DOI:
10.1039/D2RA00900E
(Paper)
RSC Adv., 2022,
12, 12552-12563
BiVO4/Fe2O3/ZnFe2O4; triple heterojunction for an enhanced PEC performance for hydrogen generation†
Received
11th February 2022
, Accepted 3rd April 2022
First published on 26th April 2022
Abstract
n/n/n triple heterojunction photoanodes made up of Zr:W-BiVO4, Fe2O3, and ZnFe2O4 metal oxides are fabricated through a simplistic spray pyrolysis method. Use of Zr and W as dopants in BiVO4 plays an important role as Zr increases the carrier density and W reduces the charge recombination. Further, Fe2O3 and ZnFe2O4 serve as a protective layer for Zr:W-BiVO4, which augmented the photoelectrochemical performance and achieved a 1.90% conversion efficiency in the triple heterojunction. XRD measurements display the crystalline nature and reduction in particle size due to strain in the sample, UV-vis absorbance shows an extended absorption towards the visible region and the FE-SEM imaging confirms the successful deposition of ZnFe2O4 over BiVO4/Fe2O3. By analyzing the band edge position, it was observed that on formation, the triple heterojunction not only suppresses the charge carrier recombination but also utilizes the band edge offset for the water splitting reaction using solar energy.
1. Introduction
India, which is the most polluted and largest economic country in the world, demands a speedy adaptation of energy from carbon-based fossil fuels to renewable energy sources with zero carbon emission to accomplish the target of 450 GW of renewable energy by the year 2030. In this transformation procedure, photoelectrochemical (PEC) water splitting utilizing solar energy-absorbing semiconductors for the production of green fuel hydrogen arises as a growing technique.1–4 Among different semiconductors, metal oxide semiconductors have gained attention due to their economical nature and stability as compared with that of other non-oxide semiconductors.5 BiVO4 and Fe2O3 with low bandgaps (1.8–2.2 eV) show good stability in the alkaline medium and are non-toxic and cost-effective materials. These are considered as excellent candidates for the oxidation reaction, but some limitations, such as the recombination of the electron–hole, smaller diffusion length, deprived electrical conductivity, etc., restrict their application as photoanodes.5–12 Recently, closed packed AIIBIIIO4 type spinel materials, viz. n-type zinc ferrite (ZnFe2O4), have gained inclusive curiosity as promising candidates for photoelectrochemical accomplishments as well as photocatalytic activities due to their distinctive properties, viz. a small bandgap (1.9 eV) for exploiting maximum visible light, brilliant photoelectrochemical stability in a basic medium, economical, nontoxic, environment and eco-friendly.13–19 However, the speedy recombination of charge carriers and low valence band potential are the major limitations of ZnFe2O4 in the field of photoelectrochemical studies.5,15,16 Their photoelectrochemical properties mostly depend upon morphology, chemical composition, and crystal size, which can be upgraded by introducing several alteration procedures, such as doping/co-doping, building a heterojunction system, use of co-catalyst, etc.10,20–22 Among these, heterojunction formation is considered as an excellent way to overcome the limitations of metal oxides due to its several advantages, viz. enhanced separation, reduced recombination of charge carriers, and extended absorption in the visible region.11,15,23,24
In this report, we fabricated a novel type-II heterojunction Zr-W:BiVO4/Fe2O3/ZnFe2O4 by a facile spray pyrolysis method. We have previously reported the fabrication of co-doped (Zr, W) bismuth vanadate films, and a maximum photoresponse of 0.13 mA cm−2 at 1.23 V/RHE was obtained with 3.5% Zr and 4% W dopant concentrations. In the present work, the deposition of Fe2O3 and ZnFe2O4 over the best performing Zr,W co-doped BiVO4 was expected to achieve the best PEC performance. The as-prepared type-II n/n/n triple heterojunction exhibited the superior PEC performance. The advantage of constructing this heterojunction falls on the following points: (i) comparable energy level of Fe2O3 over BiVO4; especially, the VB positions of Fe2O3 are more suitable for constructing a type-II heterojunction for a hole transfer reaction (a high-performance BiVO4 photoanode co-catalyzed with an ultrathin Fe2O3 layer for PEC application), (ii) similarly, the benefit of ZnFe2O4 over Fe2O3 lies in the straddling conduction band maxima position, as shown in Fig. 9(a), because the CB of ZnFe2O4 is more negative than that of Fe2O3.25,26 Hence, constructing Zr-W:BiVO4/Fe2O3/ZnFe2O4 for the first time could effectively utilize the band edge offset for a water-splitting reaction using solar energy.
2. Experimental section
2.1. Preparation of the triple heterojunction Zr-W:BiVO4/Fe2O3/ZnFe2O4 photoanode
The preparation of Zr:W-BiVO4/Fe2O3/ZnFe2O4 heterojunction thin films was completed in three steps. In the first step, Zr:W-BiVO4 thin films were prepared (the details of fabrication already reported by the authors, see ref. 29). In the second step, Fe2O3 thin films were prepared with a calculated amount of iron nitrate nonahydrate, which dissolved in DM water and deposited over Zr:W-BiVO4 at a flow rate of 500 μL min−1 at 250 °C temperature maintained over a hot plate. Finally, the films were annealed at a temperature of 450 °C for 2 h (the resulting thin films appear brown) to forms a double heterojunction, Zr:W-BiVO4 (symbolized as BF in the manuscript). In the last step, zinc ferrite thin films were prepared with a 1
:
2 ratio of zinc(II) nitrate hexahydrate and ferric nitrate nonahydrate mixed in DM water and deposited over the Zr-W:BiVO4/Fe2O3 thin films at a temperature of 250 °C to obtain the Zr-W:BiVO4/Fe2O3/ZnFe2O4 thin films (symbolized as BFZ) using a spray pyrolysis method (as shown in Fig. 1). Pristine ZnFe2O4 thin films were also prepared with the spray pyrolysis method at a 500 μL min−1 flow rate at 250 °C temperature.11,27–29 A detailed description of the prepared thin films is given in Table 1.
