DOI:
10.1039/D2RA00514J
(Paper)
RSC Adv., 2022,
12, 10825-10834
Remediation of PAHs contaminated industrial soils by hypochlorous acid: performance and mechanisms†
Received
25th January 2022
, Accepted 27th March 2022
First published on 7th April 2022
Abstract
Polycyclic aromatic hydrocarbons (PAHs) mainly originate from incomplete combustion of organic substances and are carcinogenic, mutagenic and teratogenetic, posing a high risk to the ecosystem and human health. The remediation of soils contaminated with PAHs has aroused wide public concern. In this study, hypochlorous acid (HOCl) was applied to realize PAHs removal from industrial contaminated soil with an extremely high degradation efficiency of 93.33% when the initial chlorine concentration was 5000 mg L−1. The degradation behavior of PAHs by HOCl oxidation was investigated in detail. Parameters including chlorine dosage, pH and temperature that had effects on the degradation process were evaluated systematically. The removal of PAHs was followed well with the pseudo-first-order kinetic model. It is found that HOCl and OH˙ were major contributors to the degradation products of chlorinated and oxygenated PAHs. This research provided an easy-operating and energy-saving way to realize the remediation of PAHs contaminated industrial soil practically with high efficiency.
1. Introduction
Polycyclic aromatic hydrocarbons (PAHs) are a series of persistent organic pollutants in which more than two benzene rings are linked in a dense ring form.1–3 The main source of these compounds is the incomplete combustion or pyrolysis of fossil fuels, wood and other hydrocarbon containing compounds.4–6 As a consequence of the accelerating industrialization and urbanization, PAHs are ubiquitous contaminants in the atmosphere, water bodies and soils.7 They are carcinogenic, mutagenic and teratogenetic, heavily threatening human health and environmental safety.8–11 Over the past few years, the eliminating of PAHs in the ecosystem has aroused wide public concern.12–14
Due to their hydrophobic nature, low water solubility, high octanol/water partition coefficient (Kow) and high organic carbon/water partition coefficient (Koc) (for example, benzo[a]anthracene has a log
Kow value of 5.82 and a log
Koc value of 5.3), PAHs are easily accumulated in soils by sorption mechanisms.2,15,16 For PAHs polluted soils, especially the industrial contaminated sites with the characteristics of long aging time, high pollutants concentration and complex composition, it is a major challenge to realize soil remediation environmental friendly, cost-effectively and efficiently. Traditional methods like thermal desorption, soil washing and bioremediation are not suitable for the treatment of industrial PAHs-polluted soil because of their defects of high energy consumption, possible secondary pollution and long processing period, respectively.17–20 Among various soil restoration techniques, chemical oxidation has been recognized as an efficient, economical, and easy-to-operate choice to deal with PAHs-polluted soils.
Many efforts have been paid to explore chemical oxidation methods that are effective in the removal of PAHs from contaminated soils.7,21 Various oxidants include Fenton reagents, persulfate, permanganate, and ozone are added into soils directly, converting PAHs into less harmful chemicals. For Fenton process, ferrous ions can react with hydrogen peroxide to produce hydroxyl radicals, which have a high oxidation potential and can oxide most organic matters non-selectively.22,23 However, adjusting the soil pH to 2.8–3.0 is a prerequisite for Fenton process, heavily inhibiting its wide application.24,25 Based on sulfate radical oxidation mechanism, activated persulfate is able to degrade pollutants in soils. According to diverse activation conditions (such as temperature, pH, light, transition metal, base, catalyst, and electrical activation, etc.), persulfate oxidation process will produce different free radicals to participate in the oxidation reaction, which will also have varying degrees of influences on the degradation results.26,27 Owning to its safety in use, stability in performance and environmental friendliness, persulfate oxidation system has been gaining lots of attention in academic research and practical application.28,29 For the time being, however, developing efficient, energy-saving and cost-reducing activation methods still pose major technological hurdles.30 Permanganate is a strong oxidizing agent that has been applied in organic contaminants degradation through a non-radical mechanism.31,32 But its poor disintegration performance towards chlorinated alkanes and most aromatic compounds implies its certain limitation in application.26 Ozone treatment can decompose organic pollutants directly by ozone or indirectly by produced hydroxyl radicals.33–35 The efficiency of ozone oxidation in the removal of pollutants from soil is restricted by soil structure, moisture, pH, and so on. Published articles have investigated that soil with large pore spaces favors ozone transportation.21 Besides, the increase in soil moisture leads to reduction in removal efficiency of pollutants. Consequently, it is necessary to develop an efficient chemical oxidation method that is cost-effective and widely applicable.
Our previous work has studied the oxidation degradation behavior of PAHs in contaminated industrial soil by chlorine dioxide (ClO2).36 It is found that chlorine dioxide is a safe, low cost oxidant, realizing a high PAHs degradation efficiency of 84.24% at a dosage of 0.90 mol kg−1. The mechanisms for PAHs degradation by ClO2 oxidation were proposed to be the direct oxidation by ClO2 and indirect oxidation by HOCl and OH˙. Nevertheless, the preparation of chlorine dioxide by improved sodium chlorite acidification method was sophisticated and had security risk. The gas form of chlorine dioxide also increases the difficulty of application in industrial polluted soil remediation. Inspired by the most commonly used household chlorine bleach, chlorination through adding sodium hypochlorite directly into soil system is a more convenient, more energy-saving and much safer way to realize PAHs removal.
To the best of our knowledge, no published reports have attempted to remove PAHs from contaminated industrial soils using HOCl. In this study, the degradation behavior of PAHs by HOCl oxidation was investigated in detail. Parameters affecting the PAHs degradation behavior including chlorine dosage, pH, and temperature were carefully evaluated, respectively. The kinetics, products, and mechanisms of HOCl oxidation process in PAHs contaminated industrial soil were identified. This study will provide new thoughts into the remediation of industrial polluted soil using reliable, convenient, and cost-reducing HOCl oxidation technique.
