 1 Synthesis of triazolium ILs T1, T2 and T3. | |
Thermal properties
The thermal stability of ILs determines whether they be applied in high-temperature batteries, and thus the decomposition temperatures of the ILs were measured via thermogravimetric analysis (TGA). As shown in Fig. 1(a), T1 without –OH groups displayed the highest thermal decomposition temperature (Td, 5% weight loss) of 301 °C, whereas T2 and T3 displayed a lower Tds than that of T1, indicating that the presence of –OH groups lowered the thermal stability of the ILs. This because –OH groups possess stronger polarity than that of –OCH3, and hence display a lower activation energy for degradation during heating.37,38 Furthermore, a low glass transition temperature (Tg) is beneficial for the rapid transportation of ions to some extent, especially in polyelectrolytes, and hence the Tgs of the ILs was measured via differential scanning calorimetry (DSC). Fig. 1(b) shows the DSC curves of T1, T2 and T3. Similarly, T1 displayed the lowest Tg of −59 °C, which is still was higher than that of imidazolium-based ILs with alkyl chains.39 The presence of –OH and –OCH2– groups results in increased polarity and viscosity in ILs, which is not conducive to the movement of the segments in ILs, leading to a higher Tg.40
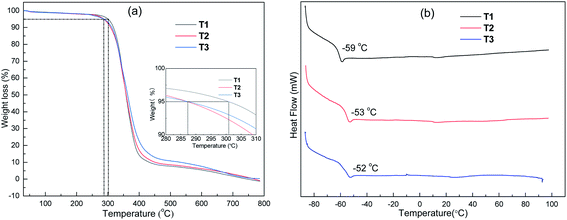 |
| Fig. 1 (a) TGA and (b) DSC curves of T1, T2 and T3. | |
Ionic conductivity
The intrinsic conductivity of the ILs at different temperatures was investigated via electrochemical impedance spectroscopy (EIS) and the results are presented in Table 1, Fig. S13 and S14,† where T1 presented the highest σ value at each temperature. For instance, at 30 °C, the conductivity of T1 (0.88 × 10−3 S cm−1) is about 3-times higher than that of T3 (0.28 × 10−3 S cm−1) and 1.5-times higher than that of T2 (0.57 × 10−3 S cm−1). The conductivity decreased with an increase in the number of terminal –OH groups also because the polarity of the –OH group is not conducive to ion transport. The above-mentioned DSC results also demonstrated this trend, that is, T1 without –OH groups displayed the lowest Tg, and hence beneficial for ion migration and enhancement in conductivity.41 Furthermore, an increase in temperature also improved the conductivity,22 where the highest σ value of T1 was 2.65 × 10−3 S cm−1 at 110 °C.
Table 1 Physicochemical properties of T1, T2 and T3
ILs |
σa/×10−3 S cm−1 |
Tg b/°C |
Td5% c/°C |
ESW d/V |
30 °C |
60 °C |
90 °C |
110 °C |
Determined by EIS. Determined by DSC. Determined by TGA. Determined by LSV. |
T1 |
0.88 |
1.71 |
2.37 |
2.65 |
−59 |
301 |
4.76 |
T2 |
0.57 |
1.46 |
2.23 |
2.55 |
−53 |
286 |
4.11 |
T3 |
0.28 |
1.14 |
1.98 |
2.39 |
−52 |
286 |
3.52 |
The above-mentioned results illustrate that T1 possesses the best performance among the ILs, but its conductivity still need to be improved. An increase in ionic conductivity depends on efficient ion transport, including the number of ions, which is determined by the portion of salts added. Another factor is the mobility of ions, which depends on the ability of the molecules to move. More importantly, researchers have proposed the use of high concentration zinc salt and lithium salt electrolyte (usually 1 M Zn2+ and 20 M Li+) to overcome the shortcomings of conventional aqueous ZIBs,42 such as low Coulomb efficiency, hydrogen/oxygen evolution reaction, and dendrite growth during charge/discharge procedure. Herein, the IL-based electrolytes with 1 M Zn(CF3SO3)2 and different amounts of LiTFSI were prepared by using T1 as the solvent, which were denoted as T1S-x (x = nLi: nZn = 5, 10, 15, 20, 21, and 25). The conductivity of T1S-x was calculated based on the measured Nyquist diagram, as shown in Fig. S15.† The conductivity increased with an increase in the amount of LiTFSI when x is less than 20 due to the increase in carrier LiTFSI salt. The maximum conductivity of 1.55 × 10−3 S cm−1 was realized when x was 20. Upon the further addition of LiTFSI, the conductivity decreased due to the increase in viscosity.43 In comparison, an aqueous electrolyte with the concentration of 1 M Zn(CF3SO3)2 + 20 M LiTFSI was prepared, which was denoted as WS-20, and its conductivity was determined to be 2.74 × 10−3 S cm−1 (calculated using the Nyquist diagram, Fig. S16†), which is higher than that of T1S-20. The conductivity of the two types of electrolytes reached the magnitude of 10−3 S cm−1, and thus they could be used in ZIBs.