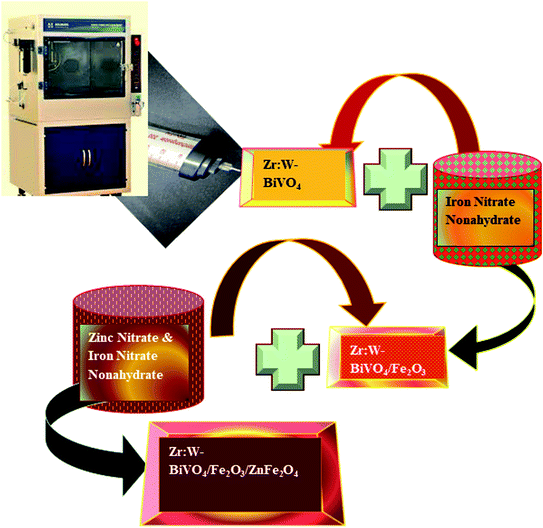 |
| Fig. 1 Schematic representation showing the preparation of the Zr:W-BiVO4/Fe2O3/ZnFe2O4 heterojunction thin film. | |
Table 1 Acronyms and description of the prepared thin films
Sample |
Acronym |
(3.5% Zr + 4% W-BiVO4) |
B |
Fe2O3 |
F |
ZnFe2O4 |
Z |
(3.5% Zr + 4% W-BiVO4) + Fe2O3 |
BF |
(3.5% Zr + 4% W-BiVO4) + ZnFe2O4 |
BZ |
Fe2O3 + ZnFe2O4 |
FZ |
(3.5% Zr + 4% W-BiVO4) + Fe2O3 + ZnFe2O4 |
BFZ |
2.2. Characterizations
2.2.1. General characterizations. XRD patterns of the prepared triple heterojunction thin films were recorded with a Bruker AXS D-8 Advance X-ray diffractometer with a Cu-Kα source having a wavelength of 1.54 Å. XRD data were further used to calculate the particle size using the Debye–Scherrer's equation.23,27 Field Emission Scanning Electron Microscopy (FE-SEM) using a JEOL, JSM 7610 F Plus system in connection with Oxford Energy Dispersive X-ray Spectroscopy (EDX) was employed for the morphology and compositional analysis. Further, the elemental composition was confirmed using X-ray photoelectron spectroscopy (PHI 5000 versa Probe II, FEI Inc.) equipped with a C60 sputter gun and Mg-Kα radiation with Ar ion. The absorbance–wavelength spectrum was recorded using a double beam UV-visible spectrophotometer (UV-2450, Shimadzu, Japan) in the 400–800 wavelength range and absorbance data were utilized for bandgap calculation using the Tauc plot.23,29,30
2.2.2. Photoelectrochemical and electrochemical characterizations. Photoelectrochemical (PEC) and electrochemical characterizations of the prepared heterojunction thin films were recorded using an electrochemical workstation (EWS, Model: PP211, CIMPS-pcs, Zahner, Germany), through backside illumination. A typical PEC cell consists of a saturated calomel electrode (SCE) as reference electrode, Pt electrode as a counter electrode, and a triple heterojunction photoanode as a working electrode dipped in 0.1 M NaOH solution at pH 13 was used for the PEC measurements. An area of 1 × 1 cm2 was exposed using a 150 W xenon arc lamp with 100 mW cm−2 light intensity.The photocurrent data obtained from the current–potential analysis were used to calculate the applied bias photon to current conversion efficiency (ABPE) using eqn (1).
|
ABPE (%) = Jph (mA cm−2){1.23 − Vb (V)}/PTotal (mW cm−2)
| (1) |
where
Jph = photocurrent density,
P = illumination intensity,
Vb =
Vmeas −
Voc (
Vmeas = photovoltage at which the photocurrent was measured,
Voc = open circuit potential). Mott–Schottky experiments were performed to find the flat band potential and charge carrier density (
Nd). The intercept of the 1/
C2 vs. the applied bias graph gives the value of the flat band potential (
eqn (2)) while
Nd was calculated using
eqn (3):
11,12 |
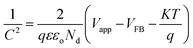 | (2) |
where,
q is the electronic charge,
ε and
εo are the permittivities of the semiconductor and vacuum, respectively,
S is the slope,
Nd is the donor density,
Vapp is the applied potential and
VFB is the flat band potential. The electrochemical surface area (ECSA) was also calculated using cyclic voltammetry under dark conditions and at different scan rates. The Nyquist analysis was executed with electrochemical impedance spectroscopy (EIS) to obtain the charge transfer kinetics at a 5 mV amplitude and in the frequency range of 100 MHz to 10 kHz. This analysis was performed under dark conditions.
3. Results and discussion
3.1. Crystal structure analysis: XRD spectrum
The crystal structure analysis was obtained from the XRD instrument. Fig. 2(a–d) displays the XRD patterns of Zr:W-BiVO4/Fe2O3/ZnFe2O4 (BFZ), Zr:W-BiVO4/Fe2O3 (BF), Fe2O3/ZnFe2O4 (FZ), Zr:W-BiVO4/ZnFe2O4 (BZ) along with Fe2O3 (F) and ZnFe2O4 (Z) which exhibited the polycrystalline nature of the prepared thin films. The diffraction peaks at 28.9° (121), 37.8° (220), 39.4°
, and 47.54° (042) corresponds to the scheelite monoclinic phase of BiVO4 (JCPDS card no. 140688) while peaks at 33.1° (104), 35.6° (110), 39.4° (006), 43.5° (202), and 56.7° (211) confirm the presence of rhombohedral α-Fe2O3 (JCPDS card no. 1890599) over the Zr:W-BiVO4 which matches with the literature reported values.29–31 In addition to this, the 2 theta peaks in the BFZ thin film at 29.9° (220), 35.3° (311), 43.1° (400), 46.8° (331), 62.6° (440), and 65.6° (531) confirms the formation of cubic ZnFe2O4 (JCPDS card no. 821042) over the Zr:W-BiVO4/Fe2O3 thin films.32,33 These XRD patterns with the existence of the highest intensity peaks of Zr:W-BiVO4 (B) at 28.9°, Fe2O3 (F) at 33.1°, and ZnFe2O4 (Z) at 35.3° approve the formation of the BFZ heterojunction. A higher angle shift in the highest intensity peak of BFZ in comparison with those of B, F, and Z was observed, which proposes strain in the sample (as shown in Fig. 2(b–d)).30
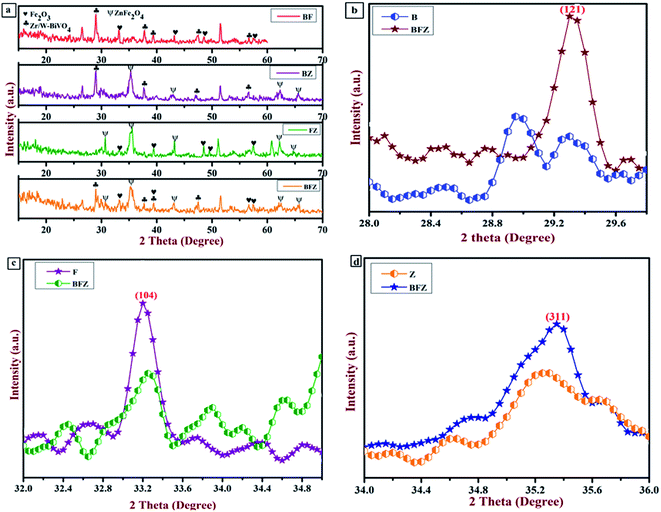 |
| Fig. 2 (a) XRD patterns of all the prepared thin films; (b) enlarged image of the highest intensity peaks of the B and BFZ samples; (c) enlarged image of highest intensity peaks of F and BFZ; (d) enlarged image of highest intensity peaks of Z and BFZ. | |
Further, the average crystallite size was also determined by Debye–Scherrer's equation and it was observed that particle size decreases in the heterojunction system in comparison with that of the other sample, which confirms the presence of lattice strain in the sample. Table 2 shows the variation in particle size in different samples.