2. Materials and methods
2.1 Chemicals and materials
High-performance-liquid-chromatography grade acetone, dichloromethane, n-hexane, and isopropanol (IPA) were acquired from Merck (Darmstadt, Germany). Sodium hydroxide (NaOH, AR), sodium thiosulfate (Na2S2O3, ≥99%), sulfuric acid (H2SO4, AR) and ethanol were bought from National Medicines Corporation Ltd. (Beijing, China). Sodium hypochlorite solution (available Cl2 as 4.00–4.99%), and 9,10-anthraquinone (≥99%) were obtained from Sigma-Aldrich (St. Louis, MO, USA). p-Benzoquinone (PBQ, ≥99%) was purchased from Tokyo Chemical Industry (TCI). tert-Butanol (TBA, ≥99%) was bought from General-reagent (Titan, China). Compounded and simplex PAH standard solutions including pyrene (≥98.4%), phenanthrene (≥98.9%), anthracene (≥99.2%), and fluoranthene (≥99.2%) were bought from AccuStandard (New Haven, CT, USA). All of the solutions here were prepared with ultra-pure water generated from a Milli-Q water purification system (Millipore, USA).
The soil contaminated with polycyclic aromatic hydrocarbons used in the experiment was collected from a coking plant (Shenyang, China). The soil samples used in this study were collected from a chronically PAHs contaminated site at the depth of 1 m below the surface using the cross-line method. In a fume hood, the collected contaminated soil samples were air-dried, ground and sieved with a 60-mesh sieve to achieve the purpose of removing leaves, debris and stones. Then the treated soils were mixed evenly and put into a sealed brown glass bottle for on-demand usage. The physical and chemical properties of the contaminated soil and the concentration of polycyclic aromatic hydrocarbons were listed in Tables S1 and S2,† respectively. The total concentration of PAHs in the contaminated soil was 2469.9 mg kg−1; the concentration of each PAHs was shown in Table S2.†
2.2 PAHs degradation procedures by HOCl oxidation
The degradation process of PAHs in contaminated industrial soil was carried out under continuous stirring at 180 rpm and at 25 °C in a sealed transparent borosilicate glass vial (CNW, Germany) with a volume of 40 mL. First, 0.5003 ± 0.0003 g of the PAHs contaminated soil was added into the vial. And then, 10 mL of sodium hypochlorite solutions (whose dosage was expressed in terms of effective chlorine concentrations) with different effective chlorine concentrations of 1000, 2000, 3000, 4000, and 5000 mg L−1 were added to initiate the reaction. The justification for the selection of the effective chlorine dosages was given in the ESI (Fig. S1†). The PAHs removal test was continued for 48 h to investigate the time/chlorine dosage-dependent behavior. The influence of pH on the degradation of PAHs in soil slurry was studied by adjusting the pH from 4.9 to 10.9 using 1 mM HCl and NaOH solution. The temperature-dependent trend of PAHs degradation was evaluated at 15, 25, and 35 °C, respectively. Soil samples were taken from the constant temperature shaker at certain time intervals to record the residual effective chlorine concentration and pH value of sodium hypochlorite. Excess Na2S2O3 was used to consume the remaining sodium hypochlorite in the samples, and then, the sample slurries were centrifuged at 3500 rpm for 40 min followed by discarding the supernatant. 10 mL of dichloromethane/n-hexane (1
:
1 v/v) solution was applied to extract the centrifuged soil in vortex mixer at 25 °C for 20 min. After that, another centrifugation process at 3500 rpm for 20 min was conducted. In the end, the upper organic solution (10–25 μL) was drawn using a liquid phase micro-injector, and then diluted to 1.0 mL with n-hexane, preparing for the analysis by GC-MS. All these degradation experiments mentioned above were repeated three times, and the average value was regarded as the result. The relative standard deviation (RSD) of the results of three independent experiments was <10%. In addition, the degradation efficiency (η) of PAHs by HOCl oxidation for each sample was calculated according to eqn (1) given below: |
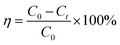 | (1) |
where C0 refers to the initial concentration of PAHs, and Ct refers to the concentration of PAHs in the soil sample at a certain time interval.
2.3 Quenching experiments
To recognize the degradation contributors of the PAHs degradation by HOCl oxidation, IPA, TBA and PBQ were used as scavengers for hydroxyl radicals (OH˙), chlorine radicals (Cl˙) and superoxide radicals (˙O2−), respectively. IPA with concentrations varying from 150 to 400 mM were added prior to the vial with industrial contaminated soil in, and then sodium hypochlorite was poured in to initiate the oxidation reaction. Samples were collected at certain time intervals. Subsequently, PAHs were extracted using 10 mL of n-hexane/dichloromethane (1
:
1 v/v) mixed solution. The quenching experiments using TBA as scavengers were performed in the similar way as IPA. PBQ was dissolved in Milli-Q water and diluted to 17.5, 35 and 70 mM. PBQ with different initial concentrations were added to the reaction system in a similar way before the addition of sodium hypochlorite. The determination of PAHs was followed the method above mentioned.
2.4 Identification of PAHs degradation products
To identify the products of PAHs degradation, the HOCl oxidation experiments were carried out in aqueous solution using anthracene, phenanthrene, pyrene and fluoranthene as representative pollutants. 10 μL of each PAH was taken from its stock solution of 1000 mg L−1, and then added to 10 mL of sodium hypochlorite solution to constitute liquid reaction system with an initial PAH concentration of 1 mg L−1. In order to meet the detection requirements for concentration of GC-MS, 50 parallels were prepared at the same way for each PAH. During the degradation process, samples were collected at certain time intervals, and quenched immediately by excess Na2S2O3. Degradation products of PAH in each parallel experiment was extracted by 5 mL of n-hexane. All these extracted solutions of 50 parallel experiments were collected and mixed together to be concentrated to 5 mL using a rotary evaporator and a pressure blowing concentrator. The remaining water was removed by passing through anhydrous sodium sulfate columns. Then the extracted solutions were concentrated to 0.5 mL by nitrogen purging. Finally, the obtained solution was suitable for GC-MS analysis.
