DOI:
10.1039/D2RA04874D
(Paper)
RSC Adv., 2022,
12, 27839-27845
Zinc ion detection using a benzothiazole-based highly selective fluorescence “turn-on” chemosensor and its real-time application†
Received
5th August 2022
, Accepted 19th September 2022
First published on 29th September 2022
Abstract
A new photochromic fluorescence chemosensor was devised and effectively synthesized using benzothiazole and imidazopyridine derivatives. A “turn-on” fluorescence sensor BIPP for Zn2+ detection was developed and has a quick response, excellent sensitivity, and remarkable selectivity over other metal ions. When Zn2+ was added to the BIPP solution, a new strong fluorescence emission peak at 542 nm formed with a considerable increase in intensity. The fluorescence color of the BIPP solution changed from blue to bright green. The binding ratio 8
:
2 was found between BIPP and Zn2+ by the results of Job's plot, HRMS and 1H-NMR. The detection limit (LOD) of BIPP towards Zn2+ was determined to be 2.36 × 10−8, which is remarkably low. The ability to detect Zn2+ in real water samples demonstrates that BIPP may also be used in environmental systems. Additionally, BIPP can be used to measure Zn2+ levels in living cells.
1. Introduction
The development of chemosensors capable of detecting a wide variety of metal ions and anions has generated considerable attention due to their wide range of biological, therapeutic, and industrial applications.1–6 Among them, the zinc (Zn2+) ion is the second most common transition metal element in humans after iron, making it a critical mineral in biological processes. The benefits of zinc include support for the immune system, regulation of hormones and digestion, and many proteins and enzymes that consist of Zn2+-ions as a cofactor have been discovered.7–9 The elevated levels of zinc ions, on the other hand, have been related to a variety of neurological diseases, including arteriosclerosis,7 Parkinson's disease,10,11 and epilepsy.12,13 On the contrary, zinc shortage in the body may cause health issues including growth retardation, diarrhoea, poor wound healing, and dermatitis. The Zn2+ ion concentration in human excretion varies widely depending on a variety of factors, including biological, habitual, and environmental factors. Therefore, zinc content assessment in real water and biological samples may be a unique diagnostic tool in medicinal, environmental, and industrial applications. The body's metabolic markers for zinc ions make it simple to identify Zn2+ ions in many investigations (proteins, enzymes, and carbohydrates, and so on).14 Due to the complexity and triviality of most analytical methods used to detect Zn2+ ions, researchers have shifted their focus to fluorescence detection technology, essential for environmental and biological system analysis.
Colorimetric and fluorescent chemosensors based on organic emissive molecules promise innovative solutions for sensor applications, since they are simple to use and can quickly provide chemical information.15–27 A plethora of fluorescent chemosensors28–37 has been developed to date based on different fluorophores, such as purine-based Schiff base,38 rhodamine,39 aminomethylnaphthol,40 coumarin,41 and quinoline containing acetyl hydrazone.42 One frequent obstacle in searching for Zn2+-detection ligands is their apparent inability to distinguish Zn2+ from its chemically identical neighbour, Cd2+. Further limiting issues, including multi-step synthesis, employment of a metal–ligand ensemble for recognition, poor chemical stability, and insignificant detection limitations, have been frequently found in a large percentage of scientific publications that assess the effectiveness of prospective Zn2+ sensing molecules.
Benzothiazole43–51 and imidazole52–58 derivatives have been intensively implemented in the development of optical chemosensors due to their easy functionalization and photostability. They are particularly effective in chelating metal ions because of sulfur, oxygen, and nitrogen atoms with lone-pair of electrons. We hypothesized that the imidazole conjugated benzothiazole moiety might be used to detect Zn2+ in an aqueous solution since they are expected to bind metal ions strongly and have excellent photophysical properties.
As part of our research interest in ion recognition,59–63 in this work, we utilize benzothiazole-linked imidazole as a fluorophore to design and construct a “turn-on” Zn2+ fluorescence probe. The structure of the synthesized probe forms an excellent conjugated system through benzothiazole and imidazole pyridine and results in a strong photon-induced electron transfer (PET) action, which interferes with the probe's fluorescence emission. This novel Zn2+ sensor is promising due to its rapid response to Zn2+, excellent sensing capability and simplicity of preparation. The sensor, named BIPP in this manuscript, was used to demonstrate the applicability by detecting Zn2+ in water, test strips, and live cells, providing a dependable method for detecting Zn2+ in environmental and biological systems.
2. Experimental section
2.1. Materials and methods
Di(pyridin-2-yl) methanone, all the other chemicals and solvents were obtained from commercial suppliers (Sigma Aldrich and TCI chemicals) and used without further purification. 1H and 13C NMR analyses were performed in Bruker 400 MHz and 100 MHz instruments. NMR spectra were stated in parts per million (ppm, δ). UV-Vis absorption spectra were monitored with the help of Hitachi-2910 spectrophotometers, and emission spectra were monitored with Hitachi F-7000 spectrophotometers. High-Resolution Mass Spectrometer (HRMS) data were collected using a Joel GC Mate II GC-Mass Spectrometer instrument.
