DOI:
10.1039/D2RA00803C
(Paper)
RSC Adv., 2022,
12, 11967-11973
Chiral fluorescent sensor based on H8-BINOL for the high enantioselective recognition of D- and L-phenylalanine†
Received
7th February 2022
, Accepted 5th April 2022
First published on 19th April 2022
Abstract
Phenylalanine an essential aromatic amino acid for humans and animals, cannot be synthesized by humans and animals on their own. However, it synthesizes important neurotransmitters and hormones in the body and is involved in gluconeogenesis and lipid metabolism. Moreover, the two opposite configurations of phenylalanine have different activities. For example, L-phenylalanine is a biologically active optical isomer involved in crucial biological processes, the lack of which will lead to intellectual disability, while D-phenylalanine only acts as a chiral intermediate. In this research, an H8-BINOL chiral fluorescent sensor modified with 1,2,3-triazole was synthesized in high yield (95%) by nucleophilic substitution and click reaction. The chiral fluorescent sensor showed high enantioselectivity toward phenylalanine. L-Phenylalanine enhanced the fluorescence response of the probe significantly, while D-phenylalanine had no obvious fluorescence response change. The enantioselective fluorescence enhancement ratio [ef = (IL − I0)/(ID − I0), where I0 is the fluorescence of the sensor without amino acids] for the highest fluorescence intensity at 20.0 equivalents of amino acids was 104.48. In this way, the probe could be used to identify and differentiate different configurations of phenylalanine.
1 Introduction
Chirality is a universal phenomenon and an important feature of nature, and is widely present in the generation of life and various evolutionary mechanisms.1–3 As most of the drugs people use in real life are chiral drugs, with further understanding and exploration of chiral issues, it is recognized that the enantiomers of some chiral compounds differ in their physiological effects and metabolic processes. Different isomers of the chiral drugs may exhibit different effects in physiological processes, so much that one enantiomer is effective in treating diseases while the other is harmful or fatal.4–6 Amino acids, which are essential for the maintenance of life, are also a class of chiral molecules.7–10 L-Phenylalanine is a physiologically active aromatic amino acid and is one of the essential amino acids that cannot be synthesized naturally by humans and animals.11 In addition to L-phenylalanine, a variety of commercially valuable derivatives can be produced through the L-phenylalanine biosynthetic pathway. L-Phenylalanine deficiency leads to a number of disorders such as phenylketonuria (PKU), one of the most common inherited disorders of amino acid metabolism that occurs due to the destruction of phenylalanine dehydrogenase (PAH)12 and manifests specifically with symptoms such as intellectual disability and irreversible brain damage.13–16 Studies have shown that L-phenylalanine can also be used as a carrier of anti-cancer drugs, introducing drug molecules directly to the nidus and the effect is three to five times that of other amino acids.17,18 However, D-phenylalanine is largely absent in human metabolism due to its specific structure and activity. It is also used as a very significant chiral intermediate in organic synthesis, new drug development, and the synthesis of peptide compounds.19,20 Therefore, it is very important to distinguish the enantiomers and detect the optical purity.
There are a number of techniques for detecting the enantiomer composition of chiral compounds. The traditional identification techniques consist of electrochemistry, ligand exchange, high-throughput screening, and spectroscopy, such as high-performance liquid chromatography (HPLC)21,22 and circular dichroism (CD)23 are commonly used. Colorimetry and fluorescent probes are excellent approaches to identify enantiomers on account of their high-pitched visual resolution, high sensitivity, ease of operation, convenient equipment, real-time imaging, and online monitoring.24–26 It has been used to differentiate the conformation of chiral molecules and determine enantiomeric composition and has led to numerous high quality and quantitative results in the enantioselective fluorescence identification of amino acids, carboxylic acids, and amines.
BINOL is a rigid structure, which is a very suitable substrate for fluorescent sensors.27–29 For example, Vivek Panyam Muralidharan has designed and synthesized chiral fluorescent BINOL bearing benzoisoquino-line-1,3-dione on the minor grooves. It was found to be a sensor for the identification of R-amino alcohols and to determine the enantiomeric composition of α-amino alcohols.27 BINOL is also easily modified and has multiple modification sites that can modulate its fluorescence by introducing various moieties at different positions. Because of the unique chirality, BINOL and its derivatives can also provide interesting fluorescence signals and good chiral recognition. In the past few years, BINOL and its derivatives have gained global visibility in the field of enantioselective fluorescent sensor, which was used in the recognition of chiral molecules.30–34 H8-BINOL is the 5,5′,6,6′ site reduction of BINOL. Similar to BINOL, due to the electron giving action of the alkyl ring and the sp3 hybridization of the carbon, the inductive effect causes the electron density of the benzene ring of H8-BINOL to increase and higher reaction activity. Most previous reports on H8-BINOL focused on asymmetric catalysis.35–40 However, few reports paid attention to the enantioselective fluorescent sensors to date.41 In 2011, Pu et al. reported H8-BINOL-diamine compounds that displayed a good enantioselective fluorescent response toward mandelic acid, and the enantioselective fluorescence enhancement ratio was up to 3.5.42 Therefore, a novel H8-BINOL-based triazole-modified fluorescent probe was designed and synthesized to investigate the enantioselective recognition of phenylalanine in this study.