Electrochemical properties
Linear sweep voltammetry (LSV) was applied to investigate the electrochemical stability of the ILs by sandwiching them between a freshly polished stainless sheet and zinc sheet, and the results are shown in Fig. 2(a) and Table 1. Among them, T1 displayed the widest ESW (4.76 V) with a cathodic limiting voltage of −1.19 V and anodic limiting voltage of +3.57 V, which is higher than that of imidazolium-based ILs with the TFSI− anion.36,44 Due to the relatively poor stability of the –OH group, T2 and T3 showed narrower ESWs of 4.11 and 3.52 V, respectively. A similar phenomenon was reported for PEO-based solid polyelectrolyte with and without terminal –OH groups.45 As reported previously, the addition of salt can reduce the “free” solvent in the system and decrease the reactivity of the solvent, thus improving the electrochemical stability.46–48 Herein, the addition of salts resulted in a broader ESW of 6.36 V for T1S-20 and 4.46 V for WS-20 (Fig. 2(b)).
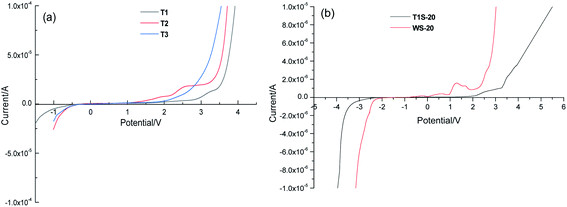 |
| Fig. 2 Linear sweep voltammetry curves of (a) T1, T2, T3 and (b) T1S-20 and WS-20 at 30 °C. | |
To evaluate the application of the ILs in ZIBs, Zn/Li3V2(PO4)3 batteries were prepared using T1S-20 and WS-20 as the electrolyte. Firstly, the cyclic voltammetry (CV) curves were tested for three cycles at a scanning rate of 0.1 mV S−1 at 30 °C. It can be seen in Fig. 3(a) that the T1S-20 system showed two pairs of redox peaks at 1.39/1.47 V and 1.32/1.41 V, which are ascribed to the stepwise Li+ extraction/insertion from/into the Li3V2(PO4)3 structure, respectively,30,49 which exhibit a good degree of coincidence. In contrast, the WS-20 system displayed a relatively large peak potential deviation (Fig. 3(b)), which may be due to polarization.50
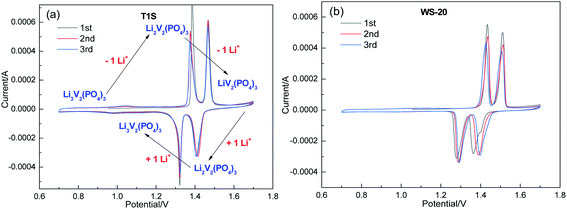 |
| Fig. 3 CV curves of Zn/Li3V2(PO4)3 batteries with (a) T1S-20 and (b) WS-20 electrolyte at a scanning rate of 0.1 mV s−1. | |
Charge/discharge performance
Fig. 4(a) shows the rate performance of T1S-x and WS-20 in the Zn/Li3V2(PO4)3 batteries at current densities of 0.2, 0.3, 0.5, 1.0 and 1.5 A g−1. With an increase in the content of LITFSI added, the T1S-x system showed an improved initial capacity, and the battery with T1S-20 delivered the highest initial capacity of 80 mA h g−1, which is better than that of aqueous electrolyte-based Zn/Li3V2(PO4)3 batteries.30 In comparison, the initial capacity of the WS-20 system was 76 mA h g−1, and the discharge capacity of the T1S-20 system was higher than that of WS-20 system at each rate. After adjusting the current density back to 0.2 A g−1, the capacity of the T1S-20 and WS-20 systems was 79 and 67 mA h g−1, respectively. With an increase in temperature to 80 °C, the capacities of both systems increased, where the WS-20 system displayed a relatively large capacity attenuation after 30 cycles (Fig. 4(b)), while the T1S-20 system could almost recover its initial capacity (111 mA h g−1). Hence, the T1S-20 system displayed a better rate performance in ZIBs.
