DOI:
10.1039/D2RA04676H
(Paper)
RSC Adv., 2022,
12, 33516-33524
A promising 1D Cd-based hybrid perovskite-type for white-light emission with high-color-rendering index†
Received
27th July 2022
, Accepted 14th November 2022
First published on 23rd November 2022
Abstract
A one dimensional (1D) perovskite-type (C6H7NBr)3[CdBr5] (abbreviated 4-BAPC) was synthesized by slow evaporation at room temperature (RT). 4-BAPC crystalizes in the monoclinic system with the space group P21/c. The 1D inorganic chains are formed by corner sharing CdBr6 octahedra. Thermal measurement shows that 4-BAPC is stable up to 190 °C. Optical characterization demonstrates that the grown crystal is an indirect bandgap material with a bandgap value of 3.93 eV, which is consistent with theoretical calculations. The electronic structure, calculated using density functional theory, reveals that the valence band originates from a combination of Br-4p orbitals and Cd-4d orbitals, whereas the conduction band originates from the Cd-5s orbitals. The photoluminescence spectroscopy shows that the obtained material exhibits a broad-band white light emission with extra-high CRI of 98 under λexc = 380 nm. This emission is mainly resulting from the self-trapped exciton recombinations within the inorganic CdBr6 octahedron, and the fluorescence within the organic conjugated ammonium salt.
Introduction
Nowadays, solid-state lighting technology is very important in our daily life because of its incredible applications for human development.1–3 To produce white-light in light-emitting diodes (LEDs), three main processes are used. The first process consists of coating a mixture of red, green, and blue (RGB) phosphors in an ultraviolet LED.4,5 The second process requires using a mixture of RGB LEDs to create WL,6,7 and the third process consists of coating a yellow phosphor in a blue LED.8,9 Despite their advantages, each process has its own cons such as low efficiency, low color rendering, and discontinuity in the photoluminescence spectrum.10–12 Hybrid organic–inorganic perovskite materials (HOIPM) have gained much attention after being reported as semiconductors in 1990.13–16 After the release of (CH3NH3)[PbI3]17 in 2009 as a high performance semiconductor solar absorber in the first perovskite solar cell with great potential and low cost, HOIPM becomes one of the most important research topics in the field of material chemistry during the past decade. Owing to their wide band gap and low dimensional, HOIPM show an extreme performance in diverse optoelectronic applications such as LEDs,18–20 lasers21 and photodetectors.22 In the last ten years, researchers reported that white light emission was easily observed in two-dimensional HOIPM such as the (EDBE)[PbBr4],23 (N-MEDA)[PbBr4],24 (C6H11NH3)2[PbBr4]25 and (C3H8N6)[PbBr4].26 White-light emission occurs when the self-trapping mechanism, resulting from strong electron–phonon coupling, is observed inside a distorted inorganic lattice.23,27,28 The performance of the white light emitter depends on two critical parameters, the color rendering index (CRI)29–31 and CIE chromaticity coordinates.32 The CRI describes the ability of the light source to render the colors of various objects with comparison to the natural sunlight.12,31 In general, the commercial LEDs show a CRI value about 80 which is considered acceptable for human eye-friendly applications. However, high-level industrial lighting applications, such as cinematography, surgery and museum galleries require an ultra-high CRI greater than 90.33,34 Previous researches on structure-properties correlation in low-dimensional metal halides structures demonstrated that 1D perovskites with large band gap could exhibit superior photoluminescence (PL) properties.35–40 In 1D-hybrid metal halide systems, the 1D assembly of the six coordinated octahedra present in general four different types of connection: face-sharing, edge-sharing, corner-sharing and a mixed connection modes.41 In this study, we report a new 1D Cd-based hybrid perovskite-type (C6H7NBr)3[CdBr5]. This material demonstrates a high ability to emit a bright white-light with a CIE chromaticity coordinate of (0.30, 0.32), an ultrahigh CRI of 98, and a correlated color temperature (CCT) of 6812 K. The experimental optical analysis and the theoretical calculation, using the density functional theory (DFT), prove that our compound belongs to large gap semiconductors and that it is suitable for optoelectronic applications as white-light emitter.
Experimental section
Synthesis
All chemical products were purchased from Sigma-Aldrich and used without any further purification. An aqueous solution containing CdBr2·4H2O (1 mmol, 0.34 g), 4-bromoaniline (3 mmol, 0.55 g) and 0.2 ml of hydrobromic acid was heated at 80 °C and stirred using a magnetic stirrer for 20 minutes. The slow evaporation of the final solution at RT during two weeks yielded yellow platelets crystals of (C6H7NBr)3[CdBr5], suitable for structure determination using X-ray diffraction in high yield (75%). The optical microscope image in Fig. 1(a) shows the morphology of the crystals.