Table 2 Variation in particle size in different samples
Sample |
Particle size (nm) |
Lattice strain |
B |
F |
Z |
B |
F |
Z |
BFZ |
27.48 |
27.07 |
10.12 |
0.0053 |
0.0047 |
0.0118 |
BZ |
31.4 |
— |
14.03 |
0.0048 |
— |
0.0085 |
FZ |
— |
28.31 |
14.08 |
— |
0.0045 |
0.0085 |
F |
— |
39.73 |
— |
— |
0.0032 |
— |
Z |
— |
— |
20.88 |
— |
— |
0.0057 |
3.2. Optical analysis: UV-visible absorption spectrum
Absorbance–wavelength spectra of the prepared thin films were investigated through a UV-vis spectrophotometer. Fig. 3(a) shows the absorption spectrum of all the thin films in the wavelength range of 300–800 nm. From Fig. 3(a), it is clear that heterojunction (BFZ) shows a prominent absorption edge at around 660 nm in comparison with those of the other samples which indicates that the sample has a strong tendency to engross a maximum number of photons and produce more charge carriers. Further, the optical band gap of all the prepared thin films was calculated using the Tauc plot, as shown in Fig. 3(b). The pristine Zr:W-BiVO4 (B) has a bandgap of 2.16 eV as shown in Fig. S1 (ESI†) while the bandgaps for F, Z, and BFZ are 2.16 eV, 2.15 eV, 2.07 eV, and 1.99 eV, respectively, confirms that the heterojunction sample demonstrated a good photoactivity in comparison with those of other samples.29,33 Table 3 shows the bandgap values of all the samples.
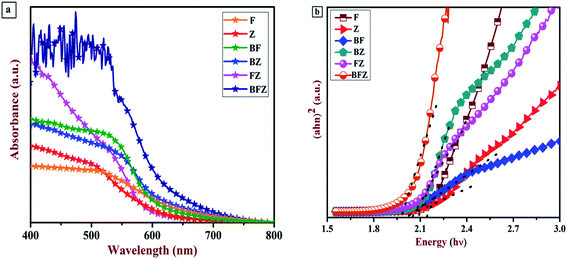 |
| Fig. 3 (a) UV-visible absorption spectrum of all the prepared thin films (b) Tauc plot of all the prepared thin films. | |
Table 3 Calculated band gap values
Sample |
Band gap (eV) |
B |
2.16 |
F |
2.10 |
Z |
1.92 |
BF |
2.01 |
BZ |
2.04 |
FZ |
2.03 |
BFZ |
1.99 |
3.3. Elemental valency and chemical compositional analysis: XPS spectra
XPS analysis was executed to examine the chemical composition and valency of each element present in the sample. Fig. 4 shows the XPS spectrum of the Zr:W-BiVO4/Fe2O3/ZnFe2O4 (BFZ) thin film. The full scan survey (Fig. 4(a)) confirms the presence of Bi, V, Zr, W, Fe, Zn, and O as the major elements while the peak at 285.2 eV is ascribed to the standard peak of a carbon atom. The presence of Bi as Bi3+, V as V5+, W as W6+ and Zr as Zr4+ was confirmed from previous work.29,34 The peak at 708.5 eV and 723.5 eV, as shown in Fig. 4(b), correspond to Fe 2p1/2 and 2p3/2, while a small satellite peak at 714.5 eV confirms the presence of Fe in the sample with a trivalent state.9,31,35 The Zn 2p XPS spectrum (Fig. 4(c)) exhibits two peaks at 1018.7 eV and 1041.9 eV, which resemble those of Zn 2p3/2 and 2p1/2. The presence of these two peaks confirms that Zn is present in the divalent state.36–39 The O 1s spectrum has two peaks at 527.2 eV and 528.8 eV, which correspond to the lattice oxygen and hydroxyl group as shown in Fig. 4(d). A shift in the peaks from the peak positions reported in the literature (i.e. in the case of Zn, 1018 eV and 1041 eV instead of 1022.1 and 1045.2 eV; in the case of O, 527 eV and 528 eV instead of 529.5 and 530.9 eV; and in the case of Fe, 723 eV and 708 eV instead of 725.2 and 711.1 eV) confirms the chemical interaction between the elements of BiVO4, ZnFe2O4, and Fe2O3, which reaffirms the formation of Fe2O3/ZnFe2O4 over the BiVO4.31
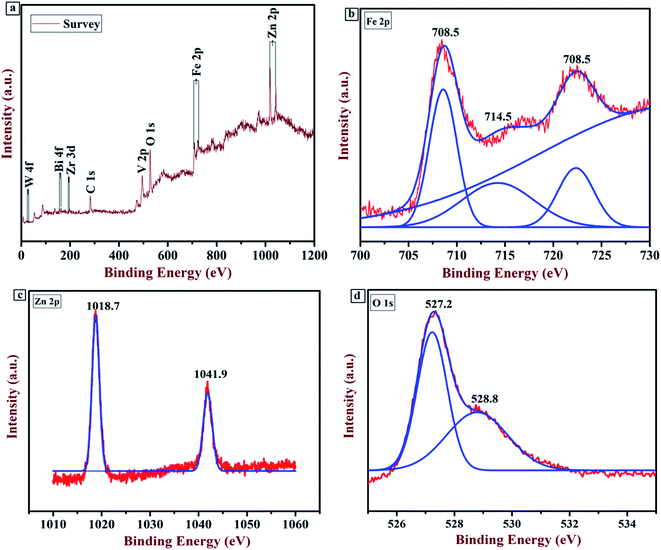 |
| Fig. 4 (a) Full scan survey of the Zr:W-BiVO4/Fe2O3/ZnFe2O4 thin film; (b) XPS spectrum of the Fe 2p element; (c) XPS spectrum of the Zn 2p element; (d) XPS spectrum of the O 1s element. | |
3.4. Morphological analysis
A morphological analysis of all the prepared thin films was executed with a JSM-7610F Field Emission Scanning Electron Microscope and the corresponding SEM images are shown in Fig. 5(a–f). A uniform and compactly grown morphology was seen in the case of BF, BZ, F, and BFZ. Spherical, round and elongated shape particles with a porous network uniformly grown over the substrate are observed in Fig. 5(d–f). The best performing triple heterojunction system (Zr:W-BiVO4/Fe2O3/ZnFe2O4) shows a root mean square (RMS) roughness of 1.51 nm, which is the highest in comparison to those of all the other samples and therefore favors a high photoelectrochemical activity (as shown in Table 4).