2.5 Analytical methods
Gas chromatography-mass spectrometry (GC-MS) (QP2010, Shimadzu, Japan) was utilized to do qualitative and quantitative analysis for PAHs and their degradation products. A SH-Rxi-5Sil MS capillary column (5% diphenyl/95% dimethyl polysiloxane) from Shimadzu with dimensions of 30.0 m × 0.25 mm × 0.25 μm was used with helium as the carrier gas (1 mL min−1). The inlet temperature, ion source temperature, and MS temperature were set to 280 °C, 230 °C, 300 °C, respectively. In SIM mode, the programmed setting of GC-MS column temperature was as follows: firstly, the column temperature was kept at 60 °C for 1 min, then the column temperature was heated to 200 °C at the rate of 10 °C min−1, and then the column temperature was continuously heated to 300 °C at the rate of 8 °C min−1 after reaching 200 °C, and then the column temperature was kept at 300 °C for 8 min before exhaust purge. In San mode, the GC-MS column temperature was programmed as follows: first, after being kept at 50 °C for 1 min, the column temperature rose from 50 °C to 300 °C at the rate of 5 °C min−1, and then the tail gas was purged after being kept at 300 °C for 5 min. N,N-Diethyl-p-phenylenediamine (DPD) colorimetric method (Hach, PCII 58700-00, USA) was applied to detect the concentration of effective chlorine in the PAHs degradation process. The system pH was monitored by a pH meter (Sartorius, PB-10, Beijing, China) that was often calibrated by standard buffer solutions (pH = 4.01, 7.00, 9.21).
3. Results and discussion
3.1 Effect of pH on PAHs degradation
The pH of the reaction system was an important factor in the degradation of PAHs. The effects of the initial pH were investigated and were presented in Fig. 1a. It could be found that when the pH increased from 4.9 to 10.9, the degradation efficiency of PAHs changed from 93.33% to 71.1%, indicating that increasing the system pH resulted in a substantial reduction in the removal ratio of PAHs. Since PAHs are a series of non-ionizable organic compounds, this definite pH-dependent degradation behavior might be ascribed to the character of chlorine. The dissociation equilibrium of chlorine was shown in eqn (2).37,38 HOCl and OCl− have different reaction rates with PAHs. In alkaline condition, more HOCl would be converted to OCl−, leading to the reduction in PAHs removal. |
HOCl ↔ H+ + OCl−, pKa = 7.5 at 25 °C
| (2) |
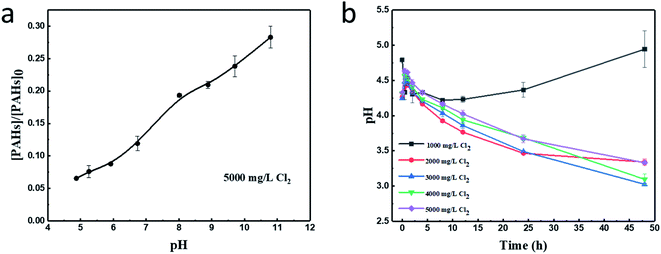 |
| Fig. 1 (a) Effect of pH on the PAHs degradation when the chlorine dosage was set as 5000 mg L−1 with a reaction time of 48 h. (b) Variation of pH values during the PAHs degradation process with different chlorine dosages. | |
With an initial pH of 7.3, the variation of pH values in the reaction system during the PAHs degradation process was recorded and shown in Fig. 1b. At first, the addition of chlorine increased the acidity of the reaction system. The pH values turned to 4.8, 4.3, 4.2, 4.3, 4.3 at the presence of 1000, 2000, 3000, 4000, 5000 mg L−1 chlorine, respectively. There was a similar trend that the pH of the reaction system decreased with time when the chlorine dosages were 2000, 3000, 4000 and 5000 mg L−1. This was attributed to the formed HOCl that could improve the soil acidity. However, for the initial chlorine concentration of 1000 mg L−1, the pH decreased to 4.2 in the first eight hours, and then increased to 4.9 in forty-eight hours. This might be the synergetic effect of the buffer capacity of soil and the acidity regulation of the small amount of newly formed HOCl.
3.2 Effect of temperature on PAHs degradation
To further elucidate the temperature-dependent PAHs degradation by HOCl oxidation, experiments were conducted at 15, 25 and 35 °C, respectively, and results were plotted in Fig. 2a and b. In Fig. 2a, about 92.76%, 93.33% and 94.2% of PAHs were removed after 48 h's reaction at 15, 25 and 35 °C. When the temperature rose by 10 °C, the degradation efficiency increased by less than 1%. Therefore, the degradation of PAHs in contaminated industrial soil by HOCl oxidation was not affected significantly by temperature variation.
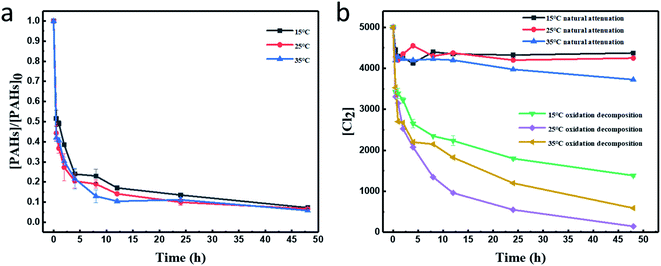 |
| Fig. 2 Influence of temperature on (a) PAHs degradation and (b) chlorine consumption. Experimental conditions: [PAHs]0 = 2469.9 mg kg−1, [Cl2]0 = 5000 mg L−1, pH = 5. | |
Fig. 2b displayed the chlorine consumption curves of PAHs degradation in contaminated industrial soil at different temperatures. As the temperature increased from 15 to 35 °C, the consumption of chlorine had a slightly rise during the natural attenuation process. Similarly, there was a clearly rising in the consumption of chlorine during the oxidation decomposition process along with the temperature increasing. The effect of temperature on the degradation of PAHs by HOCl oxidation was attributed to the consumption of chlorine varying along with the temperature.
