DOI:
10.1039/D2RA07300E
(Paper)
RSC Adv., 2022,
12, 34746-34759
Insights into chalcone analogues with potential as antioxidant additives in diesel–biodiesel blends†
Received
17th November 2022
, Accepted 17th November 2022
First published on 5th December 2022
Abstract
Biodiesel production is one of the promising strategies to reduce diesel consumption and an important contribution to climate change. However, biodiesel stability remains a challenging problem in biofuel use in the global energy matrix. In this context, organic additives have been investigated to minimize these problems and reduce harmful emissions to comply with fuel requirement standards. In this study, we discuss a comprehensive structural description, a behavior of B15 [85% volume of diesel and 15% volume of biodiesel (B100)] stability in the presence of antioxidants (chalcone analogues), and a theoretical calculation to pave the way for clarifying and expanding the potential of title compounds as an antioxidant additive for diesel–biodiesel blends. Finally, a systematic description of the oxidation stability was undertaken using a specialized machine learning computational pySIRC platform.
1. Introduction
Recently, anthropogenic environmental impacts have been under discussion focused on the world, promoting changes in different societal sectors.1,2 From the energetic scope, new policies have restricted the fossil fuels use that will gradually be replaced by renewable energy matrices, which are more sustainable and generate lower levels of pollutant emissions.3–6 The United Nations Conference on Climate Change (COP26)7 has discussed issues such as reducing pollution emissions and dependence on fossil fuels through the use of renewable energy.8,9 Countries have also made commitments to step up and phase out coal, end international financing for fossil fuels, 50% reduction in greenhouse gas emissions by 2030, and among other objectives, pave the pathway for a net-zero emission future.10–13
The use of biodiesel from biomass feedstocks is recognized as a sustainable option and advocated due to the serious global environmental security challenges.14–16 The properties of biodiesel are similar to those of petro-diesel with significant benefits, including being non-toxic, having a higher flash point (423 K for biodiesel as compared to 337 K for petro-diesel)14 and noteworthily lower pollutant emissions and particulate matter.17–19 However, the full adoption of biofuels is hampered by the fact that they are more susceptible to oxidation than mineral diesel.17,19–21 The reduction of the blend's shelf-life, when used after long storage periods, can damage mechanical elements in the engine, reduce efficiency, and have the potential to give rise to harmful emissions.17–20,22,23 The degradation rate is considered one of the most important parameters to evaluate the shelf-life of the blend during its storage, where a low value of rate constant indicates greater oxidative stability.24,25
Generally, biodiesel filled with natural or synthetic antioxidant additives can decrease the rate of degradation reactions in biodiesel.17,21,26 The additives when associated with biodiesel fractions are a gimmick to mitigate nitrogen oxide (NOx) emissions from the fuel combustion in diesel engines.17–19,27 The development of chemical additives whose properties favor better storage stability for biodiesel and its blends with diesel is needed. Previous studies have shown that the addition of chalcone compounds into diesel–biodiesel blends reduces the rate of the oxidation process.28,29 This class of flavonoid intermediates is formed by two aromatic rings linked by a three-carbon bridge having a keto carbonyl group and one α,β-unsaturation, obtained from natural sources30–32 or synthetic pathways.33,34 Some physicochemical properties, such as antibacterial,35–37 antifungal,38–40 and antioxidant,28,41 are the key issue for their ability to prevent free radical-induced oxidative damage driven by substitutions in chalcone rings as a function of their chemical flexibility.42–47
In light of these facts, and as part of our ongoing work regarding the application of chalcones as antioxidant biodiesel additives,28,29,48 in this paper, we discuss the synthesis, extensive structure characterization, oxidation stability test, and density functional theory (DFT)49 calculations for (2E)-3-(4-bromophenyl)-1-[4-(2-oxo-2-phenylethoxy)phenyl]prop-2-en-1-one (BCH) and (2E)-3-(3-nitrophenyl)-1-[4-(2-oxo-2-phenylethoxy)phenyl]prop-2-en-1-one (NCH) (see structures in Fig. 1). Because fundamental thermodynamic parameters are stimulated by changing aromatic ring substitutions50–52 we hypothesized that BCH and NCH, in terms of biodiesel feedstock,17,19,53 could be investigated as an additive for diesel–biodiesel blends. An explanation of the kinetic factors involved in oxidation stability was assessed using a machine-learning computational platform.
 |
| Fig. 1 The ORTEP diagram for BCH (a) and NCH (b). The ellipsoids are represented at a 75% probability level with the atomic numbering scheme. Hydrogen atoms are represented by spheres with arbitrary radii. Structure overlay for BCH and NCH δ0 = 4.60° (c). | |
2. Experimental and computational procedures
2.1. Synthesis and spectroscopy characterization
1.2 mL of K2CO3 (0.2 mmol) was added to the solution of hydroxychalcone (0.1 mmol) in acetone and the system cooled to 0 °C. Subsequently, phenacyl bromide (0.14 mmol) was added in a single portion, and the mixture was stirred at room temperature for 2 h. The reaction was monitored by thin-layer chromatography (TLC) and quenched with distilled water (1.2 mL), and the product was extracted with CH2Cl2 (3 × 10 mL). The synthesis was carried out with specific chemical elements (hydroxychalcone, K2CO3, phenacyl bromide, CH2Cl2, anhydrous Na2SO4, and methanol) that were purchased from companies specialized in the sale of chemical reagents.54 BCH 1H NMR (500 MHz, CDCl3): 5.39 (s, 1H); 7.02 (m, 2H); 7.49 (m, 7H); 7.66 (m, 1H); 7.71 (d, 1H, J = 15.7 Hz); 8.02 (m, 4H). 13C NMR (125 MHz, CDCl3): 70.5; 114.4; 114.6; 122.3; 124.6; 128.1; 129.0; 129.7; 130.8; 132.2; 134.2; 142.8; 161.8; 188.4. NCH 1H NMR (500 MHz, CDCl3): 5.41 (s, 1H); 7.05 (m, 2H); 7.54 (m, 2H); 7.60 (d, 1H, J = 15.9 Hz); 7.66 (m, 2H); 7.81 (d, 1H, 15.7 Hz); 7.91 (m, 1H); 8.02 (m, 2H); 8.06 (m, 2H); 8.25 (m, 1H); 8.51 (m, 1H). 13C NMR (125 MHz, CDCl3): 70.5; 114.8; 122.2; 124.5; 128.1; 129.0; 130.2; 131.0; 131.3; 131.7; 134.2; 141.0; 145.8; 162.1; 187.8; 193.4.
2.2. Crystallographic analysis
The organic phase was dried with anhydrous Na2SO4, filtered, and concentrated in a vacuum. After recrystallization by slow evaporation of methanol, the product was obtained as a yellow crystalline solid with a 34% yield. The X-ray diffraction data were obtained using a Bruker APEX II CCD diffractometer fitted with MoKα radiation (λ = 0.71073 Å) at room temperature. The structure was solved on SHELXT55 and refined on SHELXL56 using the OLEX2 (ref. 57) platform. The non-hydrogen atoms were refined anisotropically. The hydrogen atoms were refined using the riding model with the individual isotropic displacement parameters or Uiso(H) = 1.2Ueq of the bonded carbon. All supramolecular representations were generated using mercury58 and molecular interactions were checked using PLATON.59 The crystallographic information files were deposited at the Cambridge Crystallographic Data Centre (CCDC)60 under codes 2182574 and 2182577.