2 Experimental
2.1 Materials and methods
All experimental reagents used in the experiments were purchased from Energy Chemicals and Shanghai Aladdin and were not sonically purified. All analytical grade solvents were distilled prior to use. The solvents used for the probe synthesis experiments were purchased from Innochem. 1H NMR and 13C NMR were measured on a Bruker AM-400WB spectrometer using CDCl3. Fluorescence spectra data were measured on a Hitachi F-7100 fluorescence spectrophotometer with slit widths of 2.5 nm and 2.5 nm, respectively (λex = 260 nm). Melting points were carried out using an X-4 melting point tester. The optical rotation was measured on a Rudolph AUTOPOL IV automatic polarimeter. Elemental analyses were performed on a Vario EL/MACRO elemental analyzer using polyethylene as a blank control.
2.1.1 Synthesis of propargyl derivatives a and b. R-5,5′,6,6′,7,7′,8,8′-Octahydrobiol (1.00 g, 3.40 mmol) and K2CO3 (1.08 g, 7.81 mmol) were put in a 100 mL single-necked flask and 30 mL of acetone was added to dissolve them. Then, 3-bromopropyne (0.73 mL, 8.48 mmol) was added slowly dropwise to the reaction system. After the mixture was stirred for 20 min, the reaction was heated to about 55 °C for refluxing and stirred overnight. The reaction was intercepted, while thin layer chromatography detection proved the vanishing point of the start material and a new blot was generated. The temperature of the system was then reduced to ambient temperature, and the reaction solution was recovered and filtered, washed three times with acetone to collect the light yellow liquid, and dried with anhydrous MgSO4. Then, the crude product was obtained by rotary concentration on a rotary evaporator and later separated by column chromatography (silica gel 200–300 mesh, eluting solvent petroleum ether: ethyl acetate = 15
:
1, v/v) to get 0.48 g (derivatives a) of white solid in 38% yield, together with 0.45 g (derivatives b) of a white solid with a yield of 40%. [derivatives a, M.p. 97–99 °C. [α]25D +0.50 (c 0.2, CH3OH). 1H NMR (400 MHz, chloroform-d) δ 7.05 (d, J = 8.5 Hz, 1H), 6.90 (dd, J = 17.7, 8.4 Hz, 2H), 6.70 (s, 1H), 4.51 (t, J = 2.2 Hz, 2H), 4.31 (s, 1H), 2.67 (q, J = 8.7, 7.5 Hz, 4H), 2.36–2.11 (m, 3H), 2.10–1.83 (m, 2H), 1.80–1.40 (m, 10H)]. Elemental analysis results: C (experimental value: 83.01%, calculated value: 83.10%), H (experimental value: 7.09%, calculated value: 7.28%), O (experimental value: 9.90%, calculated value: 9.63%). [derivatives b, M.p. 86–87 °C. [α]25D +0.35 (c 0.2, CH3OH). 1H NMR (400 MHz, chloroform-d) δ 7.09 (d, J = 8.4 Hz, 2H), 6.94 (d, J = 8.4 Hz, 2H), 4.56 (d, J = 2.4 Hz, 4H), 2.79 (td, J = 6.4, 2.8 Hz, 4H), 2.39 (t, J = 2.3 Hz, 2H), 2.08 (q, J = 5.8, 5.0 Hz, 1H), 1.79–1.66 (m, 6H), 1.58 (s, 1H)]. Elemental analysis results: C (experimental value: 84.20%, calculated value: 84.29%), H (experimental value: 6.99%, calculated value: 7.07%), O (experimental value: 8.81%, calculated value: 8.64%).