2.2. Synthesis and characterization
2.2.1 Preparation of 2-(benzo[d]thiazol-2-yl) phenol (BTP). The reactants 2-amino thiophenol (1.1 ml, 1 mmol) and salicylaldehyde (1 ml, 1 mmol) were dissolved in 15 ml of EtOH. This reaction mixture was kept under reflux for 5 hours. The progress of the reaction was examined by TLC. A white colour solid had been obtained (1.6 g, 84% yield). 1H NMR (400 MHz, DMSO-d6): 6.991–7.7.010 (t, 1H, J = 7.6 Hz), 7.097–7.118 (d, 1H, J = 8.4 Hz), 7.392–7.413 (q, 2H, 2xArCH, J = 8.4 Hz), 7.510–7.512 (t, 1H, J = 1.6 Hz), 8.050–8.070 (d, 1H, J = 8 Hz), 8.109–8.129 (t, 2H, J = 8 Hz), 11.652 (s, 1H, OH). 13C NMR (DMSO-d6, 100 MHz): δ 117.48, 118.70, 120.20, 122.43, 122.57, 125.57, 126.92, 129.00, 132.94, 134.61, 151.91, 156.82, 165.92.
2.2.2 Synthesis of 3-(benzo[d]thiazol-2-yl)-2-hydroxybenzaldehyde (BTH). The Compound BTP (2 g, 1 mmol) was dissolved in acetic acid (20 ml), followed by the addition of hexamethylenetetramine (2.19 g, 2 mmol). The reaction mixture was refluxed for 5 hours. The completion of the reaction was inspected by TLC. After that, the reaction mixture was allowed to cool in room temperature, and it was poured into crushed ice with vigorous stirring. The filtered solid product was purified by column chromatography.64,65 (1.2 g, 53%). 1H NMR (400 MHz, DMSO-d6): δ 7.218–7.237 (t, 1H, J = 7.6 Hz), 7.505–7.523 (t, 1H, J = 7.2 Hz), 7.588–7.606 (t, 1H, J = 7.2 Hz), 7.919–7.938 (d, 1H, J = 7.6 Hz), 8.125–8.145 (d, 1H, J = 8 Hz), 8.207–8.227 (d, 1H, J = 8 Hz), 8.385–8.404 (d, 1H, J = 7.6 Hz), 10.378 (s, 1H, OH), 13.006 (s, 1H, CHO). 13C NMR (DMSO-d6, 100 MHz): δ 119.71, 120.71, 122.79, 122.82, 123.94, 126.39, 127.46, 133.43, 133.94, 135.58, 151.43, 159.50, 165.79, 192.27.
2.2.3 2-(benzo[d]thiazol-2-yl)-6-(1-(pyridin-2-yl) imidazo[1,5-a] pyridin-3-yl) phenol (BIPP). In a round bottom flask a mixture of BTH (1 g, 3.9 mmol), di(pyridin-2-yl) methanone (0.481 mg, 2.6 mmol) ammonium acetate (1.05 g, 13 mmol) was charged in acetic acid. The suspension was allowed to reflux for 6 h and the reaction was tracked by TLC. Once the reaction was completed the reaction mixture was cooled to room temperature, then poured into crushed ice, stirred for 30 minutes and filtered. The yellow solid was obtained, and then washed with diethyl ether. Yield 60%; mp 270–273 °C; 1H NMR (400 MHz, CDCl3) δ ppm 6.936–6.918 (t, 1H, J = 7.2 Hz), 7.224–7.208 (m, 1H, J = 6.4 Hz), 7.318–7.304 (t, 2H, J = 5.6 Hz), 7.553–7.533 (m, 1H, J = 8 Hz), 7.622–7.601 (m, 1H, J = 8.4 Hz), 7.872–7.869 (q, 1H, J = 8 Hz), 7.976–7.956 (t, 1H, J = 8 Hz), 8.157–8.139 (q, 2H, J = 7.2 Hz), 8.247–8.226 (m, 3H, J = 8.4 Hz), 8.648–8.636 (t, 2H, J = 4.8 Hz), 7.57–7.55 (d, 1H, J = 8 Hz), 13.151 (s, 1H). 13C NMR (100 MHz, CDCl3) δ ppm 114.36,118.22, 118.63, 120.11, 120.75, 121.39, 122.63, 122.90, 125.23, 126.50, 127.59, 130.48, 130.63, 133.31,135.31, 151.42, 155.42, 168.23. HRMS (ESI) m/z: [MH+] calcd for C34H24N2OS 421.1055; Found: 421.1134.
2.3. Stock solution preparation
The stock solution of BIPP (1 × 10−3 M) was made by dissolving in the mixture of ACN
:
H2O (8
:
2). Aqueous solutions of different nitrate metal cation salts at a concentration of 1 × 10−3 M were made in double-distilled water medium. The fluorescence and absorption were observed in ACN
:
H2O (8
:
2) solution. UV-vis absorption and emission were measured using (1 × 10−6 M) BIPP and various metals (1 × 10−6 M).