The hirshfeld surface (HS) describes intermolecular interactions through color-scaled mapping (red, white, and blue) that permits the identification and description of surface properties.52 Where red indicates regions with short intermolecular contacts, white indicates contacts around the separation of the van der Waals radii interface, and blue indicates long contact distances.52 The three-dimensional graph of the HS, the dnorm (normalized distance), was constructed by the surface distance to the closest exterior atom (de), where the molecules act as the strongest intermolecular contact receptors, by the surface distance to the closest interior atom (di), where the molecule acts as the strongest intermolecular donor contact, with the van der Waals radius (rvdWi and rvdWe) for each atom involved in this close contact with the surface:61
|
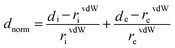 | (1) |
The complementarity between molecules in the crystal packing, hydrophobic intermolecular interactions, and interactions involving the π system (π … π interactions), was identified from the shape index surface.61 In addition, the combination of de and di, generate unique fingerprints for each molecule in the form of 2D plots, allowing a quantitative analysis of each type of intermolecular contact.61
2.3. Storage stability test
The storage stability of S10 diesel containing a 15% volume of biodiesel (S10 B15 diesel) was verified. The S10 B15 diesel was composed of 85% volume of biodiesel-free reference diesel (fuel according to RANP 764/2018,62 applied on emission and consumption tests) and 15% volume of biodiesel (B100) (composed of 60% volume of soybean oil, 37% volume of palm oil, 2% volume of bovine fat and 1% volume of pig fat). Thus, high-density polyethylene (HDPE) bottles of 1 liter capacity were filled with 300 mL of each sample: bottle 1 – diesel S10 B15 containing 109.0 ppm of the BCH, bottle 2 – diesel S10 B15 containing 100.7 ppm of NCH and bottle 3 – diesel S10 B15 without additive. The three flasks remained in an external environment for 90 days. After this period, the oxidation stability was measured using the modified Rancimat method according to the EN 15751:14 standard.63
2.4. Oxidation stability test
The modified Rancimat test method is an important experiment applied to verify diesel–biodiesel blend oxidation stability. It is a standard procedure for the storage stability certification of diesel cycle engine fuels. In the Rancimat test, the sample was heated to 110 °C and subjected to a purified airflow to supply oxygen (induction period process). Thus, oxygen promotes oxidation reactions in the sample, forming peroxides as products, which, due to the high temperature, are dissociated and form volatile compounds such as aldehydes, ketones, and carboxylic acids. These compounds are directed to a container filled with deionized water, which is coupled to a conductivity electrode, and are detected when the conductivity increases quickly, indicating high ion concentrations and the end of the test. The induction period is reported as hours. Therefore, the longer the induction period, the greater the oxidation stability of the sample.64,65 For our analysis, Metrohm 873 model equipment was applied according to EN 15751: 2014 test method.63
2.5. Computational procedures
The theoretical analysis for both chalcones was performed using DFT.49 The geometric parameters obtained from the X-ray data were optimized in the gas phase, with theoretical calculations carried out in the Gaussian09 (ref. 66) software, using M06-2X/6-311++G(d,p),67–69 From the generated wave function, Frontier molecular orbitals were calculated, (highest occupied molecular orbital (HOMO) and lowest unoccupied molecular orbital (LUMO).50,51 To investigate the reactive sites of both chalcones, the MEP map was constructed from the equation: |
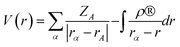 | (2) |
where® (r) is a potential created at a point r, the first term of summation is the electrostatic potential created by the nucleus and the second term is the electrostatic potential created by electrons.70
To identify the main oxidation sites of BCH and NCH, appropriate local reactivity descriptors, such as Fukui function (f),71,72 were calculated according to equations
,
, and fNBO ≈ fNBO+ + fNBO−/2. The Multiwfn package program73 was used to study the topological and Fukui functions. The oxidation reactions promoted by oxygen supply were simulated by pySIRC74 – a machine learning computational platform. The hydroxyl radical – an archetypal system of degradation reactions – was selected to mimic the oxidation effect promoted using the oxygenation process.
3. Results and discussion
3.1. Solid-state description
BCH and NCH crystallize in the P
and the P21 space groups, respectively. Table 1 summarizes the structure refinement parameters, and the ORTEP75 representation is shown in Fig. 1. Structural differences between the two compounds occur in the ligands of aromatic ring 3. A bromine atom in BCH is bonded an ortho carbon (C21), and a hydrogen atom is bonded to the meta carbon (C20). On the other hand, in NCH the ligand in position ortho is a hydrogen atom, while a nitro group is bound to the meta carbon (C20).
Table 1 Crystal data and structure refinement for BCH and NCH
Parameter |
BCH |
NCH |
Empirical formula |
C23H17BrO3 |
C23H17NO5 |
Formula weight |
421.27 |
387.38 |
Temperature K−1 |
120.02 |
120 |
Crystal system |
Triclinic |
Monoclinic |
Space group |
P![[1 with combining macron]](https://www.rsc.org/images/entities/char_0031_0304.gif) |
P21/n |
a/Å |
6.6262(3) |
7.3047(12) |
b/Å |
7.4872(4) |
11.062(2) |
c/Å |
19.2803(10) |
22.299(4) |
α/° |
87.424(2) |
90 |
β/° |
87.609(2) |
98.363(3) |
γ/° |
71.455(2) |
90 |
Volume/Å3 |
905.56(8) |
1782.7(6) |
Z |
2 |
4 |
ρcalc g cm−3 |
1.545 |
1.443 |
μ/mm−1 |
2.291 |
0.103 |
F(000) |
428 |
808 |
Crystal size mm−3 |
0.316 × 0.177 × 0.024 |
0.618 × 0.123 × 0.109 |
Radiation |
MoKα (λ = 0.71073) |
MoKα (λ = 0.71073) |
2Θ range for data collection/° |
4.232 to 56.422 |
3.692 to 56.558 |
Index ranges |
−8 ≤ h ≤ 8, −9 ≤ k ≤ 9, −25 ≤ l ≤ 25 |
−9 ≤ h ≤ 9, −14 ≤ k ≤ 14, −29 ≤ l ≤ 29 |
Reflections collected |
10 681 |
25 512 |
Independent reflections |
4424 [Rint = 0.0254, Rsigma = 0.0355] |
4432 [Rint = 0.0288, Rsigma = 0.0197] |
Data/restraints/parameters |
4424/0/244 |
4432/0/262 |
Goodness-of-fit on F2 |
1.011 |
1.019 |
Final R indexes [I ≥ 2σ (I)] |
R1 = 0.0327, wR2 = 0.0705 |
R1 = 0.0373, wR2 = 0.0981 |
Final R indexes [all data] |
R1 = 0.0447, wR2 = 0.0746 |
R1 = 0.0509, wR2 = 0.1058 |
Largest diff. peak/hole/e Å−3 |
0.43/−0.36 |
0.39/−0.23 |
Bond lengths (Table S1†) of BCH and NCH of carbon–carbon and carbon-oxygen were very close to each other for the chalcones. The largest variations occurred for O2–C9 (0.0084 Å), C21–C22 (0.0079 Å), C6–C7 (0.0063 Å), and C2–C3 (0.0058 Å). In turn, bond angles (Table S2†) calculated for the BCH and NCH present the largest variations in C20–C21–C22 (4.07°), C21–C20–C19 (4.73°), C18–C19–C20 (2.51°), where the angles in parentheses represent the calculated difference between BCH and NCH for each bonded trio atoms. The angular variations occurred because of the different compounds attached to aromatic ring 3 for each chalcone, affecting the angular positions of C18, C19, C20, C21, and C22 atoms, which together with carbon 23, form the aromatic ring 3. BCH (Fig. S1a†) is almost planar and displays an angle between aromatic rings 1 and 2 δ1 = 6.75°.