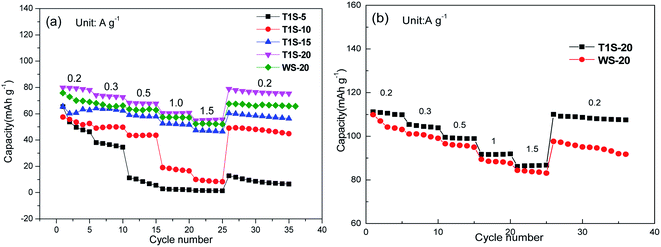 |
| Fig. 4 Rate performance of Zn/Li3V2(PO4)3 battery with T1S-x and WS-20 electrolyte at different rates at (a) 30 °C and (b) 80 °C. | |
Fig. 5 and 6 show the cyclic performance and Coulomb efficiency of the Zn/Li3V2(PO4)3 batteries based on the T1S-20 and WS-20 electrolytes at 30 °C and 80 °C. As shown in Fig. 5(a), the initial discharge capacity of the T1S-20 and WS-20 systems was 81 and 78 mA h g−1 at 30 °C, and after 50 cycles, the capacity retention rate of the T1S-20 system was 89%, which is higher than that of the WS-20 system (75%). Their initial discharge capacity and capacity retention rate are superior to that of imidazolium-IL-based Zn/polymer batteries, but much lower than that of the Zn/cobalt ferricyanide battery,51–53 and hence the cathode materials also play a crucial role in the battery performance, as also concluded from our previous investigation.30 In addition, the relatively low conductivity of the electrolytes was the main reason for the poor battery performances. At 80 °C, the initial discharge capacity of the two systems increased to 111 mA h g−1, which is comparable to that of a ZIB with a Zn(OTf)2-containing solid polymer electrolyte54 and higher than that of the Zn/MnO2 battery at high temperature.55 After 50 cycles, their discharge capacities decreased to 108 mA h g−1 (for T1S-20) and 100 mA h g−1 (for WS-20), together with the capacity retention rate of 98.2% and 90.9% (Fig. 5(b)), respectively. After 100 cycles, the capacity of the WS-20 system was 79 mA h g−1 with a capacity retention rate of 71.8%, which is much lower than that of the T1S-20 system (103.9 mA h g−1, 93.6%). Hence, the T1S-20 system has better cyclic stability than the WS-20 system. As mentioned above, the electrode materials affect the performances of ZIBs, where dissolution of the cathode materials will reduce the utilization of the active substances56 and side reactions will decrease the cycle stability and irreversibility. In the WS-20 system, the presence of water would cause the dissolution of the cathode material and the formation of (Zn(H2O)6)2+,57 as well as dendrites. On the contrary, in the T1S-20 system, its wide ESW, high thermal stability and absence of water guaranteed the stability of the battery.48,58 Also, no molecules decomposed during the charge/discharge procedure to interact with vanadium and cause its dissolution.56 Furthermore, no (Zn(H2O)6)2+ was formed in the T1S-20 system, which may generate a irreversibility issue in the Zn anode, and hence the T1S-20 system-based ZIB displayed a better performance. Besides, it can be seen from Fig. 6(a) and (b) that the ZIBs exhibited a good charge and discharge efficiency with a Coulomb efficiency of 99%. With an increase in the temperature to 80 °C, the Coulomb efficiency of the T1S-20 system remained at 99% (Fig. 6(c)), but that of the WS-20 system displayed a slight decrease (97%) (Fig. 6(d)).