2.1.2 Synthesis of R-1 probe. R-2-Alkynylmethoxy-5,5′,6,6′,7,7′,8,8′-octahydrobinol (200 mg, 0.81 mmol) was put in a 100 mL aubergine flask, evacuated several times, and protected by argon, and then 6 mL of tetrahydrofuran was added and stirred thoroughly to dissolve completely. Then 2-azidoacetate (0.09 mL, 0.97 mmol) was added to the mixture. Sodium ascorbate (129 mg, 1.70 mmol) and copper sulfate pentahydrate (337 mg, 0.81 mmol) were then accurately weighed, dissolved in 5 mL of water after a few minutes, and appended to the system. When the reaction was completed, it was monitored by thin layer chromatography and quenched by adding 15 mL of ice water to the reaction flask. It was then extracted three times with dichloromethane, collected, washed with prepared saturated brine, dried with anhydrous sodium sulphate for 30 min, and separated by column chromatography (silica gel 200–300 mesh, eluting solvent CH3OH
:
CH2Cl2 = 1
:
2, v/v). A white solid of 0.26 g was obtained with a yield of 95%. M.p. 93–95 °C. [α]25D +0.75 (c 0.2, CH3OH). Elemental analysis results: C (experimental value: 69.54%, calculated value: 69.79%), H (experimental value: 6.29%, calculated value: 6.49%), N (experimental value: 9.31%, calculated value: 9.40%), O (experimental value: 14.96%, calculated value: 14.32%). 1H NMR (400 MHz, chloroform-d) δ 7.24 (s, 1H), 7.09 (d, J = 8.4 Hz, 1H), 7.01 (d, J = 8.2 Hz, 1H), 6.93 (d, J = 8.4 Hz, 1H), 6.77 (d, J = 8.2 Hz, 1H), 5.48–4.92 (m, 4H), 3.79 (s, 3H), 3.11–2.70 (m, 4H), 2.36–2.06 (m, 4H), 1.85–1.43 (m, 11H), 1.26 (td, J = 7.1, 2.2 Hz, 2H). 13C NMR (101 MHz, chloroform-d) δ 153.26, 149.73, 145.06, 137.58, 135.84, 130.94, 129.77, 128.76, 123.23, 122.89, 111.76, 111.05, 62.45, 52.49, 50.20, 28.83, 26.81, 26.57, 22.64, 22.44.ppm. HRMS (ESI−): calcd for [C26H29N3O4+H−]– 448.2158; found 448.1774.
2.1.3 Preparation of fluorescent probe solutions. 44.7 mg of probes R-1, 44.7 mg of probes S-1, or 60.0 mg of probe R-2 were put in three 10 mL volumetric flasks, each; then, CH3OH (chromatographic grade) was added to dissolve and fix the volume to the scale line, was shaken well to dissolve completely and mixed well to obtain the required concentration of 0.001 moL l−1 of the master solution. Then, the above mixture was diluted with the same grade of methanol solution to the 2 × 10−5 mol L−1 test solution for testing (need to be ready to use).
2.1.4 Preparation of solutions required for the test. Common amino acids (D/L-alanine, D/L-aspartic acid, D/L-glutamine, D/L-glutamic acid, D/L-leucine, D/L-lysine, D/L-methionine, D/L-phenylalanine, D/L-proline, D/L-serine, D/L-valine, D/L-arginine, D/L-aspartic acid, D/L-tyrosine, D/L-cysteine, D/L-glycine) accurately weighed in a 5 mL volumetric flask, and then, 2000 μL of deionized water was pipetted dropwise to obtain a solution with a concentration of 0.1 mol L−1.