A major deviation from planarity was found between aromatic rings 2 and 3, with a plan angle of δ2 = 13.03°. In NCH, however (Fig. S1b†), the interplanar angles are δ3 = 9.24° (between rings 1 and 2) δ4 = 6.62° (between rings 2 and 3) and the largest deviation from planarity is δ5 = 21.83° (between rings 1 and 3). The superposition of the chalcones (Fig. 1c) allows the comparison between the geometric parameters of the structures. The root means squared (RMS) value predicted by the mercury program package is 0.0194 Å. Changing the substituent on aromatic ring 3 promotes a significant improvement in the planarity, since the planarity in the chalcones is related to donor–acceptor interactions in a molecule, on position and the vicinity of the substituents.76–78 Chalcones have structures that are generally identified with almost planar conformation, a conformational requirement that may be related to their potential for antifungal activity.78–82 This activity is necessary to maintain the quality of fuels since the proliferation of microorganisms is harmful to the system.83,84
Crystal engineering seeks to explore the multiplicity of intermolecular interactions in a crystalline environment. HS analysis allows the location of crucial contacts on the packaging, dependent on molecular geometry, locations, and orientation of the neighbouring molecules. The interactions listed in Table 2 were confirmed by electron density HS mapped over dnorm (ranging from −0.1019 to 1.3604 BCH and −0.1685 to 1.4111 NCH). The supramolecular arrangement of BCH (Fig. 2a and b) is stabilized by the C3–H3…Br interaction contributing to the direction [001] described as C11(20) and weak interactions C4–H4…O1, involving aromatic hydrogen since the most significant hydrogen bond motif is C11(6) and R22(16) C13–H13…O1 forming a dimer with periodicity along [100] in a bifurcated fashion. In contrast, the supramolecular structure of NCH crystal (Fig. 2c and d) has three dimers and a bifurcated interaction along the [010] direction, C5–H5…O4 (IV) C22–H22…O2 (V)C22–H22…O1 (VI), C13–H13…O1 (VII) with motifs C11(19), R22(24), R22(30) and R22(16) respectively, and C2–H2…O5 contributing to the [100] direction.
Table 2 Hydrogen-bond geometry obtained from structural analysis for BCH and NCH
|
D–H … A |
D–H (Å) |
H … A (Å) |
D … A (Å) |
D–H … A (°) |
Symmetry code |
BCH |
C3–H3…Br |
0.95(1) |
2.92(3) |
3.867(2) |
173(4) |
−2 + x, 1 + y, 1 + z |
C4–H4…O1 |
0.95(1) |
2.54(5) |
3.166(3) |
123(6) |
−1 + x,y,z |
C13–H13…O1 |
0.95(1) |
2.703(4) |
3.494(3) |
141(5) |
1 − x, 2 − y, 1 − z |
NCH |
C5–H5…O4 |
0.95(1) |
2.62(5) |
3.363(1) |
134(4) |
1 − x, 1 − y,1 − z |
C22–H22…O2 |
0.95(1) |
2.56(6) |
3.456(16) |
157(6) |
1 − x, 2 − y, 1 − z |
C22–H22…O1 |
0.95(1) |
2.64(4) |
3.254(2) |
122(6) |
1 − x, 2 − y, 1 − z |
C13–H13…O1 |
0.95(1) |
2.63(3) |
3.463(2) |
145(4) |
−x, 2 − y, 1 − z |
C2–H2…O5 |
0.95(1) |
2.47(4) |
3.2077(16) |
135(3) |
−5/2 + x,3/2 − y,1/2 + z |
 |
| Fig. 2 The representation of interactions and HS dnorm showing the contacts for BCH (a) C3–H3…Br(I) C4–H4…O1(II) and (b) C13–H13…O1 (III). The NCH (c) C5–H5…O4 (IV), C22–H22…O2 (V) and C22–H22⋯O1 (VI) and (d) C13–H13…O1 (VII) and C2–H2…O5 (VIII). | |
The π…π stacking is a type of non-covalent interaction, represented by the red and blue triangles (bow tie), and contributes to the stability of structures. For this interaction, the centroid of the aromatic rings for the compounds was calculated, with the distance between the two rings. The distance between the centroids, formed between the center of the aromatic rings is d1 = 3.746 Å and d2 = 3.729 Å for BCH (Fig. 3a) and d3 = 3.802 Å d4 = 3.709 Å and d5 = 3.725 Å for NCH (Fig. 3b). The introduction of nitro group atoms into the structure caused a decrease in the stacking distance π…π.
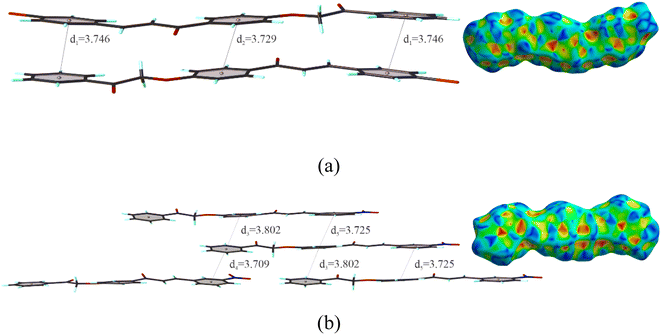 |
| Fig. 3 . Shape index surfaces for evidencing π…π interactions and representation of BCH (a) and NCH (b). | |
The relative surface area resulting from the contributions of the interactions exposed in the crystal is quantified by analysing the fingerprint (Fig. 4), generated from the de vs. di plot. The graph represents a bin formed by intervals (0.2 Å). These can be decomposed into contributions of specific atoms, which makes it possible to measure the relative importance of several contacts when comparing the structure. Most of the contacts are H⋯H, which represents 38.2% of the HS for BCH and 35.2% for NCH, characterized by the central peak of the graph. O⋯ H interactions are characterized by the small peaks at the bottom of the fingerprint, representing 16.4% for BCH and 31.6% for NCH. The high difference is explained by the presence of the nitro group and the competition caused by the bromine atom in BCH shown by the peaks at the side edge of the graph. Also, we can ascertain that for NCH the most and strongest hydrogen bonds are present. Furthermore, the C⋯ C contacts with 12.1% for BCH and 13.0% NCH, present in the center, which helps in understanding π⋯π interaction. The interlayer contacts of C⋯O show weak interactions (distances above 3.5 Å) and therefore can be neglected.