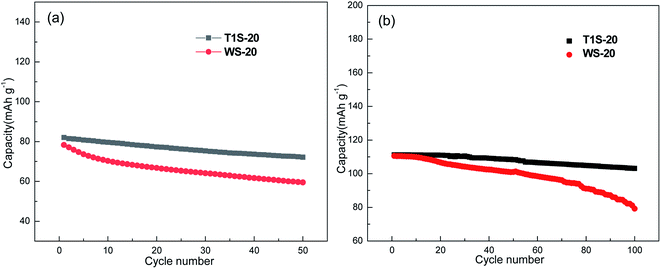 |
| Fig. 5 Cyclic performance of Zn/Li3V2(PO4)3 batteries with T1S-20 and WS-20 electrolyte at (a) 30 °C and (b) 80 °C at a current density of 0.2 A g−1. | |
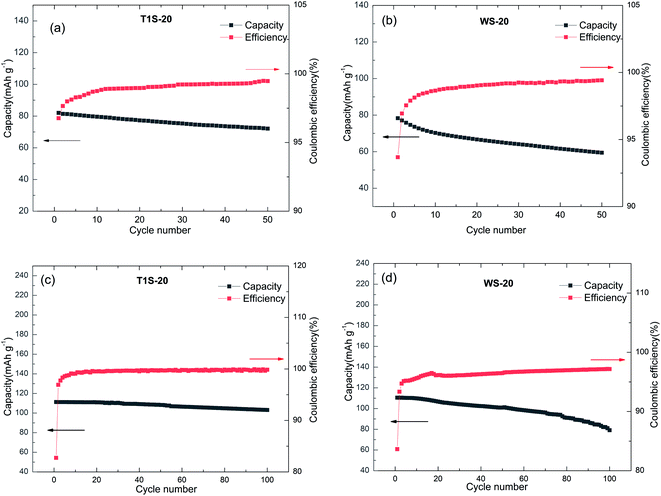 |
| Fig. 6 Coulomb efficiency of Zn/Li3V2(PO4)3 batteries with different electrolytes at 0.2 A g−1. (a) T1S-20 at 30 °C, (b) WS-20 at 30 °C, (c) T1S-20 at 80 °C and (d) WS-20 at 80 °C. | |
The constant current charge/discharge tests of T1S-20 in the Zn/Li3V2(PO4)3 battery were carried out in the voltage range of 0.7–1.7 V at a current density of 0.2 A g−1 at 30 °C and 80 °C, respectively. As shown in Fig. 7(a), two platforms located at about 1.39 and 1.48 V can be observed during the charging process. The first platform (1.39 V) is ascribed to the first Li+ ion extraction from Li3V2(PO4)3, resulting in the oxidation of V3+ to V4+. The second platform (1.48 V) corresponds to the extraction of a second Li+ ion with the transformation of Li2V2(PO4)3 to LiV2(PO4)3. The discharge platforms are located at about 1.43 and 1.33 V, respectively, corresponding to the insertion of two Li+ ions in the LiV2(PO4)3 lattice with the reduction of V4+ to V3+.30,49 At 80 °C, similar platforms could be observed (Fig. 7(b)).
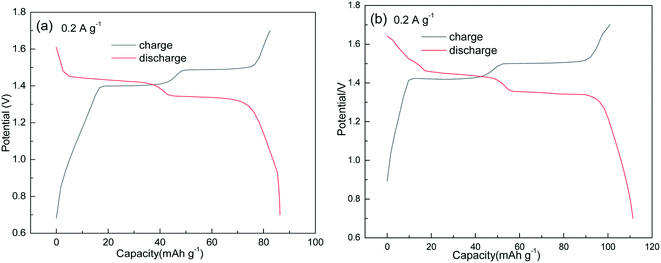 |
| Fig. 7 First charge/discharge curves of Zn/Li3V2(PO4)3 batteries with T1S-20 electrolyte at (a) 30 °C and (b) 80 °C. | |
Fig. 8 depicts the Zn foil anode in the ZIBs after 50 cycles with the WS-20 and T1S-20 electrolytes at 30 °C, verifying the superiority of the ILs. After the batteries functioned for 50 cycles, the zinc foil anode and separator were taken out and washed with anhydrous tetrahydrofuran carefully three times, and then ethanol and deionized water in succession. As shown in Fig. 8(a), the dark separator indicates the dissolution of the cathode material in WS-20, and the lumps in the SEM image (Fig. 8(c)) confirm this. By contrast, the separator in Fig. 8(b) was very clean, and no obvious dissolution of the cathode material was observed.