3. Results and discussion
3.1. Synthesis and characterization of the probe BIPP
The benzothiazole unit is particularly advantageous as a component of excited-state intramolecular proton transfer (ESIPT) probe because it is optically stable, environmentally sensitive, and exhibits brilliant fluorescence with a significant Stokes shift. Similarly, the imidazo[1,5-a]pyridine nucleus is well-known for its photophysical characteristics in the literature.66–68 On the basis of these observations, new chemosensor 2-(benzo[d]thiazol-2-yl)-6-(1-(pyridin-2-yl) imidazo[1,5-a] pyridin-3-yl) phenol (BIPP) was developed by incorporating imidazopyridine into the benzothiazole motifs. The imidazopyridine unit is electron-rich and serves as an electron donor, quenching the benzothiazole unit's fluorescence through the photoinduced electron transfer (PET) mechanism. Once BIPP coordinates selectively to Zn2+, the disruption of the PET process and subsequent generation of the “push–pull” system triggers fluorescence enhancement. Straightforward cyclization of methanones with 3-(benzo[d]thiazol-2-yl)-2-hydroxybenzaldehyde in the presence of ammonium acetate was used to accomplish the synthetic process (Scheme 1). The reaction is simple, demanding just one step and no need for catalysts or Lewis acids with high sensitivity. The structural identification of the sensor BIPP was verified by 1H NMR, 13C NMR, and HRMS spectra analysis (Fig. S1–S7†).
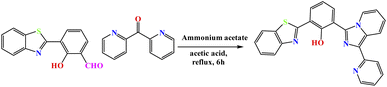 |
| Scheme 1 The synthetic route of sensor BIPP. | |
3.2. BIPP as a sensory probe for Zn2+
The UV-vis spectral measurements were carried out in a binary mixture of ACN and H2O (8
:
2, v/v). BIPP receptor absorption spectra display two λmax bands at 292 nm and 327 nm, caused by π–π* and n–π* transitions, respectively (Fig. S8†). Adding Zn2+ to the receptor BIPP caused the band at 327 nm to vanish, and a new band at 393 nm emerged. Whereas, the absorption peak at 292 nm remained undisturbed. On the other hand, there were no significant changes in the absorbance spectra of receptor BIPP with other examined cations such as Ag+, Na+, K+, Li+, Ba2+, Ca2+, Cd2+, Ce4+, Co2+, Cu2+, Fe2+, Hg2+, Mg2+, Mn2+, Ni2+, Pb2+, Sr2+, Al3+, Cr3+, Fe3+ and Ru3+, indicating that receptor BIPP binds preferentially to Zn2+ ions while in the ground state Zn2+ causes electronic perturbation in BIPP (Fig. 1a). The progressive addition of Zn2+ was used to perform UV-vis titration of the receptor BIPP in ACN
:
H2O (8
:
2, v/v) solution (Fig. 1b). Following the progressive addition of Zn2+, the disappearance of the receptors absorption band at 327 nm and the appearance of a new peak at 393 nm were gradual, with the eventual appearance of the isosbestic point at 372 nm. An interaction between Zn2+ and receptor BIPP results in creating isosbestic points, which suggest that new species has been formed.
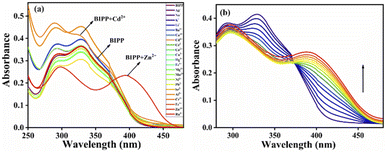 |
| Fig. 1 (a) UV-Vis absorption spectra of sensor BIPP (1 × 10−6 M) towards 5 equiv. of various anions in ACN/H2O (8 : 2, v/v) solution; (b) absorption spectra of sensor BIPP (1 × 10−6 M) in ACN/H2O (8 : 2, v/v) solution upon the addition of various concentrations of Zn2+ ions (0–2 equivalents). | |
A fluorescence technique was used to examine the receptor's metal-ion detecting characteristics in similar mixed solvent solutions by adding the nitrate salt of several series of different cations including Ag+, Na+, K+, Li+, Ba2+, Ca2+, Cd2+, Ce4+, Co2+, Cu2+, Fe2+, Hg2+, Mg2+, Mn2+, Ni2+, Pb2+, Sr2+, Al3+, Cr3+, Fe3+ and Ru3+ (Fig. 2a). Due to the prevailing photo-induced electron transfer (PET) mechanism at the excited state, the receptor BIPP exhibited faint fluorescence after being stimulated at 489 nm. The receptor BIPP demonstrated an extremely selective ‘Off–On’ fluorescence increase at 473 nm in the presence of Zn2+ among the different metal ions tested (5 equivalents). Additionally, the fluorescence titration of receptor BIPP was carried out by adding Zn2+ ions progressively over time (Fig. 2b). The fluorescence band at 489 nm of the receptor BIPP increased with increasing Zn2+ concentration. Notably, Zn2+ concentration alters the fluorescence intensity of BIPP linearly between 0.1 and 1 μM. An excellent linear correlation between the relative fluorescence intensities (I/I0 at 473 nm) was found using the derived calibration curve for the concentration range of 0.1 to 1 μM of Zn2+ ion (Fig. S9†). We found the linear equation is y = 7.05697x + 0.62667, where x is the concentration of Zn2+ and y is the absorption intensity at 473 nm. For the sensor BIPP, the detection limit was determined by taking the slope of the linear fit graph and the standard deviation from the blank BIPP fluorescence spectra, as shown in eqn (1) (see ESI†). The LOD was 2.36 × 10−8 M, far below the WHO's recommended limit of 76.5 μM.69 It was discovered that the achieved detection limit was significantly lower than that reported in current literature (Table S1†). With the addition of Zn2+, BIPP's solution has changed color under ultraviolet light from blue to bright green (Fig. 2c). The quantum yield of BIPP, on the other hand, increased dramatically when Zn2+ was added (Φ = 0.68).