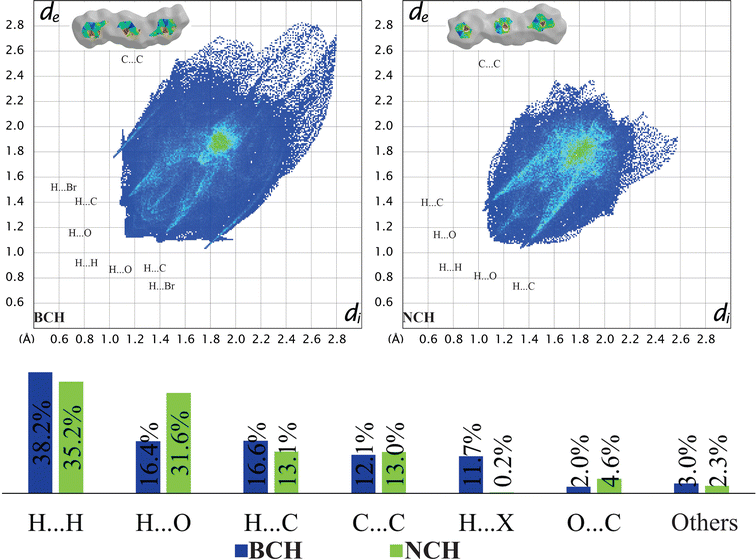 |
| Fig. 4 Fingerprint plots with quantitative analysis of the contacts for BCH and NCH (where X = Br and N). | |
3.2. Antioxidant additive analysis
The shelf-life tests evaluated the performance of BCH and NCH chalcones as antioxidant additives for diesel–biodiesel blends during storage of 90 days. Brazilian legislation does not specify the minimum oxidation stability for the commercial diesel-biodiesel blend. However, the resolution ANP 798/2019(ref. 85) determines a minimum limit of oxidation stability at 110 °C for commercial biodiesel (B100): a minimum of 12 hours, measured according to EN 15751: 2014 test method.63 However, European regulations include the specification of oxidation stability for the diesel–biodiesel blend BS EN 590:2009,86 which establishes requirements and test methods for automotive fuels used in diesel cycle engines. As such, the result of the induction period shall be higher than 20 hours for the fuel to be considered suitable for use.
During the sample preparation at the test start, chalcone concentrations added to the flasks differed by 8.3 ppm. Converting this change-over to mol quantity/volume unity, this variation is less significant, on the order of 1.2 × 10−9 mol.mL−1. Therefore, the chalcones' content was very similar in these two cases. The results shown in Table 3 indicate the oxidation stability values above 30 hours for two types of mixtures (containing BCH e NCH), both results above the induction period of biodiesel without additives that were subjected to the same stability test conditions. The average oxidation stability obtained for the fuel containing the NCH was 2.3% higher than that for the fuel with BCH. Joshi et al. (2013)87 showed that the additive concentrations (300, 400, and 500 ppm) are correlated with the stability; the additives butylated hydroxy anisole (BHA), butylated hydroxytoluene (BHT), pyrogallol (PY), propyl gallate (PG), tert-butylhydroxyquinone (TBHQ), and diphenylamine (DPA) showed the values under 30 h to B15 blend. Also, Rizwanul et al. (2014)88 showed the following oxidative stability values for the high concentration of additives (2000 ppm) to the B20 blend: TBHQ (28.38 h) > BHT (25.28 h) > BHA (22.27 h). Agarwal et al. (2015)89 showed the following values for 500 ppm concentration of additives on pure biodiesel (B100): BHT (5 h), BHA (6 h), TBHQ (4), PG (15 h) and PY (25 h). Moreover, the linear correlation between the antioxidant concentration and the induction period was observed,17,90–92 which could allow assessing the minimum additive concentrations to their respective effectiveness. The use of chalcone analogues is promising in retaining the biodiesel physicochemical properties during the oxidative processes – especially at low concentrations (typically 200–2000 ppm).17,92
Table 3 Oxidation stability results from the modified Rancimat method
Modified Rancimat |
Result 1 |
Result 2 |
Result 3 |
Average |
Standard deviation |
S10 B15 + BCH (109.0 ppm) |
32.15 h |
31.27 h |
30.73 h |
31.38 h |
0.585 |
S10 B15 + NCH (100.7 ppm) |
32.78 h |
32.00 h |
31.51 h |
32.10 h |
0.523 |
S10 B15 without additives |
31.21 h |
31.23 h |
30.78 h |
31.07 h |
0.208 |
The modified Rancimat (EN 15751: 2014 test method)63 defines the repeatability factor (r) applied to results obtained by the same operator with the same apparatus under constant operating conditions. It is determined from eqn (3) with a confidence level of 95%, in which x is the three average results (Table 3):
|
r = 0.22027 + 0.04344x
| (3) |
The uncertainties were estimated from the repeatability factor, and they are registered in Fig. 5, both equal to approximately ± 1.6 h.
 |
| Fig. 5 The measurement uncertainty estimate of oxidation stability results from the modified Rancimat method. | |
According to Karavalakis et al. (2009)93 fuel aging can be accelerated in the presence of heat, oxygen, water, metal ions, and other impurities, favoring a viscosity increase due to gum and residue formation.94 During the oxidation process of diesel–biodiesel blends, there is also a reduction in the flash point because of aldehyde formation, as well as an increase in the acidity number due to the formation of acids, mainly formic and acetic.94 Thus, an antioxidant additive must delay these fuel aging processes, guaranteeing them a longer shelf-life under ideal conditions of use. The S10 B15 blend was produced from reference biodiesel-free diesel (fuel type applied on standard emission tests), which normally shows a better value of oxidation stability in relation to common diesel, considering this application is more rigorous. Thus, it is important to perform new tests using common diesel for blends (Table 3) and verify if it would exceed 30 hours in the presence of BCH and NCH and for additive-free samples. Normally, S10 B15 diesel prepared from common B0 diesel has lower induction period values when evaluated using the modified Rancimat method. Additional tests are required to evaluate the performance of BCH and NCH as antioxidant additives applied to diesel–biodiesel blends prepared using common diesel.
3.3. Molecular modeling analysis
The HOMO and LUMO orbitals over the BCH are similar while NCH presented differences. For BCH, the HOMO and LUMO orbitals are over the aromatic rings 2 and 3, while for NCH, the LUMO orbitals are located over aromatic rings 2 and 3, and the HOMO orbitals are located over aromatic ring 2 (Fig. 6). The HOMO orbital for both chalcones characterizes the bonding orbital, ionization potential (nucleophilic character) while the LUMO orbital represents the antibonding orbital, electron affinity (electrophilic character). The energy calculated for these orbitals for BCH is ELUMO = −159.55 kJ mol−1, EHOMO = −757.66 kJ mol−1 and EGAP = 598.11 kJ mol−1 (ELUMO–EHOMO), while for NCH is ELUMO = −197.62 kJ mol−1, EHOMO = −784.00 kJ mol−1 and EGAP = 586.38 kJ mol−1.
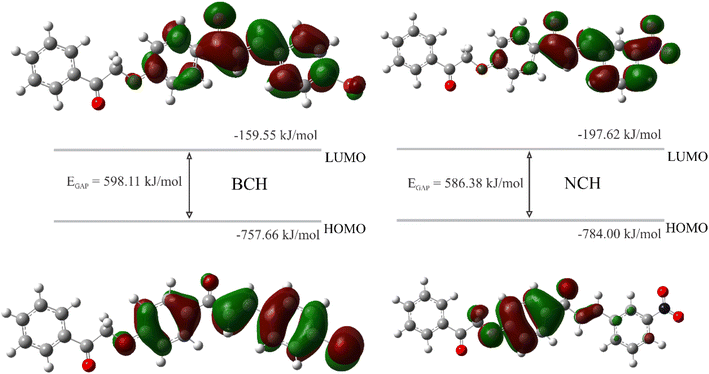 |
| Fig. 6 Frontier molecular orbitals (HOMO/LUMO) for BCH and NCH. Orbitals calculated at M06-2X/6311++G(d, p) level of theory. | |
The high GAP energy values suggest high kinetic stability and low chemical reactivity.95 According to these parameters, the EGAP for BCH suggests greater kinetic stability than NCH. However, the values are very close. Some values of EGAP for antioxidant molecules or commercial additives, such the BHT have GAP values of about 546.1 kJ mol−1,96,97 toluene derivatives with GAP values of 477.8 kJ mol−1,98 ether molecules with a GAP value ranging from 273.8 kJ mol−1 to 404.6 kJ mol−1,99 thiazolidinone molecules (308.5 to 481.8 kJ mol−1)100 and the p-phenylenediamine (56.8 kJ mol−1 to 114.5 kJ mol−1).101,102 The GAP values found for BCH (598.11 kJ mol−1) and NCH (586.38 kJ mol−1) are similar and greater than those found in the literature, indicating that both chalcones have greater kinetic stability than those already reported.