3.3 Effect of chlorine dosage on PAHs degradation
The time-dependent degradation of PAHs in contaminated industrial soil with different chlorine dosages at pH 5 was illustrated in Fig. 3a. With an initial concentration of 2469.9 mg kg−1, PAHs was degraded gradually with time elapsing. About 37.53%, 66.63%, 83.52%, 90.98% and 93.33% of PAHs were removed after 48 h with the addition of 1000, 2000, 3000, 4000 and 5000 mg L−1 of chlorine, respectively. Besides, the degradation efficiency of PAHs with different number of rings was analyzed. When the effective chlorine dosage was set as 5000 mg L−1, about 96.78% of low-molecular-weight PAHs with two and three rings and 92.57% of high-molecular-weight PAHs with four to six rings were removed after 48 h, which indicated that the degradation efficiency was probably related to their number of rings. The degradation efficiency of PAHs increased with the increase of chlorine dosage. Similarly, each degradation curve could be divided into two stages. The concentration of PAHs dropped rapidly in the first 1 h, and there existed an initial linear degradation curve. After the fast degradation stage, approximately 31.61%, 47.63%, 53.53%, 57.74% and 63.27% of PAHs were removed in the presence of 1000, 2000, 3000, 4000, 5000 mg L−1 of chlorine, respectively. In the following slow degradation stage, about 5.92%, 19%, 29.99%, 33.25% and 30.06% of PAHs were degraded in 47 h, which suggested that the degradation rate of PAHs gradually decreased. The same trend could also be discovered in Fig. 3d, which showed the consumption of effective chlorine in the soil slurry. As the reaction went on, the concentration of effective chlorine declined. And there was a sharp drop in the concentration of chlorine in the first 1 h. More than 50% of chlorine was consumed in the soil slurry with different chlorine dosages, which suggested that the fast degradation of PAHs in the initial reaction stage might be attributed to direct electron transfer between chlorine and PAHs. As shown in Fig. 3d, when the chlorine dosage was set as 1000 mg L−1, all the chlorine could be almost used up in 12 h. This was in consistence with the slow or no degradation of PAHs after 12 h in the degradation curve that exhibited in Fig. 3a. Through the comparative analysis of Fig. 3a and d, it could be found that the degradation of PAHs was closely related to the concentration of effective chlorine in the reaction system. If there was little or no chlorine remained, the degradation process would slow down or come to an end. Compared the degradation efficiency of PAHs by HClO with other reported oxidants such as persulfate, potassium permanganate, Fenton reagents, ozone and some combined oxidation systems, it was found that the degradation rate of 93.33% obtained in this work was much higher, indicating its great potential in the remediation of PAHs contaminated industrial soil (Table S3†).
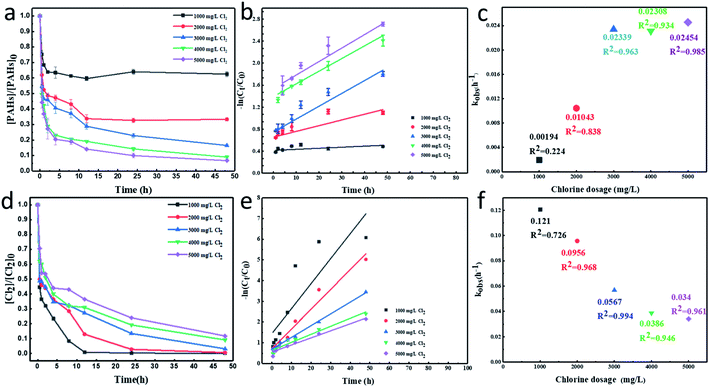 |
| Fig. 3 (a) The time-dependent degradation of PAHs with different chlorine dosages. (b) Plot of the pseudo-first-order kinetic model for PAHs degradation. (c) The kobs at different chlorine dosages. (d) The time-dependent consumption of chlorine with different chlorine dosages. (e) Plot of the pseudo-first-order kinetic model for chlorine consumption. (f) The kobs at different chlorine dosages. | |
Fig. 3b showed that the degradation behavior of PAHs with different chlorine dosages fitted well with pseudo-first-order kinetic model. Related equations and detailed statistical data of the model fitting could be found in the ESI (Table S4†). And the pseudo-first-order rate constants (kobs) were displayed in Fig. 3c. The chlorine dosage increased from 1000, 2000, to 5000 mg L−1, kobs of PAHs increased from 0.00194, 0.01043, to 0.02454 h−1, correspondingly. This illustrated that the degradation rate was affected by the concentration of chlorine in the soil slurry. Similarly, the consumption behavior of chlorine also fitted well with pseudo-first-order kinetic model. When the chlorine dosage was set as 1000 mg L−1, the kobs was determined as 0.121 h−1. The kobs decreased to 0.034 when the addition of chlorine turned to 5000 mg L−1. This suggested that the consumption rate of chlorine was negatively correlated with the chlorine dosage.
3.4 Mechanisms, possible products and proposed pathways of PAHs degradation
To explore the mechanisms of PAHs degradation by HOCl oxidation in contaminated industrial soil, the contribution of radical species was studied by radical scavenging tests. Since IPA can react rapidly with hydroxyl radicals (OH˙) with a rate constant of 3 × 109 M−1 s−1, it was employed as a scavenger for OH˙.39–41 TBA can scavenge OH˙ and Cl˙ significantly.42,43 Meanwhile, PBQ was chosen as a probe for superoxide radicals (˙O2−) due to its high reactivity.44 Fig. 4 showed the PAHs degradation behavior in the presence of IPA and PBQ, respectively. Fig. S2† displayed the effect of TBA on the PAHs degradation process. From Fig. 4a, it could be found that the degradation of PAHs was inhibited by the addition of IPA. The PAHs degradation efficiency turned to 84.53% in the presence of 150 mM IPA after 24 h, while under the condition of no IPA added, this value was 93.1%. With the addition of 230, 300 and 400 mM IPA, about 70.04%, 69.35% and 69.66% of PAHs was removed, respectively. The inhibition effect of IPA distinguished the roles of OH˙ in the degradation of PAHs. This also implied that the removal of around 20% PAHs was ascribed to the oxidation effect of OH˙. It could be found in Fig. S2† that the inhibitory effect of TBA was roughly similar with that of IPA, suggesting that Cl˙ had little impact on the PAHs degradation system. Fig. 4b showed that the degradation efficiency of PAHs had slight change with increasing PBQ dosages, indicating that the effect of ˙O2− was negligible.