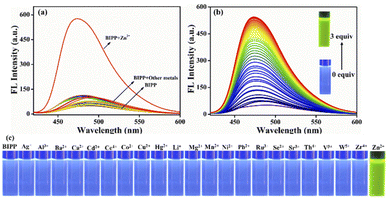 |
| Fig. 2 (a) Fluorescence spectra of BIPP (2.0 M) after the addition of 21 metal ions (5 equiv.) in an ACN/H2O (8 : 2, v/v) solution. (b) Fluorescent spectra of BIPP (2.0 M) in ACN/H2O (8 : 2, v/v) solution with different Zn2+ ions concentrations (0–3 equivalent). (c) Fluorescence bottle images of BIPP in solution with various metal ions (1 × 10−3 M). | |
3.3. Effects of competitive metal ions, pH, and time response
A chemosensor must, among other things, respond specifically to target analytes while avoiding cross-sensitivity. Hence, the competitive experiment was conducted by taking fluorescence spectrum readings of receptor BIPP in 1 equivalent with Zn2+ combined with other interfering cations, as stated above (Fig. 3a). No substantial difference was observed between Zn2+ solutions with and without other cations in the fluorescence spectra of receptor BIPP. These findings suggest that other potentially competing cations do not affect receptor BIPP's identification of Zn2+. Due to its higher sensitivity for Zn2+, receptor BIPP may be used in real-time sample analysis. The chemosensor BIPP and BIPP–Zn2+ system was examined for the effects of pH variation. The chemosensor BIPP's fluorescence pattern in the pH range of 2.0 to 12.0 is depicted in (Fig. 3b). In the pH range of 6.0 to 12.0, the fluorescence spectra of the chemosensor BIPP showed no noticeable change. When the pH falls below 6, the additional supply of H+ protonates the pyridyl and imidazole nitrogen, increasing the fluorescence. Additionally, adjusting the pH from 2.0 to 6.0 alters the chemosensor BIPP's fluorescence response to Zn2+ ions. At lower pH levels, the protonation of the pyridyl and imidazole nitrogen hinders complexation with Zn2+ because there are no lone pair of electrons available. This suggests that when the pH decreases from 6 to 2, the fluorescence of BIPP + Zn2+ increases. The interaction of Zn2+ ions with the receptor BIPP occurred quickly during the first 10 seconds, as shown (Fig. S10†), and there was no significant change in fluorescence intensity after that, even when the time period was prolonged to 60 seconds. These results show that the chemosensor BIPP has quick coordination with Zn2+, which is desired.
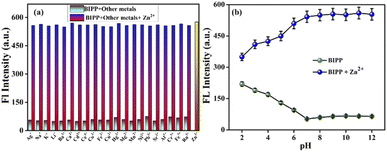 |
| Fig. 3 (a) Competitive assays for the fluorescence responses of BIPP (1 μM) in the presence of Zn2+ ions (1 μM) and other competing metal ions (5 μM) in ACN/H2O (8 : 2, v/v) solution. (b) In the absence and presence of Zn2+, the influence of pH on the fluorescence intensity of BIPP in ACN/HEPES buffer (8 : 2) (pH 2.0–12.0) solutions. | |
4. Sensing mechanism
Imidazole-nitrogen and pyridyl-nitrogen are thought to interact with Zn2+ ions to create a BIPP-Zn complex in solution. When the receptor BIPP and the Zn2+ ion interact, the ICT process speeds up, bringing the lowest excited state closer to the highest ground state.70,71 To evaluate the manner of complexation directly, 1H NMR titrations of BIPP with different doses of Zn2+ in DMSO-d6 were performed (Fig. 4). In 1H NMR, the pure BIPP displayed a hydroxyl (–OH) signal at 13.14 ppm, and the protons flanking the pyridyl nitrogen appeared at 8.73 ppm (Ha) and 8.22 ppm (Hb). But with the increasing concentration of zinc ions, the signal of Ha and Hb showed a up field shifted from 8.73 ppm to 8.69 ppm and 8.22 ppm to 8.12 ppm. The proton (Hc) located para to the phenolic –OH felt an upfield shift from 7.33 ppm to 7.22 ppm. The proton marked as Hd also shifted to the upfield region from 6.89 to 6.57 ppm. In addition, the –OH signal shifted from 13.14 ppm to 12.38.