MEP (Fig. 7) was used to predict the reactivity of compounds and determine regions where nucleophilic (blue colors) and electrophilic (red colors) attacks are expected. The most electronegative zones occur in the vicinity of oxygens for the two molecules. Due to the nitro group in the NCH, additional zones of high electronegative density are generated, while the BCH presents a zone with positive electrostatic potential (+18.64 kJ mol−1). Therefore, the order of decreasing electrophilic attack for the discussed compounds should be in BCH O3 (−125.68 kJ mol−1) > O2 (−113.50 kJ mol−1) > O1 (−112.84 kJ mol−1) and in NCH O2 (−121.87 kJ mol−1) > O1 (−119.69 kJ mol−1) > O3 (−114.26 kJ mol−1) > O4 (−110.61 kJ mol−1) > O5 (−110.84 kJ mol−1). In addition, regions with light blue coloration are sites with weak interaction.
 |
| Fig. 7 MEP map for (a) BCH and (b) NCH. The density isovalue of ρ(r) = 4.0 × 10−4 electrons per bohr3contour encompassing the molecule. | |
To evaluate the main active sites of both molecules through an oxidative process, Fukui functions were calculated. These functions represent an important approach to explaining the reactivity in chemical systems to a radical attack.71,72,103,104 Fig. 8 illustrates significant values (nomenclature in Fig. 1) for the selected atoms. According to Fukui's formulation,71 higher values are associated with a higher probability of radical attack (fNBO). In this study, the carbon attached to the bromine atom C21 in BCH has a Fukui index of 0.055, while the carbon attached to the nitro group C20 in NCH has a Fukui index of 0.035, indicating that the reactivity of the NCH is lower in an oxidative process, which is consistent with the data obtained experimentally.
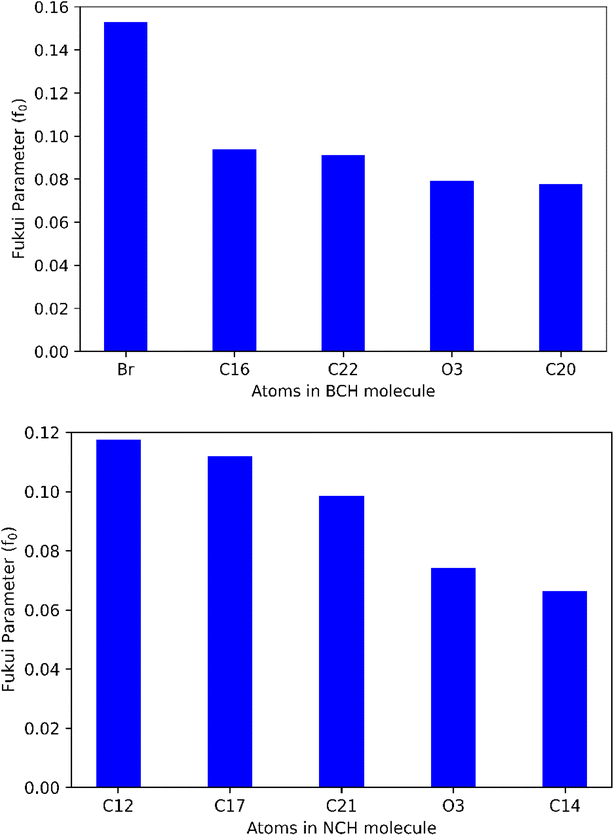 |
| Fig. 8 Fukui function values for the radical attack on selected atoms of both molecules (see nomenclature in Fig. 1). | |
The reaction rate constant is the most important parameter to reveal the efficiency of the degradation of a compound; in this sense, the oxidative degradation rates by the hydroxyl radical attack of BCH, NCH, diesel (represented by C10H20 molecule), and biodiesel (represented by C17H35COOCH3 fatty acid methyl ester) compounds were calculated using a web application based on machine learning models, pySiRC,74 whose values are 7.15 × 109 M−1 s−1, 6.01 × 109 M−1 s−1, 6.20 × 109 M−1 s−1, and 4.98 × 109 M−1 s−1, respectively. These values suggest that oxidative degradation in BCH has a higher oxidation potential than in NCH, which is consistent with the results obtained experimentally by the modified Rancimat method. Thus, the additive is first “sacrificed” so that the later degradation occurs later in the biodiesel, indicating greater oxidative stability of the biodiesel.
4. Conclusions
The structural analysis of the compounds associated with the evaluation of their physicochemical properties provides important information that contributes to the discovery of new compounds with potential technological applications, including their use as additives for biodiesel blends, considering also that the structural conformation directly affects the chemical and reactional characteristics of a molecule. In this work, it was verified that the change of the components linked to the aromatic ring 3 of both chalcones promoted important structural changes, including that from the conformation of the crystals obtained to the reaction characteristics. Also, it indicates that the application of additives in diesel–biodiesel blends is important for preserving fuel characteristics.
The oxidative stability test for fuel + chalcones indicated that the performance of NCH even at lower concentrations (109.0 ppm of BCH and 100.7 ppm of NCH) is more promising than BCH as an antioxidant agent in a diesel/biodiesel blend. The two compounds delayed the degradation of the biofuel, preserving its properties for 90 days of storage, without any additional processing. Complementary tests can confirm whether other desirable functions of an additive in this metric are maintained or not. Theoretical calculations show that compared to other compounds similar to additives, BCH and NCH show greater kinetic stability since the chalcones are likely to be first oxidized compared to diesel-biodiesel composition. The application of these methods paved the way for clarifying and expanding the potential of BCH and NCH compounds as antioxidant additives for diesel–biodiesel blends.
Conflicts of interest
The authors declare that they have no known competing financial interests or personal relationships that could have appeared to influence the work reported in this study.
Acknowledgements
The authors are grateful to Coordenação de Aperfeiçoamento de Pessoal de Nível Superior, Conselho Nacional de Desenvolvimento Científico e Tecnológico and Fundação de Amparo à Pesquisa de Goiás. Theoretical calculations were performed in the High-Performance Computing Center of the Universidade Estadual de Goiás, and Rancimat experiments were performed in the Research and Energy Efficiency Center (CAOA Montadora de Veículos Ltda).
References
- A. J. Ryan and R. H. Rothman, Engineering chemistry to meet COP26 targets, Nat. Rev. Chem., 2022, 6, 1–3 CrossRef.
- E. Aines, Z. Clulow, A. Neilson, E. Shuckburgh and S. Evans, COP26 Futures We Want-UK Country Profile, 2021 Search PubMed.
- M. Gürü, A. Koca, Ö. Can, C. Çınar and F. Şahin, Biodiesel production from waste chicken fat based sources and evaluation with Mg based additive in a diesel engine, Renewable Energy, 2010, 35, 637–643 CrossRef.
- Technology, I. E. Agency. O. of E., R&D. & (Organization), Energy technology perspectives, International Energy Agency, 2006 Search PubMed.
- T. W. Hudiburg, et al., Impacts of a 32-billion-gallon bioenergy landscape on land and fossil fuel use in the US, Nat. Energy, 2016, 1, 15005 CrossRef.
- P. Chen, et al., The heterogeneous role of energy policies in the energy transition of Asia–Pacific emerging economies, Nat. Energy, 2022, 7, 588–596 CrossRef.