 |
| Fig. 4 The effect of (a) IPA and (b) PBQ dosages on the PAHs degradation in the contaminated industrial soil by HOCl oxidation. Experimental conditions: [PAHs]0 = 2469.9 mg kg−1, [Cl2]0 = 5000 mg L−1, pH = 5. | |
Furthermore, the degradation products of PAHs during the HOCl oxidation process was identified by GC-MS in aqueous solution using anthracene, phenanthrene, pyrene and fluoranthene as representative pollutants, respectively. The chemical structures and physicochemical characteristics of these four PAH congeners were summarized in Table S5.† The total ion chromatograms and mass spectra of anthracene, phenanthrene, pyrene, fluoranthene and their degradation products were displayed in Fig. S3 and S4.† Based on the total ion chromatograms, mass spectra and the authentic standard, the structures of the identified products were exhibited in Table 1.
Table 1 Possible degradation products of PAHs by HOCl oxidation determined by GC-MS
Products |
Chemical name |
Retention time/min |
Chemical structure |
1 |
Anthracene |
15.825 |
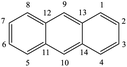 |
2 |
Anthrone |
17.54 |
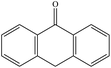 |
3 |
9,10-Anthracenedione |
17.8 |
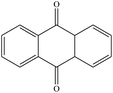 |
4 |
9,10-Dichloro-anthracene |
20.235 |
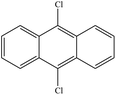 |
5 |
9-Chlorophenanthrene |
18 |
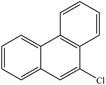 |
6 |
Diphenic anhydride |
18.72 |
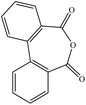 |
7 |
9,10-Phenanthrenedione |
20.05 |
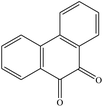 |
8 |
Pyrene |
19.365 |
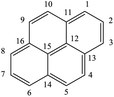 |
9 |
1-Chloropyrene |
21.67 |
 |
10 |
Phenanthro[3,4-c]furan-1,3-dione |
23.315 |
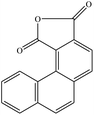 |
11 |
1,6,8-Trichloro-pyrene |
25.45 |
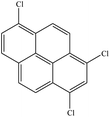 |
12 |
Fluoranthene |
18.775 |
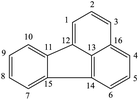 |
13 |
5,6-Dichloro-acenaphthylenone |
21.045 |
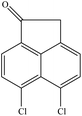 |
From Table 1, it could be found that PAHs congeners mainly generated chlorinated polycyclic aromatic hydrocarbons (Cl-PAHs) and oxygenated polycyclic aromatic hydrocarbons (O-PAHs) in the degradation process by HOCl oxidation. However, for each PAH congener, the categories of degradation products were different. Previous studies have successfully applied Fukui index (f−1(r)) to predict degradation products for electrophilic reactions, and the highest f−1(r) value indicates the most reactive position.45,46 For anthracene and phenanthrene, the value is the highest at the 9 and 10 positions, which means 9 and 10 positions are the most reactive sites for electrophilic attack.47 Correspondingly, it was observed that anthracene was degraded into 9,10-anthracenedione and 9,10-dichloro-anthracene, respectively. Anthrone was also detected as the degradation intermediate. In the degradation process by HOCl oxidation, HOCl was acted as an oxidant, capturing one electron from anthracene to form a radical cation, which then suffered a nucleophilic attack by water to produce anthrone. Subsequently, anthrone underwent another single electron transfer and was nucleophilic attacked by a second water molecule to get 9,10-anthracenedione.48 In addition, the formation of a portion of 9,10-anthracenedione was ascribed to the oxidation effect of OH˙.49 Proposed degradation pathways of anthracene by HOCl and OH˙ were shown in Fig. 5. What's more, 9,10-dichloro-anthracene was generated by aromatic electrophilic substitution reaction. In the degradation system of phenanthrene, 9-chlorophenanthrene, diphenic anhydride and 9,10-phenanthrenedione were identified as products. A single electron transfer followed by nucleophilic attacks from water molecules could be the mechanism for the formation of O-PAHs. The generation of Cl-PAHs was ascribed to the aromatic electrophilic substitution. For pyrene, f−1(r) value of 1(3,6,8)-position is higher than 4(5,9,10)-position.48 The detected 1-chloropyrene and 1,6,8-trichloro-pyrene were the Cl substitution results of pyrene. The degradation product of fluoranthene was detected as 5,6-dichloro-acenaphthylenone, which might be obtained by ring rupture of fluoranthene, further oxidation and Cl substitution in the HOCl oxidation process.