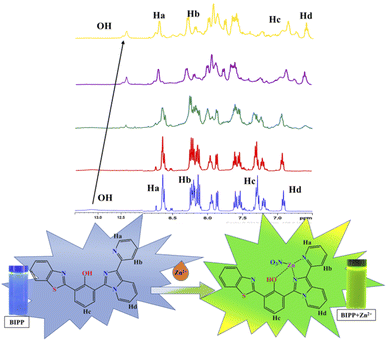 |
| Fig. 4 1H NMR spectra of BIPP (400 MHz, DMSO-d6, TMS) and the complex BIPP–Zn2+ following the addition of Zn2+ ions. | |
This confirms that the complexation happens in the region of imidazole and pyridyl ring. Furthermore, HRMS study confirmed the presence of a BIPP–Zn2+ complex (Fig. S11†). Positive ion mass analysis indicated that the peak at 546.0209 (m/z) was ascribed to [BIPP + Zn2+ + NO3]+ (calcd, 546.0214). Job plot analysis was used to comprehend the means of binding between the sensor BIPP and Zn2+ (Fig. S12†). It was discovered that the binding stoichiometry between BIPP and Zn2+ was 1
:
1. The Benesi–Hildebrand plot of BIPP derived from emissive titration data was used to determine the binding constant, which was determined to be 6.7189 × 105 M−1 between the receptor BIPP and the Zn2+ ion (Fig. S13†).72,73 In the FT-IR spectrum of BIPP, the OH functional group was attributed at 3383.48 cm−1. The OH peak did not vanish when BIPP interacted with Zn2+, indicating that the Zn2+ ion was combining with only pyridyl nitrogen (Fig. S14†).
In addition, DFT calculations using the Gaussian 09 programme were employed to support the sensing mechanism. The frontier molecular orbital (FMO) analysis of both chemosensors and the target analyte were optimised at the B3LYP/6-31G (d) level of theoretical analysis.74 Fig. 5 depicts the HOMO–LUMO surfaces of the BIPP chemosensor. The HOMO was mainly localized on the imidazo pyridine of the chemosensor units, and the LUMO orbital was dispersed over benzothiazole which accounts for the extended π conjugation. The HOMO orbital of the chemosensor units was mostly centred on the imidazo pyridine, while the LUMO orbital was spread across benzothiazole, which accounts for the extended conjugation. Similarly, in BIPP + Zn2+ complex, the electronic density of HOMO orbital is dispersed on the imidazo pyridine and on benzothiazole in LUMO orbitals. It is important to note that in the LUMO of the BIPP + Zn2+ complex, the electron density is completely orientated towards benzothiazole, making the PET process unfeasible. In addition, the energy gaps (ΔE) between HOMOs and LUMOs of BIPP and BIPP + Zn2+ were computed to be 3.82 eV and 3.17 eV, respectively. In the presence of Zn2+, the decreased energy gap was responsible for the red-shift spectral transformation in the absorption and emission spectra of BIPP.
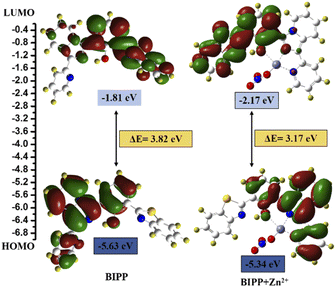 |
| Fig. 5 DFT calculated LUMO and HOMO diagrams of BIPP and BIPP + Zn2+. Application of the proposed method for analysis of environmental water samples. | |
5. Practical sample applications
To further evaluate the use of BIPP for determining the Zn2+ content of actual water samples, recovery tests were conducted using real water samples from tap and drinking water in the Vellore Institute of Technology (VIT), India campus using the previously described approach.69 For Zn2+ detection, BIPP sensor yielded recoveries of 97.5% to 103.5%, as shown Table 1. The results showed that BIPP could be used to detect Zn2+ in real samples with high accuracy, indicating certain practical value of this probe. These results show that the competing metal ions in the water sample have no effect on the accuracy of the sensor we constructed to monitor Zn2+ ions.
Table 1 Determination of Zn2+ ion in regular water samples
Water samples studied |
Concentration of standard Zn2+ ion spiked (μM) |
Concentration of Zn2+ ion found (μM) (n = 3) |
Recovery of Zn2+ ions added (%) |
Tap water |
2.0 |
1.95 |
97.5 |
4.0 |
3.97 |
99.25 |
6.0 |
6.03 |
100.5 |
Drinking water |
2.0 |
2.02 |
101 |
4.0 |
4.11 |
102.75 |
6.0 |
6.21 |
103.5 |
6. Cell imaging studies
Zn2+ ion detection in live cells using fluorescence microscopy was established by conducting fluorescence imaging studies. E. coli cells have been used to emulate living cells in this experiment.75 For 30 minutes at 37 °C, E. coli cells were incubated with BIPP in PBS with 1% DMSO as a co-solvent and imaged. After 30 minutes, the cells were infused with 2 μM Zn2+ and imaged once again. Incubation of E. coli cells with BIPP did not cause a spike in intracellular fluorescence, as was anticipated (Fig. 6b). On the other hand, when BIPP-loaded E. coli cells were incubated with Zn2+, a bright greenish-yellow fluorescence was seen (Fig. 6d). Thus, it is clear that the fluorescence increase in live cells was caused by the complexation of BIPP with Zn2+. In cytotoxicity experiments (Fig. S15†), BIPP was shown to be highly biocompatible and non-toxic to live cells. Thus, the appearance of significant fluorescence inside the intracellular region suggests that the probe BIPP is capable of detecting Zn2+ ions in living cells.