- P. Smith, et al., Essential outcomes for COP26, Global Change Biol., 2022, 28, 1–3 CrossRef PubMed.
- Y. Zhang, L. Duan and H. Esmaeili, A review on biodiesel production using various heterogeneous nanocatalysts: Operation mechanisms and performances, Biomass Bioenergy, 2022, 158, 106356 CrossRef.
- K. Romanak and T. Dixon, CO2 storage guidelines and the science of monitoring: Achieving project success under the California Low Carbon Fuel Standard CCS Protocol and other global regulations, Int. J. Greenhouse Gas Control, 2022, 113, 103523 CrossRef.
- G. Iyer, et al., Measuring progress from nationally determined contributions to mid-century strategies, Nat. Clim. Change, 2017, 7, 871–874 CrossRef.
- M. Lennan and E. Morgera, The Glasgow Climate Conference (COP26), Int. J. Mar. Coast. Law, 2022, 37, 137–151 Search PubMed.
- V. Masson-Delmotte et al., IPCC, 2018: Global Warming of 1.5 C. An IPCC Special Report on the impacts of global warming of 1.5 C above pre-industrial levels and related global greenhouse gas emission pathways, in the context of strengthening the global response to the threat of climate change, sustainable development, and efforts to eradicate poverty, Sustainable Development, and Efforts to Eradicate Poverty, 2018 Search PubMed.
- S. Whitmee, R. Green, J. Phumaphi, H. Clark and A. Haines, Bridging the evidence gap to achieve a healthy, net zero future, Lancet, 2021, 398, 1551–1553 CrossRef.
- N. N. A. N. Yusuf, S. K. Kamarudin and Z. Yaakub, Overview on the current trends in biodiesel production, Energy Convers. Manage., 2011, 52, 2741–2751 CrossRef.
- A. S. Nizami, et al., Waste biorefineries: Enabling circular economies in developing countries, Bioresour. Technol., 2017, 241, 1101–1117 CrossRef.
- B. Karpanai Selvan, et al., Utilization of biodiesel blended fuel in a diesel engine – Combustion engine performance and emission characteristics study, Fuel, 2022, 311, 122621 CrossRef.
- H. Hosseinzadeh-Bandbafha, et al., Biodiesel antioxidants and their impact on the behavior of diesel engines: A comprehensive review, Fuel Process. Technol., 2022, 232, 107264 CrossRef.
- M. A. Hazrat, et al., Techniques to improve the stability of biodiesel: a review, Environ. Chem. Lett., 2021, 19, 2209–2236 CrossRef.
- F. Sundus, M. A. Fazal and H. H. Masjuki, Tribology with biodiesel: A study on enhancing biodiesel stability and its fuel properties, Renewable Sustainable Energy Rev., 2017, 70, 399–412 CrossRef.
- D. Chandran, et al., Critical relationship between biodiesel fuel properties and degradation of fuel delivery materials of a diesel engine, Therm. Sci. Eng. Prog., 2018, 7, 20–26 CrossRef.
- Y. Palani, C. Devarajan, D. Manickam and S. Thanikodi, Performance and emission characteristics of biodiesel-blend in diesel engine: A review, Environ. Eng. Res., 2020, 27, 200338 CrossRef.
- D. Singh, et al., A review on feedstocks, production processes, and yield for different generations of biodiesel, Fuel, 2020, 262, 116553 CrossRef.
- H. Li, et al., Effects of biodiesel blends on the kinetic and thermodynamic parameters of fossil diesel during thermal degradation, Energy Convers. Manage., 2019, 198, 111930 CrossRef.
- E. Symoniuk, K. Ratusz and K. Krygier, Comparison of the oxidative
stability of linseed (Linum usitatissimum L.) oil by pressure differential scanning calorimetry and Rancimat measurements, J. Food Sci. Technol., 2016, 53, 3986–3995 CrossRef.
- F. O. Sanches-Neto, N. D. Coutinho, F. Palazzetti and V. H. Carvalho-Silva, Temperature dependence of rate constants for the H(D) + CH4 reaction in gas and aqueous phase: deformed Transition-State Theory study including quantum tunneling and diffusion effects, Struct. Chem., 2020, 31, 609–617 CrossRef.
- J. Xin, H. Imahara and S. Saka, Kinetics on the oxidation of biodiesel stabilized with antioxidant, Fuel, 2009, 88, 282–286 CrossRef.
- I. M. Rizwanul Fattah, H. H. Masjuki, M. A. Kalam, M. Mofijur and M. J. Abedin, Effect of antioxidant on the performance and emission characteristics of a diesel engine fueled with palm biodiesel blends, Energy Convers. Manage., 2014, 79, 265–272 CrossRef.
- C. A. Moreira, et al., Structural insights and antioxidant analysis of a tri-methoxy chalcone with potential as a diesel-biodiesel blend additive, Fuel Process. Technol., 2022, 227, 107122 CrossRef.
- E. C. M. Faria, et al., Comparative Study of Chalcones and Their Potential as Additives for Biofuels, Energy Fuels, 2021, 35, 552–560 CrossRef.
- G. Hou, X. Yuan, Y. Li, G. Hou and X. C. Liu, a natural chalcone, reduces 5-fluorouracil resistance of gastric cancer cells through targeting Wnt/β-catenin signal pathway, Invest. New Drugs, 2020, 38, 329–339 CrossRef.
- W. Eden, D. Alighiri, N. Wijayati and S. Mursiti, Synthesis of Chalcone Derivative from Clove Leaf Waste as a Natural Antioxidant, Pharm. Chem. J., 2021, 55, 269–274 CrossRef.
- A. Rammohan, J. S. Reddy, G. Sravya, C. N. Rao and G. V. Zyryanov, Chalcone synthesis, properties and medicinal applications: a review, Environ. Chem. Lett., 2020, 18, 433–458 CrossRef.
- S. Farooq and Z. Ngaini, Recent Synthetic Methodologies for Chalcone Synthesis (2013-2018), Curr. Organocatal., 2019, 6, 184–192 CrossRef.
- S. Verma, A. Srivastava and O. Pandey, A Review on Chalcones Synthesis and their Biological Activity, PharmaTutor, 2018, 2, 22–39 CrossRef.
- M. Xu, P. Wu, F. Shen, J. Ji and K. Rakesh, Chalcone derivatives and their antibacterial activities: Current development, Bioorg. Chem., 2019, 91, 103133 CrossRef.
- W. Dan and J. Dai, Recent developments of chalcones as potential antibacterial agents in medicinal chemistry, Eur. J. Med. Chem., 2020, 187, 111980 CrossRef.
- Y. Wang, et al., Synthesis and antibacterial activity of novel chalcone derivatives bearing a coumarin moiety, Chem. Pap., 2019, 73, 2493–2500 CrossRef.
- M. Mellado, et al., Design, synthesis, antifungal activity, and structure–activity relationship studies of chalcones and hybrid dihydrochromane–chalcones, Mol. Diversity, 2020, 24, 603–615 CrossRef PubMed.
- Y. Jin, Recent advances in natural antifungal flavonoids and their derivatives, Bioorg. Med. Chem. Lett., 2019, 29, 126589 CrossRef PubMed.
- K. Goyal, R. Kaur, A. Goyal and R. C. Awasthi, A review on synthesis and pharmacological activities, J. Appl. Pharm. Sci., 2021, 11, 1–14 Search PubMed.
- S. Lahsasni, F. Korbi and N. Aljaber, synthesis, characterization and evaluation of antioxidant activities of some novel chalcones analogues, Chem. Cent. J., 2014, 8, 1–10 CrossRef PubMed.