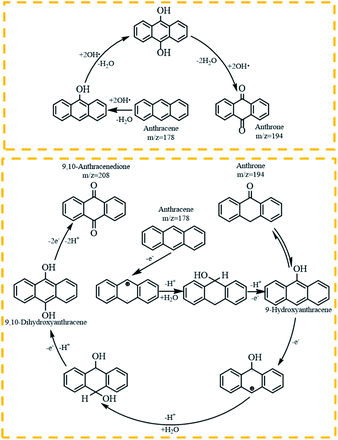 |
| Fig. 5 Proposed degradation pathway of anthracene by HOCl oxidation.48,49 | |
As mentioned above, in our previous study, the remediation of PAHs in real contaminated industrial soil could be realized by chlorine dioxide with a degradation efficiency of 84.24%.36 It was proved that HOCl played a key role in the PAHs degradation process. Inspired by former conclusions, sodium hypochlorite, whose effective ingredient is HOCl, was applied directly to achieve PAHs degradation. In this study, the degradation efficiency was improved to 93.33% by HOCl oxidation, demonstrating a more convenient and efficient method for PAHs contaminated soil remediation. However, it was identified that the degradation products of PAHs in this method were Cl-PAHs and O-PAHs. Among them, Cl-PAHs have been arising wide public concern due to their dioxin-like toxicity, which can pose potential hazardous effect on human health.48 Therefore, to realize the complete degradation (to improve the mineralization ratio) of PAHs in contaminated industrial soil is the main task in the soil remediation. Catalytic-enhanced and UV-assisted photodegradation technologies might be the effective ways that need further exploring.50
4. Conclusions
In this study, the PAHs degradation in real contaminated industrial soil by HOCl oxidation was investigated in detail. The parameters that had effects on the PAHs degradation, the kinetics, the possible products, and the mechanisms were explored in depth. Results demonstrated that the degradation efficiency of PAHs increased with an increasing in initial chlorine concentration. An extremely high degradation efficiency of 93.33% was realized after 48 h in the presence of 5000 mg L−1 chlorine at pH = 5. The PAHs degradation behavior was in accordance with the pseudo-first-order kinetic model. The pH of the reaction system played an important role in the degradation of PAHs. In the range of 4.9 to 10.9, the PAHs degradation efficiency exhibited a substantial decline with pH increasing. What's more, the influence of the temperature on the degradation process was slight and could be negligible. The results of the radical scavenging tests revealed that the hydroxyl radical was one of the contributors of the PAHs degradation. The mechanisms of the degradation of PAHs by HOCl oxidation were ascribed to the direct oxidation of HOCl and indirect oxidation of OH˙. Economically, the price of 0.5% sodium hypochlorite solution is $5.5 per ton, which effective chlorine concentration is about 5000 mg L−1. What's more, it can be used directly without activation by other reagents. HClO oxidation has been proved to be an efficient, convenient, and cost-effective method to realize PAHs degradation in contaminated industrial soil. The techniques to further enhance the degradation effects will be explored in the future.
Conflicts of interest
The authors declare no competing financial interest.
Acknowledgements
This work was supported by “Shuguang Scholar Program” (17SG52) by Shanghai Education Development Foundation and Shanghai Municipal Education Commission and Liaoning Provincial Natural Science Fund Project (2019-ZD-0550), and Scientific Research Foundation of Shanghai Institute of Technology (YJ2021-27).
References
- S. Lamichhane, K. C. Bal Krishna and R. Sarukkalige, Polycyclic aromatic hydrocarbons (PAHs) removal by sorption: A review, Chemosphere, 2016, 148, 336–353 CrossRef CAS PubMed.
- M. Kumar, N. S. Bolan, S. A. Hoang, A. D. Sawarkar, T. Jasemizad, B. Gao, S. Keerthanan, L. P. Padhye, L. Singh, S. Kumar, M. Vithanage, Y. Li, M. Zhang, M. B. Kirkham, A. Vinu and J. Rinklebe, Remediation of soils and sediments polluted with polycyclic aromatic hydrocarbons: To immobilize, mobilize, or degrade?, J. Hazard. Mater., 2021, 420, 126534 CrossRef CAS PubMed.
- Y. Gou, S. Yang, Y. Cheng, Y. Song, P. Qiao, P. Li and J. Ma, Enhanced anoxic biodegradation of polycyclic aromatic hydrocarbons (PAHs) in aged soil pretreated by hydrogen peroxide, Chem. Eng. J., 2019, 356, 524–533 CrossRef CAS.
- K. Bao, C. Zaccone, Y. Tao, J. Wang, J. Shen and Y. Zhang, Source apportionment of priority PAHs in 11 lake sediment cores from Songnen Plain, Northeast China, Water Res., 2020, 168, 115158 CrossRef CAS PubMed.
- J. E. Balmer, H. Hung, Y. Yu, R. J. Letcher and D. C. G. Muir, Sources and environmental fate of pyrogenic polycyclic aromatic hydrocarbons (PAHs) in the Arctic, Emerging Contam., 2019, 5, 128–142 CrossRef.
- Z. Yuan, B. He, X. Wu, S. L. M. Simonich, H. Liu, J. Fu, A. Chen, H. Liu and Q. Wang, Polycyclic aromatic hydrocarbons (PAHs) in urban stream sediments of Suzhou Industrial Park, an emerging eco-industrial park in China: Occurrence, sources and potential risk, Ecotoxicol. Environ. Saf., 2021, 214, 112095 CrossRef CAS PubMed.
- B. Ranc, P. Faure, V. Croze and M. O. Simonnot, Selection of oxidant doses for in situ chemical oxidation of soils contaminated by polycyclic aromatic hydrocarbons (PAHs): A review, J. Hazard. Mater., 2016, 312, 280–297 CrossRef CAS.
- X. Bai, Y. Wang, X. Zheng, K. Zhu, A. Long, X. Wu and H. Zhang, Remediation of phenanthrene contaminated soil by coupling soil washing with Tween 80, oxidation using the UV/S2O82− process and recycling of the surfactant, Chem. Eng. J., 2019, 369, 1014–1023 CrossRef CAS.
- J. Wang, Z. Luo, Y. Song, X. Zheng, L. Qu, J. Qian, Y. Wu, X. Wu and Z. Wu, Remediation of phenanthrene contaminated soil by g-C3N4/Fe3O4 composites and its phytotoxicity evaluation, Chemosphere, 2019, 221, 554–562 CrossRef CAS PubMed.
- Y. Gou, Q. Zhao, S. Yang, P. Qiao, Y. Cheng, Y. Song, Z. Sun, T. Zhang, L. Wang and Z. Liu, Enhanced degradation of polycyclic aromatic hydrocarbons in aged subsurface soil using integrated persulfate oxidation and anoxic biodegradation, Chem. Eng. J., 2020, 394, 125040 CrossRef CAS.