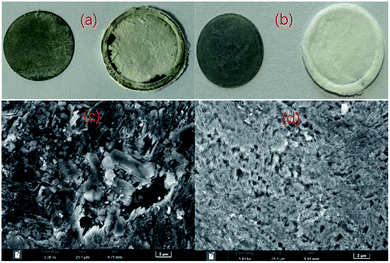 |
| Fig. 8 Digital pictures and SEM images of Zn foil anode of Zn/Li3V2(PO4)3 batteries after 50 cycles with (a and c) WS-20 and (b and d) T1S-20 electrolyte at 30 °C, respectively. | |
Subsequently, the high-temperature performance of the ZIBs was investigated. As shown in Fig. 9(a), after undergoing 100 cycles at 80 °C, the ZIB with the WS-20 electrolyte became inflated, which restricts its application at high temperature, whereas the ZIB with the T1S-20 electrolyte retained its initial shape (Fig. 9(b)). Similarly, the SEM images of their Zn foil anodes were measured. The Zn foil anode of the WS-20 electrolyte-based ZIB displayed obvious dendrites and cathode dissolution (Fig. 9(c)), which is due to the accelerated water-induced side reaction, and a similar phenomenon was observed in aqueous ZIBs.59 On the contrary, no obvious dendrites and cathode dissolution could be observed in the T1S-20 electrolyte-based ZIB under the same condition (Fig. 9(d)). Therefore, the T1S-20 electrolyte effectively alleviated the dissolution of the cathode material and displayed a good high-temperature performance.
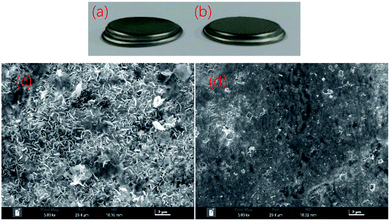 |
| Fig. 9 Digital pictures and SEM images of Zn foil anode of Zn/Li3V2(PO4)3 batteries after 100 cycles with (a and c) WS-20 and (b and d) T1S-20 electrolyte at 80 °C. | |
Conclusions
Triazolium-based ILs T1, T2 and T3 with or without hydroxyl groups were prepared and T1 without hydroxyl groups showed the best performance. By doping with Zn(CF3SO3)2 and LiTFSI, the conductivity and ESW of T1S-20 at 30 °C were 1.55 × 10−3 S cm−1 and 6.36 V, respectively. Based on this, Zn/Li3V2(PO4)3 ZIBs were assembled with T1S-20 electrolyte and their cycle stability and rate performance were investigated at 30 °C and 80 °C. Simultaneously, aqueous ZIBs with the addition of the same salts (WS-20) were investigated for comparison. The T1S-20-based ZIB displayed a better performance, where after 50 cycles, its capacity retention rate was 89% at 30 °C, which was higher than that of the WS-20-based ZIB (75%). With an increase in the temperature to 80 °C, the superiority of the T1S-20-based ZIB was much more obvious, displaying a Coulomb efficiency of 99%. In addition, there were no obvious dendrites and cathode material dissolution in the T1S-20-based ZIB. Thus, all the results demonstrated that triazolium-based ILs can be used in high-temperature ZIBs, and triazolium-based ILs with different structures are currently under investigation to further improve their performance.
Conflicts of interest
There are no conflicts to declare.
Acknowledgements
The authors thank the National Natural Science Foundation of China (No. 21764006, 51862008, 52064014), Natural Science Foundation of Hunan Province, China (No. 2020JJ4505, 2017JJ3256), the Educational Commission of Hunan Province, China (No. 17B213, 202110531024), the Research Foundation of Jishou University of Hunan Province, China (No. Jdy20032) for the financial supports of this work.