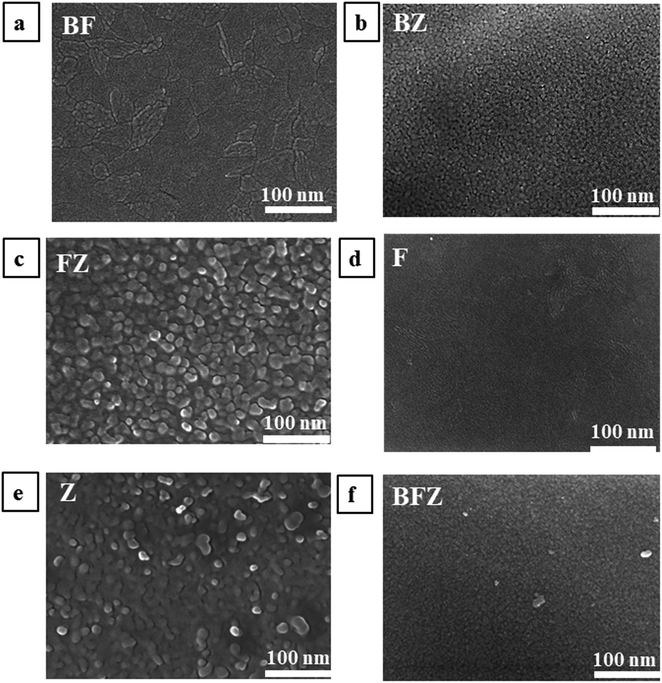 |
| Fig. 5 (a–f) FESEM images of all the prepared thin films taken at 100 nm. | |
Table 4 RMS value of all the prepared thin films
Sample |
RMS roughness (nm) |
F |
1.31 |
Z |
1.16 |
BF |
1.22 |
BZ |
1.39 |
FZ |
1.31 |
BFZ |
1.51 |
Further, EDX (Energy Dispersive X-ray Spectroscopy) analysis was also performed to confirm the elements present in the triple heterojunction thin-film BFZ. The cross-SEM image (Fig. 6(a)) of the thin film was scanned for EDX analysis from the bottom to the top direction (from B to Z) and their corresponding graphs are shown in Fig. 6(a–c). From Fig. 6(a and b) it is clear that all the elements, such as Zr, W, Bi, V, O, Fe and Zn are present in the sample and confirm the formation of heterojunction system. From Fig. 6(c) it is clear that the line scan carried out in the bottom to top direction shows that initially the amount of Bi and V increases and then decreases, which confirms that BiVO4 is present as the bottom layer and Fe is increasing towards the top layer, confirming its presence in the middle layer of Fe2O3 and top layer of ZnFe2O4. The amount of O was almost constant, which confirms that O is present throughout the sample.
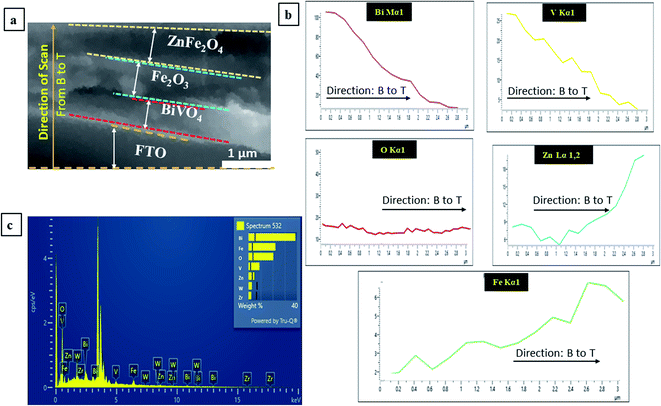 |
| Fig. 6 (a) Cross SEM image of the triple heterojunction; (b and c) EDS analysis from Bottom (B) to Top (T) (from B to Z). Here: B: BiVO4; F: Fe2O3; and Z: ZnFe2O4. | |
3.5. Photoelectrochemical analysis: current–voltage graph
The most valuable indicator for the PEC water splitting process is the conversion efficiency and linear sweep voltammetry (LSV).40 These two factors were recorded to explain the current–voltage characteristic and ABPE efficiency using three-electrode cells in a suitable electrolyte under AM 1.5G illumination. Fig. 7(a, b) and S2 (ESI†) show the current–voltage graphs (attained through linear sweep voltammetry and transient analysis) for all the prepared thin films in the potential range of −0.5 V to +1.0 V/SCE (0.5 to 2.0 V/RHE). The pristine sample here is Zr:W-BiVO4 (B), which shows the photocurrent density (PCD) of 0.13 mA cm−2 at 1.23 V/RHE (S. Saxena et al. 2021;29), and this value increased to 1.85 mA cm−2 after the deposition of Fe2O3 (F) over the B thin films. In addition to this, when ZnFe2O4 (Z) was deposited over the BF thin films, the PCD enhanced to 2.47 mA cm−2 at 1.23 V/RHE which was nineteen and two times higher than those of the B and BF samples, respectively (shown in Fig. 7(a)). This augmentation in photoresponse is due to the amended crystallinity and a properly staggered type of alignment of the B, F, and Z semiconductors. As a result, ZnFe2O4 (Z) transfers the electrons (generated through water splitting) to the core of Fe2O3 (F) and then to Zr:W-BiVO4 (B) which efficiently diminishes the recombination of photogenerated charge carriers and enhances the separation of electron–hole pairs as well as PEC performance. A chopped light study was performed in 0.1 M NaOH and the graphs are shown in Fig. 7(b). From Fig. 7(b) it is visible that the BFZ showed the minimum spikes and maximum PCD, which indicates that the sample possesses a lesser charge recombination.11,21,25,29,31 Subsequently, photogenerated holes that reach the interface participate in the water oxidation reaction. Fig. 7(c) shows the ABPE plot of all the prepared thin films. From Fig. 7(c) it is clear that BFZ possesses a maximum ABPE of 1.90%, which indicates the least charge transfer resistance and an enhanced separation of the charge carriers. Table 5 shows the photoelectrochemical parameters of all the prepared thin films recorded in a 0.1 M NaOH electrolyte.