- L. Famiyeh, K. Chen, J. Xu, Y. Sun, Q. Guo, C. Wang, J. Lv, Y. Tang, H. Yu, C. Snape and J. He, A review on analysis methods, source identification, and cancer risk evaluation of atmospheric polycyclic aromatic hydrocarbons, Sci. Total Environ., 2021, 789, 147741 CrossRef CAS PubMed.
- C. Trellu, Y. Pechaud, N. Oturan, E. Mousset, E. D. van Hullebusch, D. Huguenot and M. A. Oturan, Remediation of soils contaminated by hydrophobic organic compounds: How to recover extracting agents from soil washing solutions?, J. Hazard. Mater., 2021, 404, 124137 CrossRef CAS PubMed.
- Y. Wang, M. Wang, Y. Chen, C. Li and Z. Zhou, Microbial community structure and co-occurrence are essential for methanogenesis and its contribution to phenanthrene
degradation in paddy soil, J. Hazard. Mater., 2021, 417, 126086 CrossRef CAS PubMed.
- Q. Leng, J. Mu and G. Yang, Efficient anaerobic bioremediation of high-concentration benzo[a]pyrene in marine environments, Environ. Pollut., 2021, 284, 117210 CrossRef CAS PubMed.
- M. Cheng, G. Zeng, D. Huang, C. Yang, C. Lai, C. Zhang and Y. Liu, Advantages and challenges of Tween 80 surfactant-enhanced technologies for the remediation of soils contaminated with hydrophobic organic compounds, Chem. Eng. J., 2017, 314, 98–113 CrossRef CAS.
- W. Tan, N. Liu, Q. Dang, D. Cui, B. Xi and H. Yu, Insights into the removal efficiencies of aged polycyclic aromatic hydrocarbons in humic acids of different soil aggregate fractions by various oxidants, Environ. Pollut., 2020, 264, 114678 CrossRef CAS PubMed.
- E. V. Lau, S. Gan, H. K. Ng and P. E. Poh, Extraction agents for the removal of polycyclic aromatic hydrocarbons (PAHs) from soil in soil washing technologies, Environ. Pollut., 2014, 184, 640–649 CrossRef CAS PubMed.
- D. Ghosal, S. Ghosh, T. K. Dutta and Y. Ahn, Current State of Knowledge in Microbial Degradation of Polycyclic Aromatic Hydrocarbons (PAHs): A Review, Front. Microbiol., 2016, 7, 1369 Search PubMed.
- S. Kuppusamy, P. Thavamani, K. Venkateswarlu, Y. B. Lee, R. Naidu and M. Megharaj, Remediation approaches for polycyclic aromatic hydrocarbons (PAHs) contaminated soils: Technological constraints, emerging trends and future directions, Chemosphere, 2017, 168, 944–968 CrossRef CAS PubMed.
- C. Trellu, E. Mousset, Y. Pechaud, D. Huguenot, E. D. van Hullebusch, G. Esposito and M. A. Oturan, Removal of hydrophobic organic pollutants from soil washing/flushing solutions: A critical review, J. Hazard. Mater., 2016, 306, 149–174 CrossRef CAS PubMed.
- M. Cheng, G. Zeng, D. Huang, C. Lai, P. Xu, C. Zhang and Y. Liu, Hydroxyl radicals based advanced oxidation processes (AOPs) for remediation of soils contaminated with organic compounds: A review, Chem. Eng. J., 2016, 284, 582–598 CrossRef CAS.
- Y. Liu, S. Shi and Y. Wang, Removal of pollutants from gas streams using Fenton (-like)-based oxidation systems: A review, J. Hazard. Mater., 2021, 416, 125927 CrossRef CAS.
- C. L. Yap, S. Gan and H. K. Ng, Fenton based remediation of polycyclic aromatic hydrocarbons-contaminated soils, Chemosphere, 2011, 83, 1414–1430 CrossRef CAS PubMed.
- M. Usman, K. Hanna and S. Haderlein, Fenton oxidation to remediate PAHs in contaminated soils: A critical review of major limitations and counter-strategies, Sci. Total Environ., 2016, 569–570, 179–190 CrossRef CAS PubMed.
- X. Lai, X. Ning, Y. Zhang, Y. Li, R. Li, J. Chen and S. Wu, Treatment of simulated textile sludge using the Fenton/Cl− system: The roles of chlorine radicals and superoxide anions on PAHs removal, Environ. Res., 2021, 197, 110997 CrossRef CAS PubMed.
- Z. Zhou, X. Liu, K. Sun, C. Lin, J. Ma, M. He and W. Ouyang, Persulfate-based advanced oxidation processes (AOPs) for organic-contaminated soil remediation: A review, Chem. Eng. J., 2019, 372, 836–851 CrossRef CAS.
- U. Ushani, X. Lu, J. Wang, Z. Zhang, J. Dai, Y. Tan, S. Wang, W. Li, C. Niu, T. Cai, N. Wang and G. Zhen, Sulfate radicals-based advanced oxidation technology in various environmental remediation: A state-of-the-art review, Chem. Eng. J., 2020, 402, 126232 CrossRef CAS.
- J. Wang and S. Wang, Activation of persulfate (PS) and peroxymonosulfate (PMS) and application for the degradation of emerging contaminants, Chem. Eng. J., 2018, 334, 1502–1517 CrossRef CAS.
- F. Ghanbari and M. Moradi, Application of peroxymonosulfate and its activation methods for degradation of environmental organic pollutants: Review, Chem. Eng. J., 2017, 310, 41–62 CrossRef CAS.
- J. Lee, U. von Gunten and J. Kim, Persulfate-Based Advanced Oxidation: Critical Assessment of Opportunities and Roadblocks, Environ. Sci. Technol., 2020, 54, 3064–3081 Search PubMed.