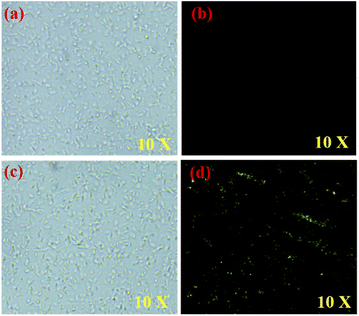 |
| Fig. 6 This picture shows bright-field and fluorescence images of E. coli cells that have been exposed to BIPP and Zn2+. (a and c) E. coli cells were incubated separately with BIPP and BIPP + Zn2+ (b) E. coli cells were treated with BIPP (d) E. coli cells were treated with BIPP and Zn2+. | |
7. Conclusion
Finally, we have devised and synthesized a new and simple benzothiazole conjugated imidazopyridine BIPP with tunable absorbance and fluorescence to detect Zn2+ ions. An increase in fluorescence intensity of BIPP at 473 nm resulted from the addition of Zn2+, which disturbed the PET process and caused the fluorescence color shift from blue to bright green. Besides, probe BIPP demonstrated superior Zn2+ specific selectivity and sensitivity in semi-aqueous conditions when compared to other competitive metal ions with a lower detection limit (2.36 × 10−8 M). The BIPP + Zn2+ complexation process was confirmed by electronic spectral titration, Job's plot, 1H NMR, and HRMS experiments. The efficacy of BIPP in imaging the presence of Zn2+ in E. coli and water samples indicates that it has a broad range of application possibilities. According to the findings in this study, we are certain that BIPP could be employed for Zn2+ detection in a variety of applications.
Conflicts of interest
There are no conflicts to declare.
Acknowledgements
Saravanan Enbanathan thanks VIT University for providing financial support through research associateship (VIT/HR/Fac.Appt./2021/6876). The DST-FIST NMR facility at VIT University is duly acknowledged. Finally, the authors thank Dr S. Meenakshi, SSL-VIT, for language editing.
Notes and references
- N. Kaur and B. Kaur, Microchem. J., 2020, 158, 105131 CrossRef CAS.
- S. Upadhyay, A. Singh, R. Sinha, S. Omer and K. Negi, J. Mol. Struct., 2019, 1193, 89–102 CrossRef CAS.
- D. Udhayakumari, Spectrochim. Acta, Part A, 2020, 228, 117817 CrossRef CAS.
- P. Roy, Coord. Chem. Rev., 2021, 427, 213562 CrossRef CAS.
- E. T. Sawey, M. Chanrion, C. Cai, G. Wu, J. Zhang, L. Zender, A. Zhao, R. W. Busuttil, H. Yee and L. Stein, Cancer Cell, 2011, 19, 347–358 CrossRef CAS PubMed.
- L. Li, R. Sun, R. Zheng and Y. Huang, Mater. Des., 2021, 205, 109759 CrossRef CAS.
- V. Gerke, C. E. Creutz and S. E. Moss, Nat. Rev. Mol. Cell Biol., 2005, 6, 449–461 CrossRef CAS.
- J. M. Berg and Y. Shi, Science, 1996, 271, 1081–1085 CrossRef CAS PubMed.
- Y. Chen, Y. Bai, Z. Han, W. He and Z. Guo, Chem. Soc. Rev., 2015, 44, 4517–4546 RSC.
- H. Huang, H. Lei, F. Yang, X. Fan, Q. Dang and Y. Li, Biomed. Pharmacother., 2018, 106, 1713–1719 CrossRef CAS PubMed.
- O. P. Ajsuvakova, A. A. Tinkov, D. Willkommen, A. A. Skalnaya, A. B. Danilov, A. A. Pilipovich, M. Aschner, A. V Skalny, B. Michalke and M. G. Skalnaya, J. Trace Elem. Med. Biol., 2020, 59, 126423 CrossRef CAS.
- M. Z. Khan, Biomed. Pharmacother., 2016, 79, 263–272 CrossRef CAS PubMed.
- U. Doboszewska, K. Młyniec, A. Wlaź, E. Poleszak, G. Nowak and P. Wlaź, Pharmacol. Ther., 2019, 193, 156–177 CrossRef CAS PubMed.
- X. Qian and Z. Xu, Chem. Soc. Rev., 2015, 44, 4487–4493 RSC.
- S. Khan, X. Chen, A. Almahri, E. S. Allehyani, F. A. Alhumaydhi, M. M. Ibrahim and S. Ali, J. Environ. Chem. Eng., 2021, 9, 106381 CrossRef CAS.
- A. Roy, M. Nandi and P. Roy, TrAC Trends Anal. Chem., 2021, 138, 116204 CrossRef CAS.
- X. Gong, X. Ding, N. Jiang, T. Zhong and G. Wang, Microchem. J., 2020, 152, 104351 CrossRef CAS.
- P. R. Dongare and A. H. Gore, ChemistrySelect, 2021, 6, 5657–5669 CrossRef CAS.
- A. Kumar, S. K. Hira and S. Dey, Eur. J. Inorg. Chem., 2020, 2020, 3771–3777 CrossRef CAS.
- C. A. S. Pothulapadu, A. Jayaraj, R. N. Priyanka and G. Sivaraman, ACS Omega, 2021, 6, 24473–24483 CrossRef CAS.