Notes and references
- C. Xu, B. Li, H. Du and F. Kang, Angew. Chem., Int. Ed., 2012, 51, 933–935 CrossRef CAS PubMed.
- Y. Zhao, Y. Zhu and X. Zhang, InfoMat, 2019, 2, 237–260 CrossRef.
- H. Pan, Y. Shao, P. Yan, Y. Cheng, K. S. Han, Z. Nie, C. Wang, J. Yang, X. Li, P. Bhattacharya, K. T. Mueller and J. Liu, Nat. Energy, 2016, 1, 16039 CrossRef CAS.
- L. Suo, O. Borodin, T. Gao, M. Olguin, J. Ho, X. Fan, C. Luo, C. Wang and K. Xu, Science, 2015, 350, 938–943 CrossRef CAS PubMed.
- J. Yan, J. Wang, H. Liu, Z. Bakenov, D. Gosselink and P. Chen, J. Power Sources, 2012, 216, 222–226 CrossRef CAS.
- S. Bai, X. Li, Y. Wen, J. Cheng, G. Cao, Y. Yang and D. Li, Acta Phys. Sin-Ch. Ed., 2016, 32, 2007–2017 CAS.
- S. Wu, Y. Wang, W. Liu, M. Ren, F. Kong, S. Wang, X. Wang, H. Zhao and J. Bao, Inorg. Chem. Front., 2018, 5, 3067–3073 RSC.
- F. Tang, J. Gao, Q. Ruan, X. Wu, X. Wu, T. Zhang, Z. Liu, Y. Xiang, Z. He and X. Wu, Electrochim. Acta, 2020, 353, 136570 CrossRef CAS.
- F. Tang, T. He, H. Zhang, X. Wu, Y. Li, F. Long, Y. Xiang, L. Zhu, J. Wu and X. Wu, J. Electroanal. Chem., 2020, 873, 114368 CrossRef CAS.
- X. Xie, S. Liang, J. Gao, S. Guo, J. Guo, C. Wang, G. Xu, X. Wu, G. Chen and J. Zhou, Energy Environ. Sci., 2020, 13, 503–510 RSC.
- M. Song, H. Tan, D. Chao and H. J. Fan, Adv. Funct. Mater., 2018, 28, 1802564 CrossRef.
- X. Song, H. He, M. Aboonasr Shiraz, H. Zhu, A. Khosrozadeh and J. Liu, Chem. Commun., 2021, 57, 1246–1249 RSC.
- A. Wang, W. Zhou, A. Huang, M. Chen, Q. Tian and J. Chen, J. Colloid Interface Sci., 2021, 586, 362–370 CrossRef CAS PubMed.
- R. Feng, X. Chi, Q. Qiu, J. Wu, J. Huang, J. Liu and Y. Liu, ACS Appl. Mater. Interfaces, 2021, 13, 40638–40647 CrossRef PubMed.
- M. Becker, D. Rentsch, D. Reber, A. Aribia, C. Battaglia and R. S. Kuhnel, Angew. Chem., Int. Ed., 2021, 60, 14100–14108 CrossRef CAS PubMed.
- N. Zhang, F. Cheng, Y. Liu, Q. Zhao, K. Lei, C. Chen, X. Liu and J. Chen, J. Am. Chem. Soc., 2016, 138, 12894–12901 CrossRef CAS PubMed.
- N. Zhang, F. Cheng, J. Liu, L. Wang, X. Long, X. Liu, F. Li and J. Chen, Nat. Commun., 2017, 8, 405 CrossRef PubMed.
- Y. Ye and Y. A. Elabd, Macromolecules, 2011, 44, 8494–8503 CrossRef CAS.
- N. Chen, Y. Guan, J. Shen, C. Guo, W. Qu, Y. Li, F. Wu and R. Chen, ACS Appl. Mater. Interfaces, 2019, 11, 12154–12160 CrossRef CAS PubMed.
- L. Liu, S. He, S. Zhang, M. Zhang, M. D. Guiver and N. Li, ACS Appl. Mater. Interfaces, 2016, 8, 4651–4660 CrossRef CAS PubMed.