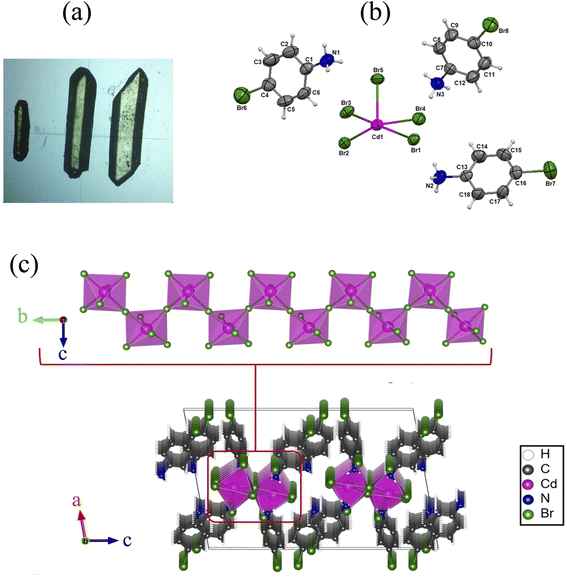 |
| Fig. 1 (a) Optical microscopy image of the microcrystals (×50), (b) asymmetric unit of 4-BAPC, (b) 1D inorganic infinite chain and (c) packing framework 4-BAPC. | |
X-ray crystallographic study
Single-crystal X-ray diffraction (XRD) data were collected using an APEX II, Bruker-AXS diffractometer with the Mo Kα radiation (λ = 0.71073 Å) at RT (293 K). The structure was solved with direct method and refined by full-matrix least-squares method based on F2 using the SHELXT software.42 The organic parts were found by the utilization of a successive Fourier calculation using the same software. All hydrogen atoms bonded to C and N atoms were geometrically assigned with a distance C–H = 0.97 Å and N–H = 0.89 Å. The crystallographic data and structure refinement conditions of (C6H7NBr)3[CdBr5] are summarized in Table 1.
Thermal and vibrational studies
The thermal analyses of (C6H7NBr)3[CdBr5] were performed on raw powders with a TGA/DTA ‘SETSYS Evolution’ (Pt crucibles, Al2O3 as a reference) under N2 flow (100 ml min−1). The thermograms were collected on 11.5 mg of the samples in a maximum temperature of 600 °C (heating speed of 5 °C min−1).
The IR spectrum was recorded in the frequency range 4000–450 cm−1 at room temperature using a PerkinElmer FT-IR 1000 spectrometer. Sample was prepared as pellets with KBr as a dispersant.
Optical and photoluminescence measurements
The UV-vis diffuse reflectance spectrum of the 4-BAPC was performed at room temperature using a Varian Cary 5000 UV-vis-NIR spectrophotometer in the wavelength range between 200 and 800 nm. The PL spectra were collected using a Horiba FluoroMax 4 spectrometer at room temperature under different excitation wavelengths.
Calculation method
Density functional theory (DFT) calculation was conducted using the QUANTUM ESPRESSO package.43,44 The generalized gradient approximation (GGA) as proposed by Perdew–Burke–Ernzerhof45 was employed with projector-augmented wave (PAW) pseudopotentials with cutoff energies of 70 Ry for the plane-wave basis set and 420 Ry for the charge density. The Brillouin zone was sampled with a 3 × 6 × 2 Monkhorst–Pack mesh for structural optimization and self-consistent calculation. A structural relaxation was conducted for the atomic positions with a force on each atom smaller than 0.01 eV Å−1 and a convergence threshold on total energy for ionic minimization less than 10−6 Ry. The energy convergence for each self-consistency step was 10−6 Ry. A Gaussian smearing of 0.01 Ry was used.
w = 1/[σ2F02 + (0.0247P)2 + 4.8948P] |
Table 1 Crystal data and structure for 4-BAPC
Crystal data |
Chemical formula |
(C6H7NBr)3[CdBr5] |
Mr |
1031.06 |
Crystal system, space group |
Monoclinic, P21/c |
Temperature (K) |
293 |
a, b, c (Å) |
15.4709 (14), 8.1826 (10), 23.466 (4) |
β (°) |
99.59 (1) |
V (A3) |
2929.1 (7) |
Z |
4 |
Radiation type |
Mo Kα radiation, λ = 0.71073 Å |
μ (mm−1) |
11.67 mm−1 |
Crystal size (mm) |
0.23 × 0.13 × 0.10 |
Data collection |
Diffractometer |
APEXII, Bruker-AXS |
Absorption correction |
Multi-scan |
Tmin, Tmax |
0.170, 0.353 |
No. of measured, independent and observed [I > 2σ(I)]reflections |
64 272, 6738, 4209 |
Rint |
0.087 |
θmax, θmin (°) |
27.5, 2.0 |
Refinement |
R[F2 > 2σ(F2)], wR(F2), S |
0.036, 0.091, 1.10 |
No. of reflections |
6738 |
No. of parameters |
274 |
No. of restraints |
0 |
H-Atom treatment |
H-Atom parameters constrained |
Δρmax, Δρmin (e Å−3) |
0.68, −1.14 |
where P = (F02 + 2Fc2)/3.