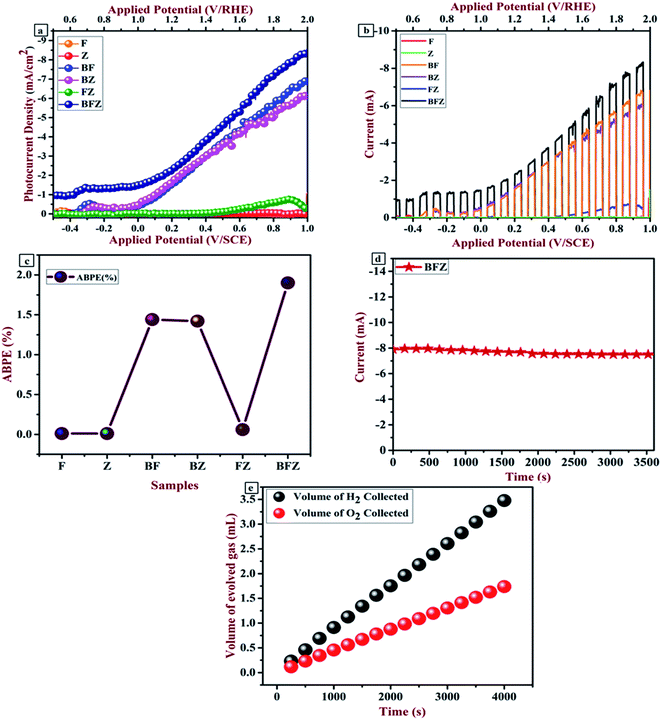 |
| Fig. 7 (a and b) Current–voltage graph obtained through LSV and transient analysis; (c) ABPE graph; (d) chronoamperometric curve; (e) evolved hydrogen and oxygen measurement. | |
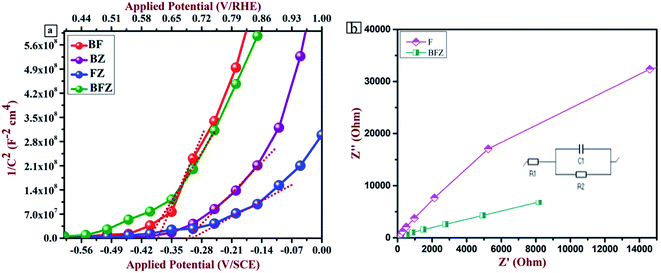 |
| Fig. 8 (a) Mott–Schottky plot for all the prepared thin films (b) Nyquist plot for the F and BFZ thin films; (Inset) fitted equivalent circuit. | |
Table 5 Photoelectrochemical parameters of all the prepared thin films recorded in a 0.1 M NaOH electrolyte
Sample |
PCD at 1.23 V/RHE (mA cm−2) |
ABPE (%) |
Flat band potential (V/SCE) |
Donor density (cm−3) |
B |
0.13 |
0.05 |
0.28 |
4.0 × 1020 |
F |
0.01 |
0.01 |
0.37 |
3.2 × 1018 |
Z |
0.01 |
0.01 |
0.27 |
3.2 × 1018 |
BF |
1.85 |
1.44 |
0.37 |
7.7 × 1018 |
BZ |
1.85 |
1.42 |
0.31 |
4.2 × 1018 |
FZ |
0.08 |
0.06 |
0.29 |
4.0 × 1018 |
BFZ |
2.47 |
1.90 |
0.39 |
8.0 × 1018 |
The stability of the best performing BFZ sample was executed in 0.1 M NaOH for 1 h (Fig. 7(d)). From Fig. 7(d) it is clear that the sample showed stability with an initial current of 7.99 mA, which decreased to 7.58 mA after continuous illumination. A graduated inverted tube was used to measure the evolution of gases (hydrogen and oxygen) using the water displacement method. The ratio of evolved H2 to O2 gas turns out to be 2
:
1, representing a faradaic efficiency of approximately unity (Fig. 7(e)).
3.6. Electrochemical analysis: Mott–Schottky and impedance analysis
Electrochemical analysis was accomplished under dark conditions using a three-electrode PEC setup to recognize the kinetics of the charge carriers across the heterojunction interface. Fig. 8(a) and S3 (ESI†) present the Mott–Schottky plots of all the prepared thin films. A Mott–Schottky plot between 1/C2 and the applied potential gives the value of the flat band potential (VFB) and the nature of the prepared thin films. From Fig. 8(a), it is clear that heterojunction BFZ possesses a more negative value of the flat band potential in comparison with those of the other samples, which indicates a better separation of the photogenerated charge carriers across the interface. In addition to this, all the thin films display a positive slope that designates the n-type nature.11,15,29,41 The flat band potential of the BFZ system is 0.39 eV/SCE which in magnitude is 0.11 eV, 0.02 eV, and 0.12 eV more negative than B, F, and Z, respectively. Further, the donor density of all the thin films was calculated and it was observed that donor density value of heterojunction BFZ is more in comparison to with those of the F and Z samples. The electrochemical active surface area (ECSA), which is involved in the water splitting reaction, was calculated from the cyclic voltammetry graphs and shown in Fig. S4 (ESI) and Table S1.† From Fig. S4(a–d) and Table S1,† it is clear that the surface area for the BFZ sample was 4.4 times and 3 times higher than those for the BZ and BF samples. This shows that BFZ exhibited efficient charge transfer kinetics at the interface.39 Table 4 shows the flat band potential and donor density value of all the prepared thin films.
To examine the transfer kinetics of charge carriers at the interface, electrochemical impedance spectroscopic analysis was performed in which the Randles–Ershler model was applied as an equivalent circuit model on the obtained Nyquist plot, which gives information about the different types of resistance, as shown in Fig. 8(b) and S5 (ESI†). In the present study, an equivalent circuit was applied on the BFZ sample as shown in Fig. 8(b) where two resistances, i.e., solution resistance (R1) and charge transfer resistance (R2), and one capacitance (C1), were observed across the interface. Generally, the arc radius in the Nyquist plot is an indicator of an improved charge transfer across the interface and it was observed that heterojunction BFZ exhibited a smaller arc radius in the Nyquist plot in comparison with those the other samples under investigation. This was due to the proper staggered type-II band alignment, which promotes the charge transfer and separation at the interface.42–44 The fitted values obtained from the equivalent circuit are shown in Table S2 (ESI†).