3 Results and discussion
3.1 Synthesis procedure
The synthesis process of sensors R-1 R-2 and S-1 is outlined in Scheme 1. R-5,5′,6,6′,7,7′,8,8′-Octahydrobiol (H8-BINOL) was used as the starting material, the fluorescent sensors R-1 and R-2 based on H8-BINOL derivatives modified with 2- and 2,2′-triazoles were obtained by a click reaction. According to the previous literature,43 R–H8-BINOL and 3-bromopropyne were synthesized as propargyl derivatives a and b. Propargyl derivative a and methyl azidoacetate were dissolved in tetrahydrofuran at room temperature and reacted with anhydrous copper sulphate and sodium ascorbate to afford 1,2,3-triazole modified H8-BINOL derivative R-1, which was further purified by column chromatography in a high yield of 95%. Similar probes R-2 and S-1 were synthesized by the same synthetic route with high yields (93% and 98%). The specific structures of the experimentally synthesized compounds were all validated by 1H NMR, 13C NMR, and ESI-MS.44
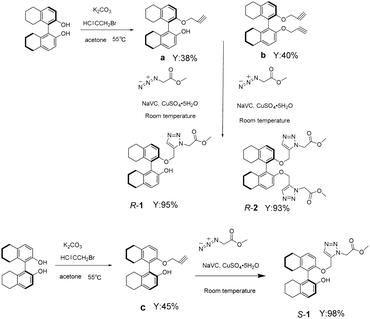 |
| Scheme 1 Synthetic procedure of fluorescent sensors R-1, R-2, and S-1. | |
3.2 Fluorescence study
The fluorescence responses of the R-1 probe toward two enantiomers of sixteen kinds of common amino acids without the involvement of metal ions were explored first. In this experiment, various amino acid (0.1 M) test solutions were prepared in distilled–deionized water or DMSO. Methanol was used to dissolve the R-1 probe (2.0 × 10−5 M). A pair of enantiomers (20.0 equivalents) of each amino acid was mixed into the solution at room temperature for fluorescence measurements. As shown in Fig. 1, L-phenylalanine and D-aspartic acid tremendously increased the fluorescence response of the R-1 probe, with I/I0 values of 2.04 and 1.43, respectively. However, D-phenylalanine and L-aspartic acid, as well as other amino acids, had little effect on the probes, with almost no fluorescence change. Thus, the novel chiral fluorescence R-1 exhibited a high degree of enantioselectivity and chemoselectivity for phenylalanine and aspartic acid.
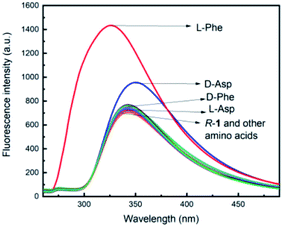 |
| Fig. 1 Fluorescence spectra of R-1 (2.0 × 10−5 M in CH3OH) with different enantiomers of sixteen ordinary amino acids (20.0 equivalents) in the absence of metal ions (λex = 260 nm, slits = 2.5/2.5 nm). | |
The fluorescence responses of the R-1 probe toward D-phenylalanine and L-phenylalanine were then studied in detail. The fluorescence spectra of the R-1 probe after the treatment with 20 equivalents of D-phenylalanine and L-phenylalanine are provided in Fig. 2a. For these measurements, the R-1 probe was blended in methanol (2.0 × 10−5 M) with either L-phenylalanine or D-phenylalanine (20 equivalents). The fluorescence spectrum of the R-1 probe at L-phenylalanine spiked from 5 to 100 equivalents, as given in Fig. 2c. As revealed in Fig. 2c, when the concentration of L-phenylalanine was raised to 20 equivalents, the fluorescence strength of the R-1 probe at 315 nm rose to 2.04 times its original value. However, when D-phenylalanine was used, the fluorescence of the R-1 probe did not change significantly over this concentration range (as shown in Fig. 2b). At 20.0 equivalents of amino acids, the maximum enantioselective fluorescence enhancement ratio45 [ef = (IL − I0)/(ID − I0), where I0 is the fluorescence of the sensor in the absence of amino acid] was 104.28. The fluorescence intensity of D-phenylalanine remained relatively constant with increasing equivalents (5–100 equivalents), while the fluorescence of L-phenylalanine then gradually increased and showed a linear relationship R = 0.9986, as shown in Fig. 2d.
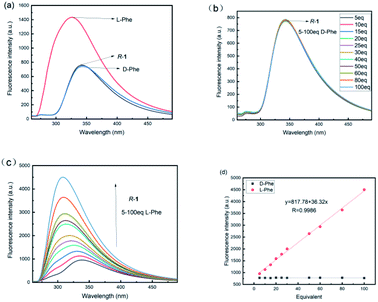 |
| Fig. 2 (A) Fluorescence spectra of R-1 (2.0 × 10−5 M in CH3OH) toward D- and L-phenylalanine (20.0 equiv.). (b) Fluorescence titration of R-1 with D-phenylalanine in CH3OH. (c) Fluorescence titration of R-1 with L-phenylalanine in CH3OH. (d) Fluorescence intensities at λ = 315 nm versus the equivalents of phenylalanine (λex = 260 nm, slits = 2.5/2.5 nm). | |
To investigate the enantiomeric composition of amino acids, the fluorescence response of R-1 with phenylalanine at different ee [ee,46 enantiomeric excess, = (l − d)/(l + d)] values were also studied. In the experiment, the R-1 probe was mixed with phenylalanine at different ee values and its fluorescence responses were then measured (Fig. 3). It was possible to measure the enantiomeric composition of amino acids when using R-1 with an excess of L-phenylalanine in methanol solution. To demonstrate the enantiomeric composition of amino acids, the fluorescence response of R-1 could be significantly enhanced by L-phenylalanine. As the excess of L-phenylalanine increased, the fluorescence intensity progressively enhanced.