Results and discussion
Structure description
The single-crystal XRD analyses show that the 4-bromoanilinium tetrabromocadmate crystallizes in the monoclinic system with the centrosymmetric space group P21/c. All the cell parameters are listed in Table S1.† The asymmetric unit is formed of three 4-bromoanilinium cations (C6H7NBr)+ and one pyramidal square based [CdBr5]3− anion (Fig. 1(a)). The Cd atoms are octahedrally coordinated by six Br atoms, the length of the Cd–Br bonds vary between 2.729(8) and 2.98(7) Å and the Br–Cd–Br angles are ranging from 81.36(2)° to 173.44(2)° as shown in Table S1.† The inorganic framework is constituted by infinite helical chains, formed with corner chairing CdBr6 octahedra, extending along b-axis, with the presence of two bridging and four terminal bromide atoms (Fig. 1(b)) similar to 1D perovskite-type structures such as (NAEP)2Pb2Cl10 H2O39 and [NH3(CH2)2Br]3CdBr5.46
Fig. S1† shows that the packing diagram is constituted by infinite corner-sharing chains linked to the organic cations by N–H⋯Br hydrogen bonds. The organic cations are symmetrically different, resulting in the existence of two different types of N–H⋯Br hydrogen bonds. The first type, shown in Fig. S1(b),† is generated by two organic cations with the nitrogen atoms N2 and N3 connected directly to the inorganic chains and occurred between two terminal bromide atoms of the same octahedra and one birding bromide atom of another octahedron from the same chain (N–H⋯Br distance equal to 2.678 Å). The second type of hydrogen bonds (Fig. S1(c)†) connects the inorganic chains to each other and it is generated by the third cation with nitrogen atom N1 between two adjacent octahedrons from two different chains by their terminal bromide atoms (N–H⋯Br distance equals to 2.570 Å). The difference in distances between the two hydrogen bond types probably implies that the shorter is slightly stronger. The hydrogen bond parameters are listed in Table S2.†
On the other hand, the analysis of the organic molecules Hirshfeld surfaces shows that they are connected together via C⋯Br (Fig. S2†) and C⋯H (Fig. S3†) short contacts. The existence of red spots on the surfaces of the organic rings mapped with shape index reveals the existence of Br⋯π and C–H⋯π interactions between the different organic moieties.
To determine the degree of the structure distortion we opt to define the parameter Δd, which indicate the deviations of the lengths of the Cd–Br distances within the CdBr6 octahedra.
where
d is the average Cd–Br bond length (2.8266 Å) and
dn refers to the individual Cd–Br bonds in the octahedra. While the calculated Δ
d gives a value of 1.059 × 10
−3, proving that CdBr
6 octahedron is highly distorted in comparison with other octahedron in perovskite structures such as (2cepiH)CdCl
3 (Δ
d = 2.58 × 10
−4),
40 (mpz)
2PbBr
10 (Δ
d = 4.5 × 10
−4),
37 and α-(DMEN)PbBr
4 (Δ
d = 1.7 × 10
−3).
47 It should be noted that the formation of hydrogen bonds between the organic and inorganic groups is in agreement with the different degrees of distortion affecting the adjacent cadmium octahedra (
Fig. 2(b)).
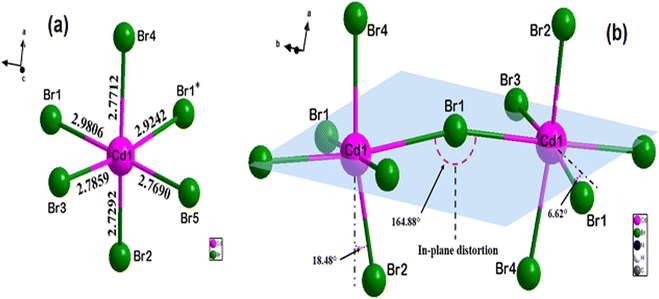 |
| Fig. 2 Geometric description of the inorganic framework: (a) distorted CdBr6 octahedron with different Cd–Br bond lengths and (b) distortion in adjacent octahedra. | |
Vibrational and thermal analysis
IR spectrum. Fig. S4† presents the infrared spectrum of the studied perovskite. We note the presence of two principal bands appearing around 2926 and 3039 cm−1, which are generally attributed to the classical stretching vibrations of N–H related to the NH3+ groups, confirming the protonation of the 4-bromoaniline molecules. The bands appearing between 2574 and 480 cm−1 are attributed to the aromatic C–C and C–H vibrational modes of the organic groups.
Thermal analysis
The TG curve (Fig. S5†) shows that the 4-BAPC is stable up to 190 °C. Above this temperature, a weight loss is detected around 368 °C. During this process, the 4-BAPC loses 50% of its initial weight. This loss is assigned to the degradation of two organic molecules and the volatilization of two bromide ions, in the form of (C6H6BrN)·HBr. The theoretical weight loss is 49.05% of the total mass. By correlating the structure of this material with the results of the thermogravimetric analysis, we can conclude that the departure of two organic cations in the first step confirms the difference in the strength of the hydrogen bonds that ensure the cohesion between these organic groups and the inorganic part. Indeed, the two cations (C6H7BrN)+ linked via hydrogen bonds to a single chain probably decompose at the first time, while the third cation of the remaining compound appears to be more stable due to its connection with two different chains, thus playing the role of a junction bridge between them.