3.7. Mechanism of enhancement in PEC performance
The mechanism behind the improved PEC performance can be explained on the basis of the band edge positions of metal oxide semiconductors, which allow the transportation of electron and hole pairs. Two methods were adopted in order to calculate the band edge positions and then compare them with literature reported values. First is the Kubelka–Munk method in which the following equations were used to estimate the conduction band and valence band positions.
Here, X is the electronegativity, ECB and EVB are the conduction band and valence band potentials, respectively, Ec is equal to 4.5 eV (energy of a free electron at the H2 scale), and Eg is the calculated band gap (from the Tauc plot). Based on these equations, a type-II heterojunction was formed between B, F and Z. The calculated conduction and valence band positions were at 0.46, 0.33 and −0.4 eV/NHE and at 2.62, 2.43 and 1.5 eV/NHE for B, F and Z, respectively before the contact (Fig. 9(a)).11,30,33,45–47
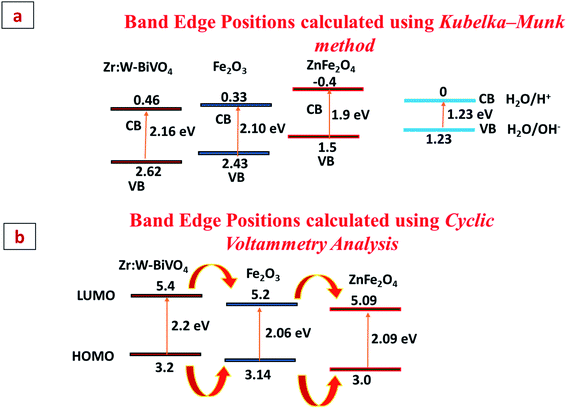 |
| Fig. 9 (a and b) Band edge positions of B, F, and Z calculated using the Kubelka–Munk method and Cyclic Voltammetry Analysis. | |
Cyclic voltammetry analysis is the second method and was also performed in order to calculate the HOMO and LUMO band edges positions and the band gap. The details and graphs are shown in Fig. 9(b) and S6 (ESI†). From the graphs it is clear that pristine BiVO4 (B) shows an EHOMO at 3.2 eV and an ELUMO at 5.4 eV, Fe2O3 (F) shows an EHOMO at 3.14 eV and an ELUMO at 5.2 eV and ZnFe2O4 (Z) shows an EHOMO at 3.0 eV and an ELUMO at 5.09 eV. The estimated band gap values for B, F and Z turn out to be 2.2 eV, 2.06 eV and 2.09 eV, respectively, which match well with the literature reported values. This shows that the sample exhibited a type-II heterojunction formation, which facilitates the charge transfer kinetics at the interface. Thus, the photogenerated electrons present in the conduction band of Z quickly move to F, then to B and then via an external circuit reach the Pt electrode, where reduction reaction takes place. Simultaneously, holes present in the valence band of B participate in the oxidation reaction and get transferred to F and then to Z, where oxidation takes place. Thus, band bending due to the appropriate band alignment would smoothen the transport of photogenerated charge carriers across the interface.48,49
4. Conclusion of the study
In the present work, we have successfully designed a n/n/n triple heterojunction photoanode consisting of Zr:W-BiVO4, Fe2O3, and ZnFe2O4 as a semiconductor metal oxide fabricated through a spray pyrolysis method. The additions of Zr and W dopants in BiVO4 increases the charge carrier density and reduces their recombination, respectively. Further, Fe2O3 and ZnFe2O4 act as a protective layer for Zr:W-BiVO4, which augments the PEC performance with a 1.90% conversion efficiency. The present study confirms that the so formed triple heterojunction not only suppresses charge recombination but also advances the water oxidation kinetics due to a better separation of the photogenerated charge carriers at the interface of the heterojunction. In addition to this, the triple heterojunction has an extended visible light absorption and maximum donor density.
Author contributions
Sakshi Saxena: conception, methodology, data curation, formal analysis investigation of data, writing-original draft; Anuradha Verma: writing-review and editing, validation, visualization; Kumari Asha: validation and resources; Neeraj Kumar Biswas: validation and resources; Anupam Srivastav: formal analysis and investigation; Vibha Rani Satsangi: conceptualization, visualization; Rohit Shrivastav: conceptualization, visualization; Sahab Dass: writing-review and editing, visualization, supervision, project administration, funding acquisition.
Conflicts of interest
There are no conflicts to declare.
Acknowledgements
Sakshi Saxena thanks the MNRE project (File No. 103/241/2015-NT), Department of Science & Technology, Ministry of Science & Technology, Govt. of India, for an Inspire Fellowship. The authors would like to thank Mr Dev Datt Pal for helping with the XPS analysis and Mr Shyamprasad Mokkapati for doing FESEM analysis.
References
- https://www.ics-hydrogen.com.
- C. Liu, H. Luo, Y. Xu, Z. Zhang, Q. Liang, W. Wang and Z. Chen, Synergistic cocatalytic effect of ultra-thin metal–organic framework and Mo-dopant for efficient photoelectrochemical water oxidation on BiVO4 photoanode, Chem. Eng. J., 2020, 384, 123333 CrossRef CAS.
- A. Eftekhari, V. J. Babu and S. Ramakrishna, Photoelectrode nanomaterials for photoelectrochemical water splitting, Int. J. Hydrogen Energy, 2017, 42(16), 11078–11109 CrossRef CAS.
- P. M. Pataniya, V. Patel and C. K. Sumesh, Electrophoretic Deposition of MoSe2–MoOx Nanosheets for Enhanced Electrocatalytic Hydrogen Evolution Reaction, ACS Appl. Energy Mater., 2021, 4(8), 7891–7899 CrossRef CAS.
- D. K. Lee, D. Lee, M. A. Lumley and K. S. Choi, Progress on ternary oxide-based photoanodes for use in photoelectrochemical cells for solar water splitting, Chem. Soc. Rev., 2019, 48(7), 2126–2157 RSC.
- X. Zhao and Z. Chen, Enhanced photoelectrochemical water splitting performance using morphology-controlled BiVO4 with W doping, Beilstein J. Nanotechnol., 2017, 8(1), 2640–2647 CrossRef PubMed.
- J. Li, J. Li, H. Yuan, W. Zhang, Z. Jiao and X. S. Zhao, Modification of BiVO4 with partially covered α-Fe2O3 spindles serving as hole-transport channels for significantly improved photoelectrochemical performance, Chem. Eng. J., 2020, 398, 125662 CrossRef CAS.
- R. Shen, L. Zhang, X. Chen, M. Jaroniec, N. Li and X. Li, Integrating 2D/2D CdS/α-Fe2O3 ultrathin bilayer Z-scheme heterojunction with metallic β-NiS nanosheet-based ohmic-junction for efficient photocatalytic H2 evolution, Appl. Catal., B, 2020, 266, 118619 CrossRef CAS.