- Y. Jin, S. Fang, M. Chai, L. Yang and S. Hirano, Ind. Eng. Chem. Res., 2012, 51, 11011–11020 CrossRef CAS.
- J. Wu, J. Chen, J. Wang, X. Liao, M. Xie and R. Sun, Polym. Chem., 2016, 7, 633–642 RSC.
- J. Wu, C. Wang, D. Zhou, X. Liao, M. Xie and R. Sun, Macromol. Rapid Commun., 2016, 37, 2017–2022 CrossRef CAS PubMed.
- D. Flachard, A. Serghei, M. Fumagalli and E. Drockenmuller, Polym. Int., 2019, 68, 1591–1598 CrossRef CAS.
- W. Deng, Z. Zhou, Y. Li, M. Zhang, X. Yuan, J. Hu, Z. Li, C. Li and R. Li, ACS Nano, 2020, 14, 15776–15785 CrossRef PubMed.
- J. Liu, N. Nie, J. Wang, M. Hu, J. Zhang, M. Li and Y. Huang, Mater. Today Energy, 2020, 16, 100372 CrossRef.
- X. Li, H. Wang, X. Sun, J. Li and Y. Liu, ACS Appl. Energy Mater., 2021, 4, 12718–12727 CrossRef CAS.
- K. Yoshii, T. Uto, N. Tachikawa and Y. Katayama, Phys. Chem. Chem. Phys., 2020, 22, 19480–19491 RSC.
- S. Brutti, E. Simonetti, M. De Francesco, A. Sarra, A. Paolone, O. Palumbo, S. Fantini, R. Lin, A. Falgayrat, H. Choi, M. Kuenzel, S. Passerini and G. B. Appetecchi, J. Power Sources, 2020, 479, 228791 CrossRef CAS.
- Y. Jiang, Q. Zou, S. Liu, H. Zeng, L. Chen, Y. Xiang, J. Li, X. Wu, J. Wu and L. Xiong, J. Electroanal. Chem., 2021, 900, 115685 CrossRef CAS.
- H. Li, C. Wang, X. Liao, M. Xie and R. Sun, Polymer, 2017, 112, 297–305 CrossRef CAS.
- D. Basak, S. Christensen, S. K. Surampudi, C. Versek, D. T. Toscano, M. T. Tuominen, R. C. Hayward and D. Venkataraman, Chem. Commun., 2011, 47, 5566–5568 RSC.
- H. Li, J. Wang, H. Han, J. Wu and M. Xie, React. Funct. Polym., 2018, 127, 20–28 CrossRef CAS.
- A. Jourdain, A. Serghei and E. Drockenmuller, ACS Macro Lett., 2016, 5, 1283–1286 CrossRef CAS.
- M. M. Obadia, A. Jourdain, P. Cassagnau, D. Montarnal and E. Drockenmuller, Adv. Funct. Mater., 2017, 27, 1703258 CrossRef.
- Z. Liu, T. Cui, T. Lu, M. Shapouri Ghazvini and F. Endres, J. Phys. Chem. C, 2016, 120, 20224–20231 CrossRef CAS.
- Z. Xue, Y. Zhang, X. Zhou, Y. Cao and T. Mu, Thermochim. Acta, 2014, 578, 59–67 CrossRef CAS.
- J. Vuković, D. Steinmeier, M. D. Lechner, S. Jovanović and B. Božić, Polym. Degrad. Stab., 2006, 91, 1903–1908 CrossRef.
- E. P. Yambou, B. Gorska and F. Béguin, J. Mol. Liq., 2020, 298, 111959 CrossRef CAS.
- C. Sirisopanaporn, A. Fernicola and B. Scrosati, J. Power Sources, 2009, 186, 490–495 CrossRef CAS.
- M. Verma and H. Sahu, Ionics, 2015, 21, 3223–3231 CrossRef CAS.
- F. Wang, O. Borodin, T. Gao, X. Fan, W. Sun, F. Han, A. Faraone, J. A. Dura, K. Xu and C. Wang, Nat. Mater., 2018, 17, 543–549 CrossRef CAS PubMed.