- C. Zhuang, et al., Chalcone: A Privileged Structure in Medicinal Chemistry, Chem. Rev., 2017, 117, 7762–7810 CrossRef.
- O. Serifi, et al., Antioxidant behaviour of 2′-hydroxy-chalcones: a study of their electrochemical properties, J. Phys. Org. Chem., 2013, 26, 226–231 CrossRef.
- B. Mathew, et al., Anti-oxidant behavior of functionalized chalcone-a combined quantum chemical and crystallographic structural investigation, J. Mol. Struct., 2017, 1146, 301–308 CrossRef.
- N. A. Shakil, M. K. Singh, M. Sathiyendiran, J. Kumar and J. C. Padaria, Microwave synthesis, characterization and bio-efficacy evaluation of novel chalcone based 6-carbethoxy-2-cyclohexen-1-one and 2H-indazol-3-ol derivatives, Eur. J. Med. Chem., 2013, 59, 120–131 CrossRef.
- N. Cotelle, P. Hapiot, J. Pinson, C. Rolando and H. Vézin, Polyphenols Deriving from Chalcones: Investigations of Redox Activities, J. Phys. Chem. B, 2005, 109, 23720–23729 CrossRef CAS PubMed.
- B. P. Bandgar, S. S. Gawande, R. G. Bodade, N. M. Gawande and C. N. Khobragade, Synthesis and biological evaluation of a novel series of pyrazole chalcones as anti-inflammatory, antioxidant and antimicrobial agents, Bioorg. Med. Chem., 2009, 17, 8168–8173 CrossRef CAS.
- L. O. Sallum, et al., Cyclohexanone-Based Chalcones as Alternatives for Fuel Additives, ACS Omega, 2022, 7, 11871–11886 CrossRef CAS.
- K. Burke, Perspective on density functional theory, J. Chem. Phys., 2012, 15, 150901 CrossRef.
- G. H. Grant, W. G. Richards and G. William, Computational chemistry, Oxford University Primers, 1996 Search PubMed.
- D. H. Pereira, F. A. La Porta, R. T. Santiago, D. R. Garcia and T. C. Ramalho, New Perspectives on the Role of Frontier Molecular Orbitals in the Study of Chemical Reactivity: A Review, Revista Virtual de Química, 2016, 8, 425–453 CrossRef.
- M. A. Spackman and D. Jayatilaka, Hirshfeld surface analysis, CrystEngComm, 2009, 11, 19–32 RSC.
- J. Pullen and K. Saeed, Experimental study of the factors affecting the oxidation stability of biodiesel FAME fuels, Fuel Process. Technol., 2014, 125, 223–235 CrossRef.
- Y. Duan, Y. Wang and D. Li, A Facile Approach for Synthesis of Benzofuro[2,3-c ]Pyridines via Intramolecular Cascade Annulations, Chin. J. Chem., 2014, 32, 1103–1106 CrossRef.
- G. M. Sheldrick, SHELXT – Integrated space-group and crystal-structure determination, Acta Crystallogr., Sect. A: Found. Adv., 2015, 71, 3–8 CrossRef PubMed.
- G. M. Sheldrick, Crystal structure refinement with SHELXL, Acta Crystallogr., Sect. C, 2015, 71, 3–8 CrossRef.
- O. V. Dolomanov, L. J. Bourhis, R. J. Gildea, J. A. K. Howard and H. J. Puschmann, OLEX2: a complete structure solution, refinement and general all round good thing Olex2, J. Appl. Crystallogr., 2009, 42, 339–341 CrossRef.
- C. F. Macrae, et al., Mercury : visualization and analysis of crystal structures, J. Appl. Crystallogr., 2006, 39, 453–457 CrossRef CAS.
- A. L. Spek, Single-crystal structure validation with the program PLATON, J. Appl. Crystallogr., 2003, 36, 7–13 CrossRef CAS.
- C. R. Groom, I. J. Bruno, M. P. Lightfoot and S. C. Ward, The Cambridge structural database, Acta Crystallogr., Sect. B: Struct. Sci., Cryst. Eng. Mater., 2016, 72, 171–179 CrossRef CAS.
- J. J. McKinnon, M. A. Spackman and A. S. Mitchell, Novel tools for visualizing and exploring intermolecular interactions in molecular crystals, Acta Crystallogr., Sect. B, 2004, 60, 627–668 CrossRef.
- ANP - Site of the National Agency for Petroleum, Natural Gas and Biofuels, ANP Resolution No. 764 Search PubMed.
- BS EN 15751-14: Automotive Fuels – Fatty acid Methyl Ester (FAME) Fuel and Blends with Diesel Fuel – Determination of Oxidation Stability by Accelerated Oxidation Method, 2014, vol. 22 Search PubMed.
- X. Li, et al., Oxidation degree of soybean oil at induction time point under Rancimat test condition: Theoretical derivation and experimental observation, Food Res. Int., 2019, 120, 756–762 CrossRef CAS PubMed.
- J. Zhou, Y. Xiong and X. Liu, Evaluation of the oxidation stability of biodiesel stabilized with antioxidants using the Rancimat and PDSC methods, Fuel, 2017, 188, 61–68 CrossRef.
- M. Frisch, G. Trucks, H. B. Schlegel, G. Scuseria, M. Robb, J. Cheeseman, G. Scalmani, V. Barone, B. Mennucci and G. Petersson, Gaussian 09, Revision A. 02, Gaussian Inc., Wallingford, CT, 2009, p. 200 Search PubMed.
- Y. Zhao and D. G. Truhlar, The M06 suite of density functionals for main group thermochemistry, thermochemical kinetics, noncovalent interactions, excited states, and transition elements: Two new functionals and systematic testing of four M06-class functionals and 12 other function, Theor. Chem. Acc., 2008, 120, 215–241 Search PubMed.
- R. Krishnan, J. S. Binkley, R. Seeger and J. A. Pople, Self-consistent molecular orbital methods. XX. A basis set for correlated wave functions, J. Chem. Phys., 1980, 72, 650–654 CrossRef.
- A. D. McLean and G. S. Chandler, Contracted Gaussian basis sets for molecular calculations. I. Second row atoms, Z=11–18, J. Chem. Phys., 1980, 72, 5639–5648 CrossRef.
- P. Sjoberg and P. Politzer, Use of the Electrostatic Potential at the Molecular Surface, J. Phys. Chem., 1990, 94, 3959–3961 CrossRef.
- P. López and F. Méndez, Fukui function as a descriptor of the imidazolium protonated cation resonance hybrid structure, Org. Lett., 2004, 6, 1781–1783 CrossRef PubMed.
- J. Melin, P. W. Ayers and J. V. Ortiz, Removing electrons can increase the electron density: a computational study of negative Fukui functions, J. Phys. Chem. A, 2007, 111, 10017–10019 CrossRef PubMed.
- T. Lu and F. Chen, Multiwfn: A multifunctional wavefunction analyzer, J. Comput. Chem., 2012, 33, 580–592 CrossRef.
- F. O. Sanches-Neto, J. R. D. S, L. H. K. Q. Junior and V. H. Carvalho-Silva, ‘pySiR. C. ’: Machine learning combined with molecular fingerprints to predict the reaction rate constant of the radical-based oxidation processes of aqueous organic contaminants, Environ. Sci. Technol., 2021, 55, 12437–12448 CrossRef PubMed.
- L. J. Farrugia, ORTEP-3 for Windows-a version of ORTEP-III with a Graphical User Interface (GUI), J. Appl. Crystallogr., 1997, 30, 565 CrossRef.