 |
| Fig. 3 Fluorescence response at λem = 315 nm for the mixtures of R-1 toward L-phenylalanine (100.0 equiv.) at various ee values. | |
The large non-linear plot is shown in Fig. 3. It also demonstrated that the conformation of the amino acid did not improve the fluorescence, which could greatly curtail the fluorescence enhancement by its enantiomer. The most simple-minded approach to describe this phenomenon was that the two configurations of amino acids may bind to each other and slowly form a structure between them.47 This structure prevented them from binding to the probe for reaction. A surplus of the enantiomeric amino acid of the configuration that enhanced fluorescence reacted with the probe and turned on the fluorescence of the sensor, inducing it to enhance fluorescence.
The fluorescence reaction of the R-1 enantiomer S-1 toward D- and L-phenylalanine was also studied under the same conditions. As displayed in Fig. 4-a, the fluorescence responses of S-1 toward the phenylalanine enantiomer were identical to that of the R-1 probe, both recognizing only L-phenylalanine. We also investigated the fluorescence response of R-2, the double substitution product of R-1, toward D- and L-phenylalanine, as displayed in Fig. 4b. As shown, the fluorescence responses of R-2 to the phenylalanine enantiomer were identical to those of the R-1 probe. All three probes exhibited that L-phenylalanine could display a significant fluorescence enhancement, while D-phenylalanine exhibited no obvious change. Hence, it was clear that the recognition of phenylalanine was independent of the configuration of the probes and the degree of triazole modification. Further study was focused on the exact mechanism of the recognition.
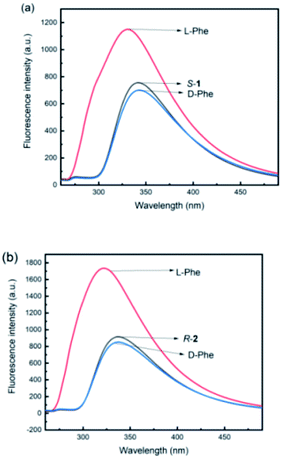 |
| Fig. 4 (a) Fluorescence spectra of R-2 (2.0 × 10−5 M in CH3OH) toward D- and L-phenylalanine (20.0 equiv.) in the absence of metal ions. (b) Fluorescence spectra of S-1 (2.0 × 10−5 M in CH3OH) toward D- and L-phenylalanine (20.0 equiv.) in the absence of metal ions (λex = 260 nm. Excitation/emission slit widths: 2.5/2.5 nm). | |
4 Conclusions
In summary, a novel fluorescent sensor of 1,2,3-triazole-modified H8-BINOL derivative with high correspondence selectivity was synthesized by nucleophilic substitution and click reaction with a high yield. It could be used as a novel chiral fluorescence sensor to identify and differentiate different configurations of phenylalanine as it exhibited high enantioselectivity and sensitivity to L-phenylalanine in the fluorescence experiments. The maximum enantioselective fluorescence enhancement ratio was 104.48 at 20.0 amino acid equivalents. The results showed that the novel synthesized sensor had the great possibility to be used as a fluorescent sensor to identify the different configurations of phenylalanine as well as to determine the enantiomeric composition of amino acids. Moreover, in contrast to previously reported amino acid fluorescent probes, the newly synthesized sensor also has the advantage of convenient use in observing fluorescence enhancement, because when many previously reported amino acid fluorescent probes are used in observing fluorescence enhancement, additional metals have to be used.
Conflicts of interest
There are no conflicts to declare.
Acknowledgements
The authors are grateful for the financial support of the National Natural Science Foundation of China (No. 21462018 and 21861025), the Science Fund of the Technology Office of Jiangxi, China (20192BAB203003), and the Jiangxi Science and Technology Normal University Program for Graduate Innovation Fund (YC2020-X21).
Notes and references
- S. Tokunaga, Y. Itoh, H. Tanaka, F. Araoka and T. Aida, Redox-Responsive Chiral Dopant for Quick Electrochemical Color Modulation of Cholesteric Liquid Crystal, J. Am. Chem. Soc., 2018, 140(35), 10946–10949 CrossRef CAS PubMed.
- M. Y. Mulla, E. Tuccori, M. Magliulo, G. Lattanzi, G. Palazzo, K. Persaud and L. Torsi, Capacitance-modulated transistor detects odorant binding protein chiral interactions, Nat. Commun., 2015, 6, 6010–6018 CrossRef CAS PubMed.