- X. He, F. Ding, X. Sun, Y. Zheng, W. Xu, L. Ye, H. Chen and J. Shen, Inorg. Chem., 2021, 60, 5563–5572 CrossRef CAS PubMed.
- G. Wang, X. Chen, S. Liu, C. Wong and S. Chu, ACS Nano, 2016, 10, 1788–1794 CrossRef CAS PubMed.
- J. Su, S. Zhang, C. Wang, M. Li, J. Wang, F. Su and Z. Wang, ACS Omega, 2021, 6, 14819–14823 CrossRef CAS PubMed.
- A. C. Di Wu and T. G. Sedgwick, Chem. Soc. Rev., 2017, 46, 7105–7123 RSC.
- P. Kumar, S. Pachisia and R. Gupta, Inorg. Chem. Front., 2021, 8, 3587–3607 RSC.
- M. H. Chua, H. Zhou, Q. Zhu, B. Z. Tang and J. W. Xu, Mater. Chem. Front., 2021, 5, 659–708 RSC.
- A. D. Cabral, T. B. Radu, E. D. de Araujo and P. T. Gunning, RSC Chem. Biol., 2021, 2, 815–829 RSC.
- Y. Li, Z. Yang, Z. Liu, B. Wang and S. Li, Sens. Actuators, B, 2011, 160, 1504–1507 CrossRef CAS.
- P. Mazumdar, S. Maity, D. Das, S. Samanta, M. Shyamal and A. Misra, Sens. Actuators, B, 2017, 238, 1266–1276 CrossRef CAS.
- C. Patra, A. K. Bhanja, C. Sen, D. Ojha, D. Chattopadhyay, A. Mahapatra and C. Sinha, Sens. Actuators, B, 2016, 228, 287–294 CrossRef CAS.
- Q.-F. Li, J.-T. Wang, S. Wu, G.-W. Ge, J. Huang, Z. Wang, P. Yang and J. Lin, Sens. Actuators, B, 2018, 259, 484–491 CrossRef CAS.
- X. Gao, X. Zhuang, C. Tian, H. Liu, W.-F. Lai, Z. Wang, X. Yang, L. Chen and A. L. Rogach, Sens. Actuators, B, 2020, 307, 127626 CrossRef CAS.
- L. Wang, W. Li, W. Zhi, Y. Huang, J. Han, Y. Wang, Y. Ren and L. Ni, Sens. Actuators, B, 2018, 260, 243–254 CrossRef CAS.
- J. Wang, X. Liu and Y. Pang, J. Mater. Chem. B, 2014, 2, 6634–6638 RSC.
- A. Gogoi, S. Mukherjee, A. Ramesh and G. Das, RSC Adv., 2015, 5, 63634–63640 RSC.
- K. Mawai, S. Nathani, P. Roy, U. P. Singh and K. Ghosh, Dalt. Trans., 2018, 47, 6421–6434 RSC.
- N. S. Mohamad, N. H. Zakaria, N. Daud, L. L. Tan, G. C. Ta, L. Y. Heng and N. I. Hassan, Sensors, 2021, 21, 311 CrossRef CAS PubMed.
- B. Mariammal, A. Shylaja, S. V. Kumar, S. R. Rubina and R. R. Kumar, J. Heterocycl. Chem., 2020, 57, 3882–3889 CrossRef CAS.
- Z. Wang, S. Cui, S. Qiu and S. Pu, J. Photochem. Photobiol., A, 2019, 376, 185–195 CrossRef CAS.
- S. Park, H. Lee, Y. Yi, M. H. Lim and C. Kim, Inorg. Chim. Acta, 2020, 513, 119936 CrossRef CAS.
- J. Mou, H. Qi, R. Xiang, S. Xu, J. Liu, S. Meng, N. Chen, Y. Xue and D. Pei, New J. Chem., 2021, 45, 2958–2966 RSC.
- X. He, Q. Xie, J. Fan, C. Xu, W. Xu, Y. Li, F. Ding, H. Deng, H. Chen and J. Shen, Dyes Pigm., 2020, 177, 108255 CrossRef CAS.
- W.-N. Wu, P.-D. Mao, Y. Wang, X.-L. Zhao, Z.-Q. Xu, Z.-H. Xu and Y. Xue, Spectrochim. Acta, Part A, 2018, 188, 324–331 CrossRef CAS PubMed.
- Z. Yu, W. Huang, S. Xu and S. Ke, Microchem. J., 2021, 164, 106009 CrossRef CAS.
- S. Li, D. Cao, X. Meng, Z. Hu, Z. Li, C. Yuan, T. Zhou, X. Han and W. Ma, Spectrochim. Acta, Part A, 2020, 230, 118022 CrossRef CAS PubMed.
- W. R. Lovett, A. Al Hamd, S. Casa and M. Henary, Dyes Pigm., 2021, 190, 109268 CrossRef CAS.
- R. Singh, A. Gogoi and G. Das, RSC Adv., 2016, 6, 112246–112252 RSC.
- H.-W. Zheng, Y. Kang, M. Wu, Q.-F. Liang, J.-Q. Zheng, X.-J. Zheng and L.-P. Jin, Dalt. Trans., 2021, 50, 3916–3922 RSC.