- S. J. K. Pond, et al., One- and Two-Photon Spectroscopy of Donor−Acceptor−Donor Distyrylbenzene Derivatives: Effect of Cyano Substitution and Distortion from Planarity, J. Phys. Chem. A, 2002, 106, 11470–11480 CrossRef.
- P. P. Firmino, et al., Synthesis, Molecular Structure, Thermal and Spectroscopic Analysis of a Novel Bromochalcone Derivative with Larvicidal Activity, Crystals, 2022, 12, 440 CrossRef.
- K. Jomová, et al., A Switch between Antioxidant and Prooxidant Properties of the Phenolic Compounds Myricetin, Morin, 3′,4′-Dihydroxyflavone, Taxifolin and 4-Hydroxy-Coumarin in the Presence of Copper(II) Ions: A Spectroscopic, Absorption Titration and DNA Damage Study, Molecules, 2019, 24, 4335 CrossRef.
- S. N. López, et al., vitro antifungal evaluation and structure–activity relationships of a new series of chalcone derivatives and synthetic analogues, with inhibitory properties against polymers of the fungal cell wall, Bioorg. Med. Chem., 2001, 9, 1999–2013 CrossRef.
- Z. Nowakowska, A review of anti-infective and anti-inflammatory chalcones, Eur. J. Med. Chem., 2007, 42, 125–137 CrossRef.
- D. Gupta and D. Jain, Chalcone derivatives as potential antifungal agents: Synthesis, and antifungal activity, J. Adv. Pharm. Technol. Res., 2015, 6, 114 CrossRef.
- M. Leopoldini, T. Marino, N. Russo and M. Toscano, Antioxidant Properties of Phenolic Compounds: H-Atom versus Electron Transfer Mechanism, J. Phys. Chem. A, 2004, 108, 4916–4922 CrossRef.
- I. Lawan, et al., Synthesis, properties and effects of a multi-functional biodiesel fuel additive, Fuel Process. Technol., 2020, 198, 106228 CrossRef.
- R. K. Saluja, V. Kumar and R. Sham, Stability of biodiesel – A review, Renewable Sustainable Energy Rev., 2016, 62, 866–881 CrossRef.
- ANP - Site of the National Agency for Petroleum, Natural Gas and Biofuels, ANP Resolution No. 798/2019, available in: https://www.gov.br/anp/pt-br/assuntos/producao-e-fornecimento-de-biocombustiveis/biodiesel/especificacao-do-biodiesel.
- British Standards Institution, BS EN 590:2013 Automotive fuels Diesel Requirements and test methods, BSI, 2013 Search PubMed.
- G. Joshi, B. Y. Lamba, D. S. Rawat, S. Mallick and K. S. R. Murthy, Evaluation of Additive Effects on Oxidation Stability of Jatropha Curcas Biodiesel Blends with Conventional Diesel Sold at Retail Outlets, Ind. Eng. Chem. Res., 2013, 52, 7586–7592 CrossRef.
- I. M. Rizwanul Fattah, et al., Experimental investigation of performance and regulated emissions of a diesel engine with Calophyllum inophyllum biodiesel blends accompanied by oxidation inhibitors, Energy Convers. Manage., 2014, 83, 232–240 CrossRef.
- A. K. Agarwal, D. Khurana and A. Dhar, Improving oxidation stability of biodiesels derived from Karanja, Neem and Jatropha: step forward in the direction of commercialisation, J. Cleaner Prod., 2015, 107, 646–652 CrossRef.
- W. W. Focke, I. van der Westhuizen and X. Oosthuysen, Biodiesel oxidative stability from Rancimat data, Thermochim. Acta, 2016, 633, 116–121 CrossRef.
- S. Nogales-Delgado, A. Guiberteau and J. M. Encinar, Effect of tert-butylhydroquinone on biodiesel properties during extreme oxidation conditions, Fuel, 2022, 310, 122339 CrossRef.
- K. Varatharajan and D. S. Pushparani, Screening of antioxidant additives for biodiesel fuels, Renewable Sustainable Energy Rev., 2018, 82, 2017–2028 CrossRef.
- G. Karavalakis, D. Karonis and S. Stournas, Evaluation of the Oxidation Stability of Diesel/Biodiesel Blends using the Modified Rancimat Method, SAE Int. J. Fuels Lubr., 2009, 2, 839–849 CrossRef.
- C. Romola, et al., A comprehensive review of the selection of natural and synthetic antioxidants to enhance the oxidative stability of biodiesel, Renewable Sustainable Energy Rev., 2021, 145, 111109 CrossRef.
- G. Zhang and C. B. Musgrave, Comparison of DFT Methods for Molecular Orbital Eigenvalue Calculations, J. Phys. Chem. A, 2007, 111, 1554–1561 CrossRef.
- P. Chinna Babu, N. Sundaraganesan, Ö. Dereli and E. F. T.-I. R. Türkkan, FT-Raman spectra, density functional computations of the vibrational spectra and molecular geometry of butylated hydroxy toluene, Spectrochim. Acta, Part A, 2011, 79, 562–569 CrossRef.
- R. Raja, S. Seshadri, T. Gnanasambandan and R. R. Saravanan, Crystal growth and properties of NLO optical crystal – Butylated Hydroxy Toluene (BHT), Spectrochim. Acta, Part A, 2015, 138, 13–20 CrossRef PubMed.
- V. Krishna Kumar, S. Suganya and R. Mathammal, Molecular structure, vibrational spectra, HOMO, LUMO and NMR studies of 2,3,4,5,6-Penta Bromo Toluene and Bromo Durene based on density functional calculations, Spectrochim. Acta, Part A, 2014, 125, 201–210 CrossRef.
- A. S. Rad, Al-doped graphene as modified nanostructure sensor for some ether molecules: Ab-initio study, Synth. Met., 2015, 209, 419–425 CrossRef.
- H. Mohammed, S. Attia, M. Nessim, M. Shaaban and A. El-Bassoussi, Studies on Some Thiazolidinones as Antioxidants for Local Base Oil, Egypt. J. Chem., 2019, 62, 1219–1234 Search PubMed.
- M. A. Diab, A. Z. El-Sonbati, N. A. El-Ghamaz, Sh. M. Morgan and O. El-Shahat, Conducting polymers X: Dielectric constant, conduction mechanism and correlation between theoretical parameters and electrical conductivity of poly (N,N′-bis-sulphinyl p-phenylenediamine-2,6-diaminopyridine) and poly (N,N′-bis-sulphinyl p-phenylenediamine, Eur. Polym. J., 2019, 115, 268–281 CrossRef.
- S. Yang, C. Ye, X. Song, L. He and F. Liao, Theoretical calculation based synthesis of a poly(p-phenylenediamine)–Fe 3 O 4 composite: a magnetically recyclable photocatalyst with high selectivity for acid dyes, RSC Adv., 2014, 4, 54810–54818 RSC.
- D. A. Milenković, et al., Advanced oxidation process of coumarins by hydroxyl radical: Towards the new mechanism leading to less toxic products, Chem. Eng. J., 2020, 395, 124971 CrossRef.
- F. O. Sanches-Neto, B. Ramos, A. M. Lastre-acosta, A. C. S. C. Teixeira and V. H. Carvalho-Silva, Aqueous Picloram Degradation by Hydroxyl Radicals: Unveiling Mechanism, Kinetics, and Ecotoxicity through Experimental and Theoretical Approaches, Chemosphere, 2021, 130401, DOI:10.1016/j.chemosphere.2021.130401.
|
This journal is © The Royal Society of Chemistry 2022 |
Click here to see how this site uses Cookies. View our privacy policy here.