- S. M. Tao and L. Y. Lin, Design of efficient Mn-doped α-Fe2O3/Ti-doped α-Fe2O3 homojunction for catalyzing photoelectrochemical water splitting, Int. J. Hydrogen Energy, 2020, 45(11), 6487–6499 CrossRef CAS.
- A. Banerjee, B. Mondal, A. Verma, V. R. Satsangi, R. Shrivastav, A. Dey and S. Dass, Enhancing efficiency of Fe2O3 for robust and proficient solar water splitting using a highly dispersed bioinspired catalyst, J. Catal., 2017, 352, 83–92 CrossRef CAS.
- A. Verma, A. Srivastav, S. A. Khan, V. R. Satsangi, R. Shrivastav, D. K. Avasthi and S. Dass, Enhanced photoelectrochemical response of plasmonic Au embedded BiVO4/Fe2O3 heterojunction, Phys. Chem. Chem. Phys., 2017, 19(23), 15039–15049 RSC.
- K. Asha, V. R. Satsangi, R. Shrivastav, R. Kant and S. Dass, Effect of morphology and impact of the electrode/electrolyte interface on the PEC response of Fe2O3 based systems – comparison of two preparation techniques, RSC Adv., 2020, 10(69), 42256–42266 RSC.
- O. M. Lemine, M. Bououdina, M. Sajieddine, A. M. Al-Saie, M. Shafi, A. Khatab, M. Al-Hilali and M. Henini, Synthesis, structural, magnetic and optical properties of nanocrystalline ZnFe2O4, Phys. B, 2011, 406(10), 1989–1994 CrossRef CAS.
- M. L. Baynosa, A. H. Mady, D. R. Kumar, M. S. Sayed, D. Tuma and J. J. Shim, Eco-friendly synthesis of recyclable mesoporous zinc ferrite@reduced graphene oxide nanocomposite for efficient photocatalytic dye degradation under solar radiation, J. Colloid Interface Sci., 2020, 561, 459–469 CrossRef CAS PubMed.
- J. Wang, Q. Zhang, F. Deng, X. Luo and D. D. Dionysiou, Rapid toxicity elimination of organic pollutants by the photocatalysis of environment-friendly and magnetically recoverable step-scheme SnFe2O4/ZnFe2O4 nano-heterojunctions, Chem. Eng. J., 2020, 379, 122264 CrossRef CAS.
- L. Kong, Z. Jiang, T. Xiao, L. Lu, M. O. Jones and P. P. Edwards, Exceptional visible-light-driven
photocatalytic activity over BiOBr–ZnFe2O4 heterojunctions, Chem. Commun., 2011, 47(19), 5512–5514 RSC.
- N. Guijarro, P. Bornoz, M. Prévot, X. Yu, X. Zhu, M. Johnson, X. Jeanbourquin, F. Le Formal and K. Sivula, Evaluating spinel ferrites MFe2O4 (M = Cu, Mg, Zn) as photoanodes for solar water oxidation: prospects and limitations, Sustainable Energy Fuels, 2018, 2(1), 103–117 RSC.
- D. H. Taffa, R. Dillert, A. C. Ulpe, K. C. Bauerfeind, T. Bredow, D. W. Bahnemann and M. Wark, Photoelectrochemical and theoretical investigations of spinel type ferrites (MxFe3−xO4) for water splitting: a mini-review, J. Photonics Energy, 2016, 7(1), 012009 CrossRef.
- L. C. Sonia, M. Victory and S. Phanjoubam, A Comparative Study of the Properties of Zinc Ferrite Nanoparticles Synthesized by Different Techniques for Nanofluid Preparation, Integr. Ferroelectr., 2020, 204(1), 100–111 CrossRef CAS.
- J. S. Ma, L. Y. Lin and Y. S. Chen, Facile solid-state synthesis for producing molybdenum and tungsten co-doped monoclinic BiVO4 as the photocatalyst for photoelectrochemical water oxidation, Int. J. Hydrogen Energy, 2019, 44(16), 7905–7914 CrossRef CAS.
- S. Cao, X. Yan, Z. Kang, Q. Liang, X. Liao and Y. Zhang, Band alignment engineering for improved performance and stability of ZnFe2O4 modified CdS/ZnO nanostructured photoanode for PEC water splitting, Nano Energy, 2016, 24, 25–31 CrossRef CAS.
- A. Irshad and N. Munichandraiah, Photochemical deposition of Co-Ac catalyst on ZnO nanorods for solar water oxidation, J. Electrochem. Soc., 2015, 162(4), H235 CrossRef CAS.
- A. Verma, A. Srivastav, S. Sharma, P. Badami, V. R. Satsangi, R. Shrivastav, A. M. Kannan, D. K. Avasthi and S. Dass, MWCNTs and Cu2O sensitized TiFe2O3 photoanode for improved water splitting performance, Int. J. Hydrogen Energy, 2018, 43(12), 6049–6059 CrossRef CAS.
- C. Miao, S. Ji, G. Xu, G. Liu, L. Zhang and C. Ye, Micro-nano-structured Fe2O3:Ti/ZnFe2O4 heterojunction films for water oxidation, ACS Appl. Mater. Interfaces, 2012, 4(8), 4428–4433 CrossRef CAS PubMed.
- K. J. McDonald and K. S. Choi, Synthesis and photoelectrochemical properties of Fe2O3/ZnFe2O4 composite photoanodes for use in solar water oxidation, Chem. Mater., 2011, 23(21), 4863–4869 CrossRef CAS.
- Y. L. Li, Y. Liu, Y. J. Hao, X. J. Wang, R. H. Liu and F. T. Li, Fabrication of core–shell BiVO4@Fe2O3 heterojunctions for realizing photocatalytic hydrogen evolution via conduction band elevation, Mater. Des., 2020, 187, 108379 CrossRef CAS.
- A. Srivastav, P. Kumar, A. Verma, Y. R. Smith, V. R. Satsangi, R. Shrivastav, U. V. Waghmare and S. Dass, Experimental and first-principles studies of BiVO4/BiV1−xMnxO4−y n–n+ homojunction for efficient charge carrier separation in sunlight induced water splitting, Int. J. Hydrogen Energy, 2018, 43(33), 15815–15822 CrossRef CAS.
- S. A. Salman, N. A. Bakr and R. K. Ismail, Study of the effect of annealing on optical properties of ZnFe2O4 films prepared by chemical spray pyrolysis method, International Journal of Thin Film Science and Technology, 2016, 5(1), 33–37 Search PubMed.