- L. J. Chen, H. B. Yang and M. Shionoya, Chiral Metallosupramolecular Architectures, Chem. Soc. Rev., 2017, 46, 2555–2576 RSC.
- N. Micali, H. Engelkamp, P. G. van Rhee, P. C. M. Christianen, L. M. Scolaro and J. C. Maan, Selection of supramolecular chirality by application of rotational and magnetic forces, Nat. Chem., 2012, 4, 201–207 CrossRef CAS PubMed.
- M. P. Tiwari and A. Prasad, Molecularly imprinted polymer based enantioselective sensing devices: A review, Anal. Chim. Acta, 2015, 853, 1–18 CrossRef CAS PubMed.
- Z. He, S. Zang, Y. Liu, Y. He and H. Lei, A multi-walled carbon nanotubes-poly(L-lysine) modified enantioselective immunosensor for ofloxacin by using multi-enzyme-labeled gold nanoflower as signal enhance, Biosens. Bioelectron., 2015, 73, 85–92 CrossRef CAS PubMed.
- V. Schurig Differentiation of Enantiomers. Springer. Heidelberg. 2013 Search PubMed.
- L. Pu, Fluorescence of Organic Molecules in Chiral Recognition, Chem. Rev., 2004, 104, 1687–1716 CrossRef CAS PubMed.
- G. A. Hembury, V. V. Borovkov and Y. Inoue, Chirality-sensing supramolecular systems, Chem. Rev., 2008, 108, 1–73 CrossRef CAS PubMed.
- L. Pu, Enantioselective fluorescent sensors: a tale of BINOL, Acc. Chem. Res., 2012, 45, 150–163 CrossRef CAS PubMed.
- J. Zhang, X. Zhou, D. Wang, Y. Wang, L. Chen and S. Tan, Selective Separation of L-Aromatic Amino Acid by L-Phenylalanine-Crosslinked Chitosan Resin, Asian J. Chem., 2013, 25(16), 9050–9054 CrossRef CAS.
- K. A. Konovalov, W. Wang and X. Huang, Conformational selection turns on phenylalanine hydroxylase, J. Biol. Chem., 2018, 293(51), 19544–19545 CrossRef CAS PubMed.
- K. Ikeda, E. Schiltz, T. Fujii, M. Takahashi, K. Mitsui, Y. Kodera and H. Nishimura, Phenylalanine ammonia-lyase modified with polyethylene glycol: Potential therapeutic agent for phenylketonuria, Appl. Biochem. Biotechnol., 2005, 29(3), 283–287 CAS.
- H. L. Levy, C. N. Sarkissian and C. R. Scriver, Phenylalanine ammonia lyase (PAL): From discovery to enzyme substitution therapy for phenylketonuria, Mol. Genet. Metab., 2018, 124(4), 223–229 CrossRef CAS PubMed.
- K. M. Sumaily and A. H. Mujamammi, A new look at an old topic, advances in laboratory diagnosis, and therapeutic strategies, Int. J. Health Sci., 2017, 11(5), 63–70 Search PubMed.
- E. R. Vardy, A. MacDonald, S. Ford and D. L. Hofman, Phenylketonuria, co-morbidity, and ageing: A review, J. Inherit. Metab. Dis., 2020, 43(2), 167–178 CrossRef PubMed.
- C. K. Savile, J. M. Janey, E. C. Mundorff, J. C. Moore, S. Tam, W. R. Jarvis, J. C. Colbeck, A. Krebber, F. J. Fleitz, J. Brands, P. N. Devine, G. W. Huisman and G. J. Hughes, Biocatalytic Asymmetric Synthesis of Chiral Amines from Ketones Applied to Sitagliptin Manufacture, Science, 2010, 329, 305–309 CrossRef CAS PubMed.
- S. Haldar, J. Chintapalli and C. M. Croce, Taxol Induces bcl-2 Phosphorylation and Death of Prostate Cancer Cells, Cancer Res., 1996, 56, 1253–1255 CAS.
- C. Lu, S. Zhang, W. Song, J. Liu, X. Chen, L. Liu and J. Wu, Efficient Synthesis of D-Phenylalanine from L-Phenylalanine via a Tri-Enzymatic Cascade Pathway, ChemCatChem, 2021, 13(13), 3165–3173 CrossRef CAS.