Semiconducting properties
In order to predict the electronic properties of the 4-BAPC, the solid-state diffuse reflectance spectrum of the synthesized compound was collected at room temperature. The absorption spectrum (Fig. 3(a)), was obtained from the reflectance spectrum using the Kubelka–Munk (K–M) function:
where R is the reflectance, K is the K–M absorption coefficient, and S is the scattering coefficient.48 The 4-BAPC presents an absorption peak in the ultraviolet region with a wavelength around 270 nm. As presented in Fig. 3(a) the optical absorbance spectrum shows an intense absorption peak around 4.24 eV (292 nm), which is attributed to the electronic transition from the valence to the conduction band.28,49,50 The weak bands observed between 2.5 and 4 eV are probably assigned to the excitons confined into the [CdBr5]∞3− chains and to the charge transfer between the organic and the inorganic species. The band gap energies of 4-BAPC can be estimated from the Tauc plots as a function of the photon energy (hν). This method is often used to estimate an optical band gap of a given semiconductor with parabolic bands at the Fermi energy level. By means of a slight fitting of direct and indirect Tauc plots (Fig. 3(b) and (c)), the direct and indirect band gap energies were found to be Egd = 4.27 eV and Egind = 3.93 eV, respectively. The obtained energy gap values are reasonable and close to others values of hybrid organic–inorganic Cd-based compounds from literature, such as the ferroelectric perovskite (3-pyrrolinium)[CdCl3]51 (4-5eV), the semiconductor (C5H9NH3)4[CdBr6] (4.58 eV), and (C5H12N)[CdCl3] (4.65 eV).49,52
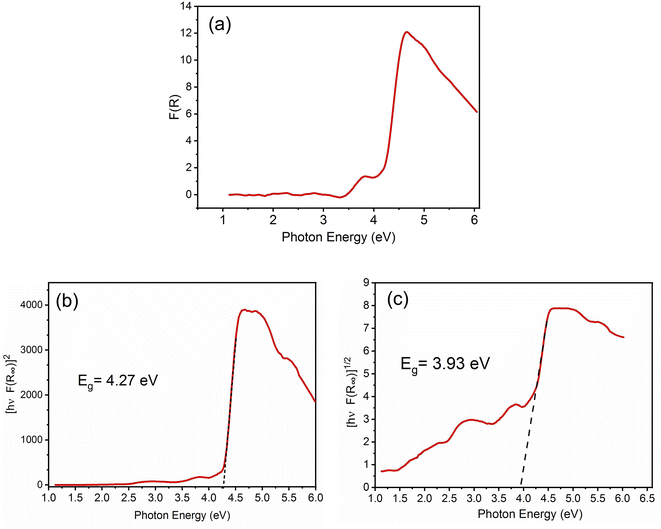 |
| Fig. 3 (a) Kubelka–Munk absorption spectrum, (b) Tauc plot for direct gap and (c) Tauc plot for indirect gap (h is the Planck constant, ν is the vibrational frequency of the oscillator, and F(R∞) is the K–M absorption coefficient). | |
To gain more insight into the electronic properties of this new perovskite, we have performed DFT calculations. Fig. 4(a) and (b) present the electronic band structure and the density of states (DOS), respectively. It is clear that our material has an indirect band gap of 3.16 eV, with the valence band maximum (VBM) located between C2 and Y2 points, and the conduction band minimum (CBM) at the Y2 valley of the Brillouin zone. The direct band gap located at Y2 point has a value close to that of the indirect band gap (only a few meV larger) as shown in Fig. S6 of the ESI.† The calculated band gap values are less than those measured optically (4.24 and 3.93 eV for the direct and indirect band gap, respectively), this is due to the fact that the GGA method underestimates band gaps.53 An analysis of the partial density of states (PDOS) displayed in Fig. 4(c), reveals that the VBM originates from a combination of Br-4p orbitals and Cd-4d orbitals, whereas the CBM originates from the Cd-5s orbital.
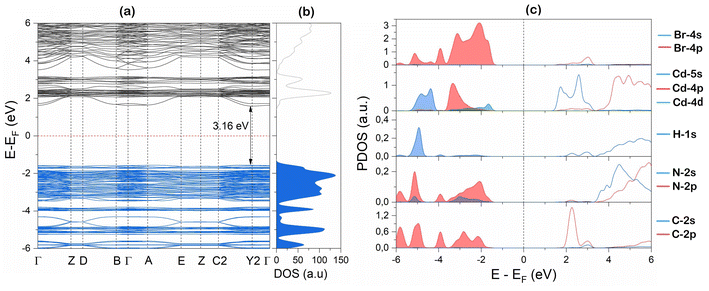 |
| Fig. 4 DFT simulation of 4-BAPC (a) band structure, (b) total density of states (DOS), and (c) partial density of states (PDOS). | |
Photoluminescence
The photoluminescence of 4-BAPC was explored to investigate the photo-physical properties. Here, the material was excited under different energies to study its photoluminescent properties. It is well known that the sub-gap excitation (EExc < Eg) of Cd-based materials improves the self-trapping mechanism within the CdBrx clusters, and generates furthermore to the narrow excitonic emission, a broad-band covering the visible region leading to white light emission.54,55 Hence, we use in this section two different excitation energies; the first one is above the gap value and the second one is below the gap value in order to enhance the emission through STE mechanism. In our case, the excitation of the material with photon energy higher than the band-gap, of 4.92 eV (250 nm), shows that the synthesized compound exhibits broad emission in the UV-vis region at room temperature (Fig. 5).