- S. Saxena, A. Verma, N. K. Biswas, S. A. Khan, V. R. Satsangi, R. Shrivastav and S. Dass, Zr–W Co-doping in BiVO4 – Synergistic effect in photoelectrochemical water splitting, Mater. Chem. Phys., 2021, 267, 124675 CrossRef CAS.
- G. Kaur, S. A. Khan, V. R. Satsangi, S. Dass and R. Shrivastav, Nano-hetero-structured thin films, ZnO/Ag-(α)Fe2O3, with n/n junction, as efficient photoanode for renewable hydrogen generation via photoelectrochemical water splitting, Renewable Energy, 2021, 164, 156–170 CrossRef CAS.
- J. Li, J. Li, H. Yuan, W. Zhang, Z. Jiao and X. S. Zhao, Modification of BiVO4 with partially covered α-Fe2O3 spindles serving as hole-transport channels for significantly improved photoelectrochemical performance, Chem. Eng. J., 2020, 398, 125662 CrossRef CAS.
- X. Wang, J. Feng, Z. Zhang, W. Zeng, M. Gao, Y. Lv, T. Wei, Y. Ren and Z. Fan, Pt enhanced the photo-Fenton activity of ZnFe2O4/α-Fe2O3 heterostructure synthesized via one-step hydrothermal method, J. Colloid Interface Sci., 2020, 561, 793–800 CrossRef CAS PubMed.
- X. Xu and W. Song, Synthesis and photocatalytic activity of heterojunction ZnFe2O4–BiVO4, Materials Technology, 2017, 32(8), 472–479 CrossRef CAS.
- P. Kumar, P. Sharma, R. Shrivastav, S. Dass and V. R. Satsangi, Electrodeposited zirconium-doped α-Fe2O3 thin film for photoelectrochemical water splitting, Int. J. Hydrogen Energy, 2011, 36(4), 2777–2784 CrossRef CAS.
- K. Uma, B. KrishnaKumar, G. T. Pan, T. C. Yang and J. H. Lin, Enriched silver plasmon resonance activity on the sonochemical synthesis of ZnO flowers with α-Fe2O3 as an efficient catalyst for photo-Fenton reaction and photo-oxidation of ethanol, Journal of Water Process Engineering, 2020, 34, 101089 CrossRef.
- A. A. Tahir and K. U. Wijayantha, Photoelectrochemical water splitting at nanostructured ZnFe2O4 electrodes, J. Photochem. Photobiol., A, 2010, 216(2–3), 119–125 CrossRef CAS.
- M. Wang, Y. Sun, H. Chen, Y. Zhang, X. Wu, K. Huang and S. Feng, Enhanced photoelectrochemical activity of nanostructured ZnFe2O4 thin films prepared by the electrospray technique, CrystEngComm, 2017, 19(5), 772–775 RSC.
- Y. Guo, Y. Fu, Y. Liu and S. Shen, Photoelectrochemical activity of ZnFe2O4 modified α-Fe2O3 nanorod array films, RSC Adv., 2014, 4(70), 36967–36972 RSC.
- R. Appiah-Ntiamoah, A. F. Baye and H. Kim, In Situ Electrochemical Formation of a Core–Shell ZnFe2O4@Zn(Fe)OOH Heterostructural Catalyst for Efficient Water Oxidation in Alkaline Medium, ChemElectroChem, 2020, 7(16), 3478–3486 CrossRef CAS.
- M. Chakraborty, D. Roy, A. Sharma and R. Thangavel, Post-treatment with ZnFe2O4 nanoparticles to improve photo-electrochemical performance of ZnO nanorods based photoelectrodes, Sol. Energy Mater. Sol. Cells, 2019, 200, 109975 CrossRef CAS.
- K. Asha, A. Banerjee, S. Saxena, S. A. Khan, I. Sulaniya, V. R. Satsangi, R. Shrivastav, R. Kant and S. Dass, Morphological influence of electrode/electrolyte interface towards augmenting the efficiency of photoelectrochemical water splitting – A case study on ZnO, J. Power Sources, 2019, 432, 38–47 CrossRef CAS.
- S. S. Bhat, S. A. Lee, J. M. Suh, S. P. Hong and H. W. Jang, Triple planar heterojunction of SnO2/WO3/BiVO4 with enhanced photoelectrochemical performance under front illumination, Appl. Sci., 2018, 8(10), 1765 CrossRef.
- N. A. Mohamed, J. Safaei, A. F. Ismail, M. N. Khalid, M. F. Jailani, M. F. Noh, N. A. Arzaee, D. Zhou, J. S. Sagu and M. A. Teridi, Boosting photocatalytic activities of BiVO4 by creation of g-C3N4/ZnO@BiVO4 heterojunction, Mater. Res. Bull., 2020, 125, 110779 CrossRef CAS.
- S. Bai, J. Han, K. Zhang, J. Sun, J. Guo, R. Luo, D. Li and A. Chen, Triadic layered double hydroxide modified semiconductor heterojunction for PEC water splitting, ACS Sustainable Chem. Eng., 2020, 8(10), 4076–4084 CrossRef CAS.
- T. W. Kim and K. S. Choi, Improving stability and photoelectrochemical performance of BiVO4 photoanodes in basic media by adding a ZnFe2O4 layer, J. Phys. Chem. Lett., 2016, 7(3), 447–451 CrossRef CAS PubMed.
- T. B. Nguyen and R. A. Doong, Heterostructured ZnFe2O4/TiO2 nanocomposites with a highly recyclable visible-light-response for bisphenol A degradation, RSC Adv., 2017, 7(79), 50006–50016 RSC.
- K. Xiong, K. Wang, L. Chen, X. Wang, Q. Fan, J. Courtois, Y. Liu, X. Tuo and M. Yan, Heterostructured ZnFe2O4/Fe2TiO5/TiO2 Composite Nanotube Arrays with an Improved Photocatalysis Degradation Efficiency Under Simulated Sunlight Irradiation, Nano-Micro Lett., 2018, 10(1), 17 CrossRef PubMed.
- A. Shafiee, M. M. Salleh and M. Yahaya, Determination of HOMO and LUMO of [6,6]-phenyl C61-butyric acid 3-ethylthiophene ester and poly(3-octyl-thiophene-2,5-diyl) through voltammetry characterization, Sains Malays., 2011, 40(2), 173–176 CAS.
- L. Leonat, G. Sbarcea and I. V. Branzoi, Cyclic voltammetry for energy levels estimation of organic materials, UPB Scientific Bulletin, Series B: Chemistry and Materials Science, 2013, 75(3), 111–118 CAS.
|
This journal is © The Royal Society of Chemistry 2022 |
Click here to see how this site uses Cookies. View our privacy policy here.