- M. Munoz, S. Recio, M. Rosso, M. Redondo, R. Covenas and J. Physiol, The antiproliferative action of [D-Arg(1), D-Phe(5), D-Trp(7,9), LEU(11)] substance Panalogue antagonist against small-cell- and non-small-cell lung cancer cells could be due to the pharmacological profile of its tachykinin receptor antagonist, Pharmacol, 2015, 66, 421–426 CAS.
- T. Noguchi, B. Roy, D. Yoshihara, J. Sakamoto, T. Yamamoto and S. Shinkai, Emergent Molecular Recognition through Self-Assembly: Unexpected Selectivity for Hyaluronic Acid among Glycosaminoglycans, Angew. Chem. Int. Ed., 2017, 56(41), 12518–12522 CrossRef CAS PubMed.
- A. Gogolashvili, E. Tatunashvili, L. Chankvetadze, T. Sohajda, J. Szeman, M. Gumustas, S. A. Ozkan, A. Salgado and B. Chankvetadze, Separation of enilconazole enantiomers in capillary electrophoresis with cyclodextrin-type chiral selectors and investigation of structure of selectorselectand complexes by using nuclear magnetic resonance spectroscopy, J. Chromatogr., A, 2018, 1571, 231–239 CrossRef CAS PubMed.
- I. Crnolatac, I. . Rogan, B. Majić and I. Piantanida, Small molecule probes finely differentiate between various ds- and ss-DNA and RNA by fluorescence, CD and NMR response, Anal. Chim. Acta, 2016, 940, 128–135 CrossRef CAS PubMed.
- T. Noguchi, B. Roy, D. Yoshihara, J. Sakamoto, T. Yamamoto and S. Shinkai, A Chiral Recognition System Orchestrated by Self-Assembly: Molecular Chirality, Self-Assembly Morphology, and Fluorescence Response, Angew. Chem., Int. Ed., 2017, 56(41), 12518–12522 CrossRef CAS PubMed.
- K. Wen, S. Yu, Z. Huang, L. Chen, M. Xiao, X. Yu and L. Pu, Rational Design of a Fluorescent Sensor to Simultaneously Determine Both the Enantiomeric Composition and the Concentration of Chiral Functional Amines, J. Am. Chem. Soc., 2015, 137(13), 4517–4524 CrossRef CAS PubMed.
- X. Zhang, J. Yin and J. Yoon, Recent advances in development of chiral fluorescent and colorimetric sensors, Chem. Rev., 2014, 114, 4918–4959 CrossRef CAS PubMed.
- V. P. Muralidharan and K. I. Sathiyanarayanan, Naphthalimide-Based Chiral Fluorescence Sensor Employing (S)-BINOL Unit for Highly Enantioselective Recognition of a-Amino Alcohols with Opposite Chiral Selectivity, ChemistrySelect, 2018, 3111–3117 CrossRef CAS.
- Z. B. Li, J. Lin and L. Pu, A Cyclohexyl-1,2-diamine-Derived Bis(binaphthyl) Macrocycle: Enhanced Sensitivity and Enantioselectivity in the Fluorescent Recognition of Mandelic Acid, Angew. Chem., Int. Ed., 2005, 44, 1690–1693 CrossRef CAS PubMed.
- Z. B. Li, J. Lin, M. Sabat, M. Hyacinth and L. Pu, Enantioselective Fluorescent Recognition of Chiral Acids by Cyclohexane-1,2-diamine-Based Bisbinaphthyl Molecules, J. Org. Chem., 2007, 72, 4905–4916 CrossRef CAS PubMed.
- K. Guo, P. Wang and W. Tan, et al., Structure of a Dimeric BINOL-Imine-Zn(II) Complex and Its Role in Enantioselective Fluorescent Recognition, Inorg. Chem., 2020, 59(24), 17992–17998 CrossRef CAS PubMed.
- L. Hu, S. Yu and Y. Wang, et al., Enhanced Fluorescence of 3,3'-Diformyl BINOL by Functional Secondary Amines, Org. Lett., 2017, 19(14), 3779–3782 CrossRef CAS PubMed.
- A. S. Gupta, G. Kumar, K. Paul and V. Luxami, BINOL-based differential chromo-fluorescent sensor and its application in miniaturized 1-2/4-2 bit encoders and decoders, New J. Chem., 2018, 42, 2491–2497 RSC.
- K. Wen, S. Yu and Z. Huang, et al., Rational Design of a Fluorescent Sensor to Simultaneously Determine Both the Enantiomeric Composition and the Concentration of Chiral Functional Amines, J. Am. Chem. Soc., 2015, 137, 4517–4524 CrossRef CAS PubMed.