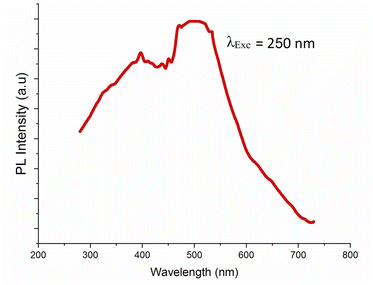 |
| Fig. 5 PL spectrum of the (4-BAPC) under 250 nm. | |
Under sub-gap excitation of 380 nm, the PL spectrum (Fig. 6(a)) shows that the 4-BAPC exhibits a wide emission band spanning the majority of the visible range. The main emission spectrum is similar to that observed in 1D and 0D Cd-based hybrid materials, such as (2cepiH)CdCl3 (2cepi = 1-(2-chloroethyl)piperidine),40 (C5H9–NH3)4CdBr6,49 and (HMEDA)CdBr4 (HMEDA = hexamethylenediamine).50 Fig. 6(b) shows the map of the chromaticity coordinates (x,y) of the emitted light in the CIE 1931 chromaticity diagram. The chromaticity coordinates of the light rays emitted by our material under 380 nm are (0.30, 0.32), very close to that of the ideal sun light (0.33, 0.33). The corresponding calculated CRI and CCT are 98 and 6812 K, respectively, indicating that the synthesized compound emit a cold white light with an extra high CRI.
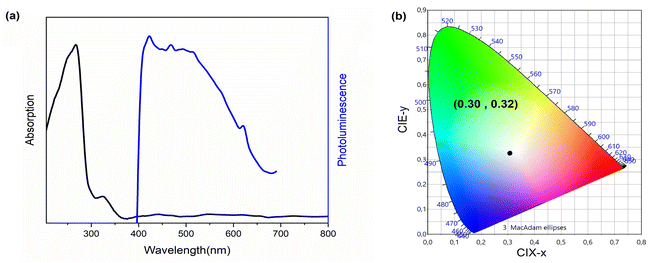 |
| Fig. 6 (a) Absorption and emission spectra (λexc = 380 nm) of 4-BAPC and (b) CIE coordinates of the same compound. | |
Recently, several research papers proved the pertinent role of the π-conjugated organic cations in the emissive behaviors of hybrid materials. Depending on the molecular packing mode, the emission from organic cations can be phosphorescence or fluorescence in nature and then, the photoluminescence performances of hybrid materials can be tailored by modifying supramolecular assembly modes.56 Hence, to scrutinize the emission origin within the 4-BAPC structure and to more understand the effect of the organic species (C6H7BrN)+ on the photoluminescence properties, we recorded the emission spectra of the pure organic 4-bromoaniline (C6H6BrN) and the (C6H7BrN)+·Br− salt under excitation wavelength of 200 nm (Fig. 7). The emission spectra illustrate that they display an intense broad-band emission spanning the whole UV-vis region. The revealing of the white light emission from C6H6BrN and (C6H7BrN)+·Br− proves the emissive properties of the organic moieties.
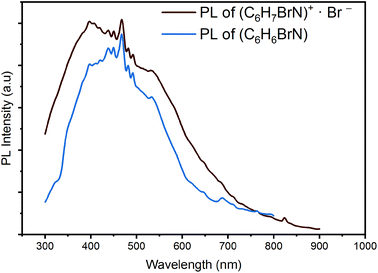 |
| Fig. 7 PL spectra of the organic molecule C6H6BrN and (C6H7BrN)+·Br− ammonium salt (λExc = 200 nm). | |
In fact, recent researches on organic fluorescent materials show that intermolecular non-covalent interactions, such as anion⋯π, C–H⋯π and N–H⋯π, enhance the excited state intramolecular proton transfer (ESIPT) mechanism leading to an ultrafast fluorescence resulting from the transitions from different excited singlet states
to the excited states
.57–59 On the other hand, Br−⋯H+ hydrogen interactions can inhibit the molecular vibrational movement which improves the luminescence properties.60 As shown in the structure description section, the XRD results demonstrate the existence of C–H⋯π and Br⋯π interactions between the different organic molecules (see Fig. S2 and S3†). As the structure of the pure organic molecule C6H6BrN was previously reported,61 we analyse the Hirshfeld surface of the C6H6BrN molecule (Fig. S7†). This analysis shows that the organic molecules are linked through N–H⋯π and Br⋯π interactions. Hence, the fluorescence from the pure organic C6H6BrN is stabilized through the ESIPT mechanism which remain observed within the 4-BAPC structure, where the N–H⋯π contacts were replaced by the C–H⋯π ones. Therefore, the emission of the white light from our material is due to the organic fluorescence and the STE within the inorganic sub-lattice.
To analyze the emission processes inside the crystal, a Gaussian deconvolution of the luminescence spectrum of the salt was realized (Fig. S8†). The best multi-peak fitting of the PL spectrum shows that the white light emission of the salt results from four overlapped fluorescence bands observed at 311, 383, 497 and 623 nm which originate from multi-excitonic transitions from excited singlet states
to the excited singlet state
. The comparison between the photoluminescence spectra of the (C6H7BrN)+·Br− salt (Fig. S8†) and that of (C6H7NBr)3[CdBr5], Fig. 8(a), shows that the luminescence bands observed for the salt are also observed in the emission spectrum of the hybrid material. The appearance of two extra bands “EF” at 456 and “ES” at 575 nm results from the free exciton's emission and the self-trapped exciton's emission within the inorganic cluster, respectively. The proposed PL mechanism within 4-BAPC is presented in Fig. 8(b). To study the effect the variation of the excitation energy on the luminescence properties of the material, the PL response of 4-BAPC was recorded under different excitation energies. As shown in Fig. 9, increasing the excitation energy from 320 to 420 nm, the broad band WLE from the material still observed.