- C. Sravani and A. Sivaramakrishna, ChemistrySelect, 2017, 2, 5688–5694 CrossRef CAS.
- B. Suh, D. Choe and C. Kim, Color. Technol., 2021, 137, 512–519 CAS.
- S. Erdemir, S. Malkondu and O. Alici, Color. Technol., 2015, 131, 32–37 CAS.
- C. J. Rha, H. Lee and C. Kim, Inorg. Chim. Acta, 2020, 511, 119788 CrossRef CAS.
- L. Stroea, M. Murariu and V. Melinte, J. Mol. Liq., 2020, 318, 114316 CrossRef CAS.
- S. A. Khan, Q. Ullah, H. Parveen, S. Mukhtar, K. A. Alzahrani and M. Asad, J. Photochem. Photobiol., A, 2021, 406, 113022 CrossRef CAS.
- J. S. Ganesan, M. Sepperumal, A. Balasubramaniem and S. Ayyanar, Spectrochim. Acta, Part A, 2020, 230, 117993 CrossRef CAS PubMed.
- G. Emandi, K. J. Flanagan and M. O. Senge, Photochem. Photobiol. Sci., 2018, 17, 1450–1461 CrossRef CAS.
- A. O. Eseola, H. Görls, M. Bangesh and W. Plass, New J. Chem., 2018, 42, 7884–7900 RSC.
- R. C. M. Ferreira, S. P. G. Costa, H. Gonçalves, M. Belsley and M. M. M. Raposo, New J. Chem., 2017, 41, 12866–12878 RSC.
- D. Jothi, S. Manickam, S. Sawminathan, S. Munusamy, S. K. A. Kumar and S. K. Iyer, Dyes Pigm., 2022, 197, 109826 CrossRef CAS.
- D. Jothi, S. Munusamy and S. K. Iyer, J. Photochem. Photobiol., A, 2021, 420, 113491 CrossRef CAS.
- S. Sawminathan, S. Munusamy, S. Manickam, D. Jothi and S. KulathuIyer, Dyes Pigm., 2021, 196, 109755 CrossRef CAS.
- S. Enbanathan, S. Munusamy, D. Jothi, S. Manoj kumar, A. P. Gopal and S. KulathuIyer, Dyes Pigm., 2022, 110514 CrossRef CAS.
- S. Munusamy, S. Swaminathan, D. Jothi, V. P. Muralidharan and S. K. Iyer, RSC Adv., 2021, 11, 15656–15662 RSC.
- Y. Yang, Y. Feng, Y.-Z. Wang, F.-Z. Qiu, X.-L. Tang, G.-L. Zhang and W.-S. Liu, Sens. Actuators, B, 2017, 253, 1055–1062 CrossRef CAS.
- Y. Wang and S. Yang, Chem. Phys. Lett., 2020, 761, 138024 CrossRef CAS.
- A. M. Blanco-Rodriguez, H. Kvapilova, J. Sykora, M. Towrie, C. Nervi, G. Volpi, S. Zalis and A. Vlcek Jr, J. Am. Chem. Soc., 2014, 136, 5963–5973 CrossRef CAS PubMed.
- C. Garino, T. Ruiu, L. Salassa, A. Albertino, G. Volpi, C. Nervi, R. Gobetto and K. I. Hardcastle, Eur. J. Inorg. Chem., 2008, 3587–3591 CrossRef CAS.
- G. Volpi, C. Garino, L. Salassa, J. Fiedler, K. I. Hardcastle, R. Gobetto and C. Nervi, Chem.–Eur. J., 2009, 15, 6415–6427 CrossRef CAS PubMed.
- V. Gite, N. Khairnar, A. Basu, A. Kuwar, N. Singh, J. Singh, B. Bondhopadhyay, K. Tayade and S. K. Sahoo, Dalton Trans., 2015, 44, 2097–2102 RSC.
- M. Patil, S. Bothra, S. K. Sahoo, H. A. Rather, R. Vasita, R. Bendre and A. Kuwar, Sens. Actuators, B, 2018, 270, 200–206 CrossRef CAS.
- S. Seenan and S. Kulathu Iyer, J. Org. Chem., 2020, 85, 1871–1881 CrossRef PubMed.
- F. dos Santos Carlos, L. A. da Silva, C. Zanlorenzi and F. S. Nunes, Inorg. Chim. Acta, 2020, 508, 119634 CrossRef.
- Y. Oubelkacem, T. Lamhasni, A. El Bakkali, S. A. Lyazidi, M. Haddad and A. Ben-Ncer, Spectrochim. Acta, Part A, 2021, 247, 119093 CrossRef CAS.
- S. Enbanathan, S. Manickam, S. Munusamy, D. Jothi, S. Manoj Kumar and S. Kulathu Iyer, New J. Chem., 2022, 46(14), 6570–6576 RSC.
- D. Jothi, S. Munusamy, S. Manoj kumar, S. Enbanathan and S. Kulathu Iyer, RSC Adv., 2021, 12(14), 8570–8577 RSC.
|
This journal is © The Royal Society of Chemistry 2022 |
Click here to see how this site uses Cookies. View our privacy policy here.