- T. Hashimoto, Y. Naganawa and K. Maruoka, Desymmetrizing asymmetric ring expansion of cyclohexanones with α-diazoacetates catalyzed by chiral aluminum Lewis acid, J. Am. Chem. Soc., 2011, 133, 8834–8837 CrossRef CAS PubMed.
- Y. S. Fan, Y. J. Jiang, D. An, D. Sha, J. C. Antilla and S. Zhang, H8-BINOL chiral imidodiphosphoric acids catalyzed enantioselective synthesis of dihydroindolo-/-pyrrolo[1,2-a]quinoxalines, Org. Lett., 2014, 16(23), 6112–6115 CrossRef CAS PubMed.
- Y. Hayashi, N. Yamamura, T. Kusukawa and T. Harada, Enhancement of the Catalytic Activity of Chiral H8-BINOL Titanium Complexes by Introduction of Sterically Demanding Groups at the 3-Position, Chem. - Eur J., 2016, 22, 12095–12105 CrossRef CAS PubMed.
- C. Wang, P. Anbaei and L. Pu, Highly Enantioselective Fluorescent Recognition of Both Unfunctionalized and Functionalized Chiral Amines by a Facile Amide Formation from a Perfluoroalkyl Ketone, Chem. - Eur J., 2016, 22, 7255–7261 CrossRef CAS PubMed.
- S. Iqbal, S. Yu, L. Jiang, X. Wang, Y. Chen, Y. Wang, X. Yu and L. Pu, Simultaneous Determination of Concentration and Enantiomeric Composition of Amino Acids in Aqueous Solution by Using a Tetrabromobinaphthyl Dialdehyde Probe, Chem. - Eur J., 2019, 25(42), 9967–9972 CrossRef CAS PubMed.
- K. H. Lam, L. Xu, L. Feng, Q. H. Fan, F. L. Lam and W. h. Lo, Highly Enantioselective Iridium-Catalyzed Hydrogenation of Quinoline Derivatives Using Chiral Phosphinite H8-BINAPO, Adv. Synth. Catal., 2005, 347(14), 1755–1758 CrossRef CAS.
- K. Wu, M. H. Zhuo, D. Sha, Y. S. Fan, D. An, Y. J. Jiang and S. P. Zhang, H8-BINOL chiral imidodiphosphoric acid catalyzed highly enantioselective aza-Friede-Crafts reactions of pyrroles and enamides/imines, Chem. Commun., 2015, 51(38), 8054–8057 RSC.
- Y. Fangfang, C. Yun, J. Hui and W. Xuemei, Recent advances of BINOL-based sensors for enantioselective fluorescence recognition, Analyst, 2020, 145, 6769–6812 RSC.
- Y. Shanshan, M. Albert, DeBerardinis, M. Turlington and P. Lin, Study of the Fluorescent Properties of Partially Hydrogenated 1, 1'-Bi-2-naphthol-amine Molecules and Their Use for Enantioselective Fluorescent Recognition, J. Org. Chem., 2011, 76, 2814–2819 CrossRef PubMed.
- A. Rani, G. Singh, A. Singh, U. Maqbool, G. Kaur and J. Singh, CuAAC-ensembled 1,2,3-triazole-linked isosteres as pharmacophores in drug discovery: review, RSC Adv., 2020, 10, 5610–5635 RSC.
- B. Ashoka, N. Hariramb, S. Siengchinc and A. V. Rajulud, Modification of tamarind fruit shell powder with in situ generated copper nanoparticles by single step hydrothermal method, J. Bioresour. Bioprod.c, 2020, 5(3), 180–185 CrossRef.
- F. Zhao., Du. Yi, J. Tian., D. Shi., Y. Wang., L. Hu., Yu. Shanshan, X. Yu and Pu. Lin, Enantioselective Fluorescent Recognition of Amino Acids in Aqueous Solution by Using a Chiral Aldehyde Probe, Eur. J. Org Chem., 2018, 16, 1891–1895 CrossRef.
- S. Nian and Pu. Lin, Amphiphilic Polymer-Based Fluorescent Probe for Enantioselective Recognition of Amino Acids in Immiscible Water and Organic Phases, Chem. – Eur J., 2017, 23, 18066–18073 CrossRef CAS PubMed.
- M. NashirUddin, T. Ferdous, Z. Islam, M. S. Jahan and M. A. Quaiyyum, Development of chemometric model for characterization of non-wood by FT-NIR data, J. Bioresour. Bioprod., 2020, 5(3), 196–203 CrossRef.
|
This journal is © The Royal Society of Chemistry 2022 |
Click here to see how this site uses Cookies. View our privacy policy here.