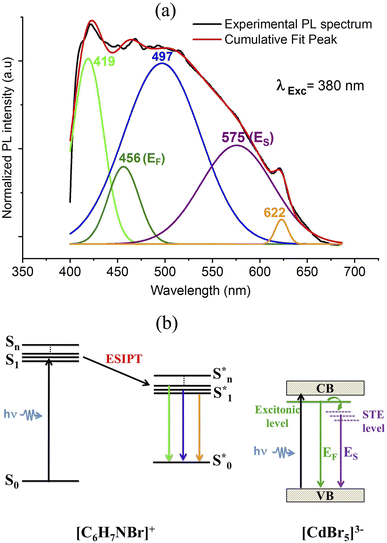 |
| Fig. 8 Multi-peaks fitting of the PL spectrum of 4-BAPC (a) with the proposed emission diagram under subgap excitation of 380 nm (b). | |
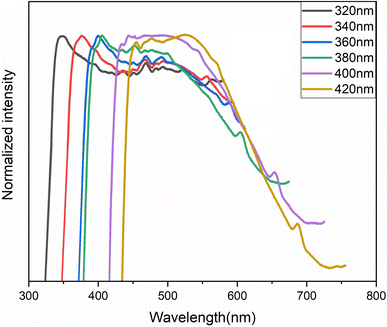 |
| Fig. 9 Emission spectra of 4-BAPC with different wavelengths excitation at room temperature. | |
The lifetime measurements were recorded and presented in Fig. 10. The time resolved PL decay of three emission peaks were recorded under excitation wavelength of 365 nm. The measured average lifetimes at 406, 586 and 666 nm were obtained by fitting the experimental data using a bi-exponential decay function and they are found to be 2, 1.97 and 2.3 ns, respectively. The fast decay of the emission bands confirms the fluorescence nature of 4-BAPC.
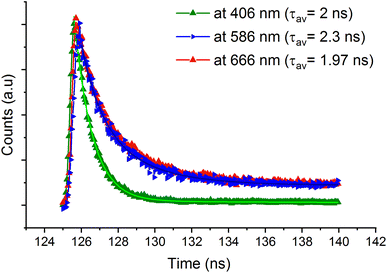 |
| Fig. 10 The time resolved PL decays recorded under 365 nm. | |
Conclusion
In summary, we report a new lead-free hybrid organic–inorganic perovskite-type, 4-BAPC, with 1D chains formed with corner-sharing CdBr6 octahedra. The UV-vis absorption analysis shows that the 4-BAPC presents a large band gap energy which makes it suitable for optoelectronic applications. The calculated band structure reveals that the valence band originates from a combination of Br-4p orbitals and Cd-4d orbitals, whereas the conduction band originates from the Cd-5s orbital. The synthesized molecular crystal exhibit an efficient white-light emission characterized by an ultra-high CRI up to 98 and a CIE chromaticity coordinates of (0.30, 0.32) with a CCT of 6812 K. This emission results from the excitonic transition within the inorganic framework and from the fluorescence of the organic molecules.
Conflicts of interest
There are no conflicts to declare.
Acknowledgements
The authors extend their appreciation to the Deanship of Scientific Research at Imam Mohammad Ibn Saud Islamic University (IMSIU) for funding and supporting this work through Research Partnership Program no RP-21-09-71.
References
- Y. L. Chang and Z. H. Lu, J. Disp. Technol., 2013, 9, 459–468 CAS.
- T. H. Kim, W. Wang and Q. Li, Front. Chem. Sci. Eng., 2012, 6, 13–26 CrossRef CAS.
- S. W. Sanderson and K. L. Simons, Resour. Policy, 2014, 43, 1730–1746 CrossRef.
- I. Speier and M. Salsbury, Sixth Int. Conf. Solid State Light., 2006, 6337, 63371F Search PubMed.
- Y. Ohno, Fourth Int. Conf. Solid State Light., 2004, 5530, 88 Search PubMed.
- S. Muthu and J. Gaines, Conf. Rec. - IAS Annu. Meet., 2003, 1, 515–522 Search PubMed.
- S. Muthu, F. J. P. Schuurmans and M. D. Pashley, IEEE J. Sel. Top. Quantum Electron., 2002, 8, 333–338 CrossRef CAS.
- K. H. Lee and S. W. R. Lee, Proc. Electron. Packag. Technol. Conf., 4th, 2006, 379–384 Search PubMed.
- J. Yum, S.-Y. Seo, S. Lee and Y.-E. Sung, J. Electrochem. Soc., 2003, 150, H47 CrossRef CAS.
- M. Pan, B. Bin Du, Y. X. Zhu, M. Q. Yue, Z. W. Wei and C. Y. Su, Chem.–Eur. J., 2016, 22, 2440–2451 CrossRef CAS PubMed.