- J. Li, S. Pang, Z. Wang, Q. Guo, J. Duan, S. Sun, L. Wang, Y. Cao and J. Jiang, Oxidative transformation of emerging organic contaminants by aqueous permanganate: Kinetics, products, toxicity changes, and effects of manganese products, Water Res., 2021, 203, 117513 CrossRef CAS PubMed.
- X. Xie, W. Zhao, Y. Hu, X. Xu and H. Cheng, Permanganate oxidation and ferric ion precipitation (KMnO4-Fe(III)) process for treating phenylarsenic compounds, Chem. Eng. J., 2019, 357, 600–610 CrossRef CAS.
- J. Wang and Z. Bai, Fe-based catalysts for heterogeneous catalytic ozonation of emerging contaminants in water and wastewater, Chem. Eng. J., 2017, 312, 79–98 CrossRef CAS.
- J. Wang and S. Wang, Reactive species in advanced oxidation processes: Formation, identification and reaction mechanism, Chem. Eng. J., 2020, 401, 126158 CrossRef CAS.
- Y. Lee and U. von Gunten, Oxidative transformation of micropollutants during municipal wastewater treatment: Comparison of kinetic aspects of selective (chlorine, chlorine dioxide, ferrateVI, and ozone) and non-selective oxidants (hydroxyl radical), Water Res., 2010, 44, 555–566 CrossRef CAS PubMed.
- Y. Sun, W. Niu, X. Hu, X. Ma, Y. Sun and Y. Wen, Oxidative degradation of polycyclic aromatic hydrocarbons in contaminated industrial soil using chlorine dioxide, Chem. Eng. J., 2020, 394, 124857 CrossRef CAS.
- W. Wang, Q. Wu, N. Huang, T. Wang and H. Hu, Synergistic effect between UV and chlorine (UV/chlorine) on the degradation of carbamazepine: Influence factors and radical species, Water Res., 2016, 98, 190–198 CrossRef CAS PubMed.
- Y. Zhu, M. Wu, N. Gao, W. Chu, K. Li and S. Chen, Degradation of phenacetin by the UV/chlorine advanced oxidation process: Kinetics, pathways, and toxicity evaluation, Chem. Eng. J., 2018, 335, 520–529 CrossRef CAS.
- A. L. Teel and R. J. Watts, Degradation of carbon tetrachloride by modified Fenton's reagent, J. Hazard. Mater., 2002, 94, 179–189 CrossRef CAS.
- X. Fu, X. Gu, S. Lu, V. K. Sharma, M. L. Brusseau, Y. Xue, M. Danish, G. Y. Fu, Z. Qiu and Q. Sui, Benzene oxidation by Fe(III)-activated percarbonate: matrix-constituent effects and degradation pathways, Chem. Eng. J., 2017, 309, 22–29 CrossRef CAS PubMed.
- G. V. Buxton, C. L. Greenstock, W. P. Helman and A. B. Ross, Critical Review of rate constants for reactions of hydrated electrons, hydrogen atoms and hydroxyl radicals (˙OH/˙O−) in Aqueous Solution, J. Phys. Chem. Ref. Data, 1988, 17, 513–886 CrossRef CAS.
- Z. Wu, K. Guo, J. Fang, X. Yang, H. Xiao, S. Hou, X. Kong, C. Shang, X. Yang and F. Meng, Factors affecting the roles of reactive species in the degradation of micropollutants by the UV/chlorine process, Water Res., 2017, 126, 351–360 CrossRef CAS PubMed.
- N. Y. Shafikov, A. A. Gusmanov and Y. S. Zimin, Kinetics of the Reaction of Ozone with tert-Butanol, Kinet. Catal., 2002, 43, 799–801 CrossRef CAS.
- Z. Ni, C. Zhang, Z. Wang, S. Zhao, X. Fan and H. Jia, Performance and potential mechanism of transformation of polycyclic aromatic hydrocarbons (PAHs) on various iron oxides, J. Hazard. Mater., 2021, 403, 123993 CrossRef CAS PubMed.
- M. Altarawneh and B. Z. Dlugogorski, Formation and Chlorination of Carbazole, Phenoxazine, and Phenazine, Environ. Sci. Technol., 2015, 49, 2215–2221 CrossRef CAS PubMed.
- X. Xu, D. Wang, C. Li, H. Feng and Z. Wang, Characterization of the reactivity and chlorinated products of carbazole during aqueous chlorination, Environ. Pollut., 2017, 225, 412–418 CrossRef CAS PubMed.
- B. Lee, M. Iso and M. Hosomi, Prediction of Fenton oxidation positions in polycyclic aromatic hydrocarbons by Frontier electron density, Chemosphere, 2001, 42, 431–435 CrossRef CAS PubMed.
- X. Xu, R. Xiao, D. D. Dionysiou, R. Spinney, T. Fu, Q. Li, Z. Wang, D. Wang and Z. Wei, Kinetics and mechanisms of the formation of chlorinated and oxygenated polycyclic aromatic hydrocarbons during chlorination, Chem. Eng. J., 2018, 351, 248–257 CrossRef CAS.
- V. Nguyen, L. Phan Thi, Q. Van Le, P. Singh, P. Raizada and P. Kajitvichyanukul, Tailored photocatalysts and revealed reaction pathways for photodegradation of polycyclic aromatic hydrocarbons (PAHs) in water, soil and other sources, Chemosphere, 2020, 260, 127529 CrossRef CAS PubMed.
- B. Shao, J. Zhu, G. Zhou, B. Pan, X. Zhang, L. Dong and X. Guan, Importance of High-Valent Iron Complex and Reactive Radicals in Organic Contaminants' Abatement by the Fe-TAML/Free Chlorine System, ACS ES&T Engg, 2021, 1, 1401–1409 Search PubMed.
Footnote |
† Electronic supplementary information (ESI) available. See DOI: 10.1039/d2ra00514j |
|
This journal is © The Royal Society of Chemistry 2022 |
Click here to see how this site uses Cookies. View our privacy policy here.