- X. Hu, R. Muchakayala, S. Song, J. Wang, J. Chen and M. Tan, Int. J. Hydrogen Energy, 2018, 43, 3741–3749 CrossRef CAS.
- Z. Liu, T. Cui, G. Li and F. Endres, Langmuir, 2017, 33, 9539–9547 CrossRef CAS PubMed.
- X. Yang, M. Jiang, X. Gao, D. Bao, Q. Sun, N. Holmes, H. Duan, S. Mukherjee, K. Adair, C. Zhao, J. Liang, W. Li, J. Li, Y. Liu, H. Huang, L. Zhang, S. Lu, Q. Lu, R. Li, C. V. Singh and X. Sun, Energy Environ. Sci., 2020, 13, 1318–1325 RSC.
- M. Chen, J. Wu, T. Ye, J. Ye, C. Zhao, S. Bi, J. Yan, B. Mao and G. Feng, Nat. Commun., 2020, 11, 5809 CrossRef CAS PubMed.
- O. Borodin, Curr. Opin. Electrochem., 2019, 13, 86–93 CrossRef CAS.
- Z. Fang, Y. Luo, H. Liu, Z. Hong, H. Wu, F. Zhao, P. Liu, Q. Li, S. Fan, W. Duan and J. Wang, Adv. Sci., 2021, 8, 2100736 CrossRef CAS PubMed.
- C. Li, W. Yuan, C. Li, H. Wang, L. Wang, Y. Liu and N. Zhang, Chem. Commun., 2021, 57, 4319–4322 RSC.
- D. Cheng, Y. F. Yang, J. L. Xie, C. J. Fang, G. Q. Zhang and J. Xiong, J. Mater. Chem. A, 2015, 3, 14348–14357 RSC.
- T. J. Simons, M. Salsamendi, P. C. Howlett, M. Forsyth, D. R. MacFarlane and C. Pozo-Gonzalo, ChemElectroChem, 2015, 2, 2071–2078 CrossRef CAS.
- Z. Liu, A. Prowald, O. Höfft, G. Li, A. Lahiri and F. Endres, ChemElectroChem, 2018, 5, 2321–2325 CrossRef CAS.
- L. Ma, S. Chen, N. Li, Z. Liu, Z. Tang, J. A. Zapien, S. Chen, J. Fan and C. Zhi, Adv. Mater., 2020, 32, 1908121 CrossRef CAS PubMed.
- Z. Chen, X. L. Li, D. H. Wang, Q. Yang, L. T. Ma, Z. D. Huang, G. J. Liang, A. Chen, Y. Guo, B. B. Dong, X. Y. Huang, C. Yang and C. Y. Zhi, Energy Environ. Sci., 2021, 14, 3492–3501 RSC.
- F. N. A. Mo, H. F. Li, Z. X. Pei, G. J. Liang, L. T. Ma, Q. Yang, D. H. Wang, Y. Huang and C. Y. Zhi, Sci. Bull., 2018, 63, 1077–1086 CrossRef CAS.
- H. N. Si, L. Li, W. J. Hao, L. Seidl, X. L. Cheng, H. Y. Xu, G. X. Jia, O. Schneider, S. L. An and X. P. Qiu, ACS Appl. Energy. Mater., 2019, 2, 5050–5056 CrossRef CAS.
- C. Liu, X. Xie, B. Lu, J. Zhou and S. Liang, ACS Energy Lett., 2021, 6, 1015–1033 CrossRef CAS.
- X. C. Wang, Z. F. Shang, A. K. Yang, Q. Zhang, F. Y. Cheng, D. Z. Jia and J. Chen, Chem, 2019, 5, 364–375 CAS.
- J. Huang, X. Chi, Y. Du, Q. Qiu and Y. Liu, ACS Appl. Mater. Interfaces, 2021, 13, 4008–4016 CrossRef CAS PubMed.
Footnote |
† Electronic supplementary information (ESI) available: 1H NMR and 13C NMR spectra of ILs T1, T2, T3 and corresponding intermediates, Nyquist plots of and conductivities of ILs. See DOI: 10.1039/d2ra00298a |
|
This journal is © The Royal Society of Chemistry 2022 |
Click here to see how this site uses Cookies. View our privacy policy here.