- D. Chen, Z. Wan, Y. Zhou, X. Zhou, Y. Yu, J. Zhong, M. Ding and Z. Ji, ACS Appl. Mater. Interfaces, 2015, 7, 19484–19493 CrossRef CAS PubMed.
- S. Ye, F. Xiao, Y. X. Pan, Y. Y. Ma and Q. Y. Zhang, Mater. Sci. Eng., R, 2010, 71, 1–34 CrossRef.
- D. B. Mitzi, S. Wang, C. A. Feild, C. A. Chess and A. M. Guloy, Science, 1995, 267, 1473–1476 CrossRef CAS PubMed.
- R. J. Stead, M. A. Barradas, D. P. Mikhailidis, M. E. Hodson, J. C. Batten and P. Dandona, Platelet function in patients with cystic fibrosis, 1986, vol. 25 Search PubMed.
- D. B. Mitzi, C. A. Feild, Z. Schlesinger and R. B. Laibowitz, J. Solid State Chem., 1995, 159–163 CrossRef CAS.
- D. B. Mitzi, C. A. Feild, W. T. A. Harrison and A. M. Guloy, Nature, 1994, 369, 467–469 CrossRef CAS.
- K. A., T. K., S. Y. and M. T., J. Am. Chem. Soc., 2009, 131, 6050–6051 CrossRef PubMed.
- M. Yuan, L. N. Quan, R. Comin, G. Walters, R. Sabatini, O. Voznyy, S. Hoogland, Y. Zhao, E. M. Beauregard, P. Kanjanaboos, Z. Lu, D. H. Kim and E. H. Sargent, Nat. Nanotechnol., 2016, 11, 872–877 CrossRef CAS PubMed.
- G. Li, Z. K. Tan, D. Di, M. L. Lai, L. Jiang, J. H. W. Lim, R. H. Friend and N. C. Greenham, Nano Lett., 2015, 15, 2640–2644 CrossRef CAS PubMed.
- H. Cho, S. H. Jeong, M. H. Park, Y. H. Kim, C. Wolf, C. L. Lee, J. H. Heo, A. Sadhanala, N. S. Myoung, S. Yoo, S. H. Im, R. H. Friend and T. W. Lee, Science, 2015, 350, 1222–1225 CrossRef CAS PubMed.
- G. Xing, N. Mathews, S. S. Lim, N. Yantara, X. Liu, D. Sabba, M. Grätzel, S. Mhaisalkar and T. C. Sum, Nat. Mater., 2014, 13, 476–480 CrossRef CAS PubMed.
- F. X. Liang, L. Liang, X. Y. Zhao, L. B. Luo, Y. H. Liu, X. W. Tong, Z. X. Zhang and J. C. A. Huang, Adv. Opt. Mater., 2019, 7, 1–9 Search PubMed.
- E. R. Dohner, A. Jaffe, L. R. Bradshaw and H. I. Karunadasa, J. Am. Chem. Soc., 2014, 136, 13154–13157 CrossRef CAS PubMed.
- E. R. Dohner, E. T. Hoke and H. I. Karunadasa, J. Am. Chem. Soc., 2014, 136, 1718–1721 CrossRef CAS PubMed.
- A. Yangui, D. Garrot, J. S. Lauret, A. Lusson, G. Bouchez, E. Deleporte, S. Pillet, E. E. Bendeif, M. Castro, S. Triki, Y. Abid and K. Boukheddaden, J. Phys. Chem. C, 2015, 119, 23638–23647 CrossRef CAS.
- R. Msalmi, S. Elleuch, B. Hamdi, E. Radicchi, A. Tozri, H. Naïli and M. R. Berber, New J. Chem., 2021, 45, 20850–20859 RSC.
- K. Thirumal, W. K. Chong, W. Xie, R. Ganguly, S. K. Muduli, M. Sherburne, M. Asta, S. Mhaisalkar, T. C. Sum, H. Sen Soo and N. Mathews, Chem. Mater., 2017, 29, 3947–3953 CrossRef CAS.
- A. Yangui, S. Pillet, E. E. Bendeif, A. Lusson, S. Triki, Y. Abid and K. Boukheddaden, ACS Photonics, 2018, 5, 1599–1611 CrossRef CAS.
- Y.-J. Tung, M. M. Lu, M. S. Weaver, M. Hack and J. J. Brown, Org. Light. Mater. Devices VII, 2004, 5214, 114 CAS.
- Q. Dai, C. E. Duty and M. Z. Hu, Small, 2010, 6, 1577–1588 CrossRef CAS PubMed.
- J. J. Zhang, R. Hu, X. J. Yu, B. Xie and X. B. Luo, Opt. Laser Technol., 2017, 88, 161–165 CrossRef CAS.
- N. Sandor and H. Yaguchi, Color Res. Appl., 2004, 29, 168 CrossRef.
- M. S. Rea and J. P. Freyssinier, Color Res. Appl., 2010, 35, 401–409 CrossRef.
- G. He, J. Xu and H. Yan, AIP Adv., 2011, 1, 032160 CrossRef.
- Y. Peng, Y. Yao, L. Li, Z. Wu, S. Wang and J. Luo, J. Mater. Chem. C, 2018, 6, 6033–6037 RSC.
- H. Barkaoui, H. Abid, S. Zelewski, J. Urban, M. Baranowski, A. Mlayah, S. Triki, P. Plochocka and Y. Abid, Adv. Opt. Mater., 2019, 7, 1–9 RSC.
- L. Mao, P. Guo, M. Kepenekian, I. Hadar, C. Katan, J. Even, R. D. Schaller, C. C. Stoumpos and M. G. Kanatzidis, J. Am. Chem. Soc., 2018, 140, 13078–13088 CrossRef CAS PubMed.
- W. F. Zhang, W. J. Pan, T. Xu, R. Y. Song, Y. Y. Zhao, C. Y. Yue and X. W. Lei, Inorg. Chem., 2020, 59, 14085–14092 CrossRef CAS PubMed.
- D. Li, W. Wu, S. Wang, X. Zhang, L. Li, Y. Yao, Y. Peng and J. Luo, J. Mater. Chem. C, 2020, 8, 6710–6714 RSC.
- Z. Qi, Y. Chen, Y. Guo, X. Yang, F. Q. Zhang, G. Zhou and X. M. Zhang, J. Mater. Chem. C, 2021, 9, 88–94 RSC.
- D. Cortecchia, J. Yin, A. Petrozza and C. Soci, Mater. Chem. C 10.1039/C9TC01036J.
- G. Sheldrick, Acta Crystallogr., 2015, 71, 3–8 CrossRef PubMed.
- J. Enkovaara, C. Rostgaard and J. J. Mortensen, Phys. Condens. Matter, 2017, 29, 465901 CrossRef PubMed.
- P. Giannozzi, S. Baroni, N. Bonini, M. Calandra, R. Car, C. Cavazzoni, D. Ceresoli, G. L. Chiarotti, M. Cococcioni, I. Dabo, A. Dal Corso, S. De Gironcoli, S. Fabris, G. Fratesi, R. Gebauer, U. Gerstmann, C. Gougoussis, A. Kokalj, M. Lazzeri, L. Martin-Samos, N. Marzari, F. Mauri, R. Mazzarello, S. Paolini, A. Pasquarello, L. Paulatto, C. Sbraccia, S. Scandolo, G. Sclauzero, A. P. Seitsonen, A. Smogunov, P. Umari and R. M. Wentzcovitch, J. Phys.: Condens. Matter, 2009, 21, 395502 CrossRef PubMed.
- J. P. Perdew, K. Burke and M. Ernzerhof, Phys. Rev. Lett., 1996, 77, 3865–3868 CrossRef CAS PubMed.
- H. P. Chen, Z. X. Wang, C. Chen, Y. Lu, Z. Yin, X. F. Sun and D. W. Fu, Dalton Trans., 2017, 46, 4711–4716 RSC.
- M. Lingling, W. Yilei, S. Constantinos C, R. W. Michael and G. K. Mercouri, J. Am. Chem. Soc., 2017, 139, 5210–5215 CrossRef PubMed.
- G. Kortüm, W. Braun and G. Herzog, Angew. Chem., 1963, 75, 653–661 CrossRef.
- W. Sasa, L. Li, Z. Sun, C. Ji, S. Liu, Z. Wu, S. Zhao, M. Hong and J. Luo, J. Mater. Chem. C, 2017, 5, 4731–4735 RSC.
- X. W. Sun, C. He, W. L. Liu, M. J. Pan, W. J. Dong, L. F. Chen and G. Lei, Chem.–Asian J., 2020, 15, 3050–3058 CrossRef PubMed.
- M. Ferroelectricity, H. Ye, Y. Zhang and R. Xiong, Angew. Chem., Int. Ed., 2014, 1–7 Search PubMed.
- E. C. C. Baly, Annu. Rep. Prog. Chem., 1913, 10, 28–53 RSC.
- L. Sun, W. Zhou, Y. Liang, L. Liu and P. Wu, Comput. Mater. Sci., 2016, 117, 489–495 CrossRef CAS.
- R. Msalmi, S. Elleuch, B. Hamdi, W. Abd El-Fattah, N. Ben Hamadi and H. Naili, RSC Adv., 2022, 12, 10431 RSC.
- R. Roccanova, M. Houck, A. Yangui, D. Han, H. Shi, Y. Wu, D. T. Glatzhofer, D. R. Powell, S. Chen, H. Fourati, A. Lusson, K. Boukheddaden, M. Du and B. Saparov, ACS Omega, 2018, 3, 18791–18802 CrossRef CAS PubMed.
- Z. Huang and X. Ma, Cell Rep. Phys. Sci., 2020, 1, 100167 CrossRef CAS.
- V. S. Padalkar and S. Seki, Chem. Soc. Rev., 2016, 45, 169–202 RSC.
- A. Shahraki, A. Ebrahimi, S. Rezazadeh and R. Behazin, Mol. Syst. Des. Eng., 2021, 6, 66–79 RSC.
- H. C. Joshi and L. Antonov, Molecules, 2021, 26, 1475 CrossRef CAS PubMed.
- S. Feng, Q. Huang, S. Yang, Z. Lin and Q. Ling, Chem. Sci., 2021, 14451–14458 RSC.
- A. Dey, R. K. R. Jetti, R. Boese and G. R. Desiraju, CrystEngComm, 2003, 5, 248–252 RSC.
|
This journal is © The Royal Society of Chemistry 2022 |
Click here to see how this site uses Cookies. View our privacy policy here.