DOI:
10.1039/D1RE00074H
(Review Article)
React. Chem. Eng., 2022,
7, 9-40
The advanced synthetic modifications and applications of multifunctional PAMAM dendritic composites
Received
19th February 2021
, Accepted 24th October 2021
First published on 25th October 2021
Abstract
The profound advances in dendrimer chemistry have led to new horizons in polymer science. Moreover, this has promisingly uncovered opportunities to develop new versatile polymers in the form of poly(amidoamine) dendrimer hybrids to utilize their intrinsic distinctive properties. The unique three-dimensional globular shape, highly branched structure, monodispersity, controlled topology, nanoarchitecture, and massive number of cavities have spurred polymer researchers to construct new poly(amidoamine) dendrimer composites using organic and inorganic materials. Thus, to meet the prospective needs of modern scientific research, significant efforts have been made to functionalize PAMAM, encompassing applications in the biomedical and non-biomedical areas. This review article attempts to consolidate the research work to date relating to the synthesis of organic/inorganic hybrids of poly(amidoamine) dendrimers and their potential applications in the biomedical field, controlled agrochemical release, catalysis, wastewater treatment, imaging, biosensors, etc.
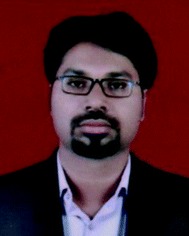 Ramkrishna Y. Patle | Mr. Ramkrishna Y. Patle has over 7 years of teaching experience. He has experience as an officiating Principal for the session 2014–15 and is now working as an Assistant Professor and Head of the Chemistry department at Mahatma Gandhi College of Science, Gadchandur, Chandrapur (M.S.). He completed Post Graduate studies in Chemistry at the Department of Chemistry, Post Graduate Teaching Department, Mahatma Jyotiba Phuley Campus, R.T.M. Nagpur University, Nagpur. He is pursuing a PhD under the supervision of Prof. (Mrs.) J. S. Meshram from the Department of Chemistry, RTM Nagpur University, Nagpur. He has published a book entitled “A Textbook of Organic Chemistry” in 2020 and two research articles in international journals. He is working on PAMAM dendrimers and their applications. |
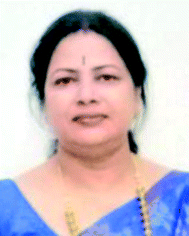 Jyotsna S. Meshram | Prof. (Dr.) Jyotsna S. Meshram serves as Professor and Head in the Post Graduate Teaching Department of Chemistry, Rashtrasant Tukadoji Maharaj Nagpur University, Nagpur. She has more than 27 years of teaching and research experience. She has been actively involved in various research projects, national and international conferences, and invited lectures in India and abroad. She works in areas like green chemistry, organic chemistry, medicinal chemistry, and dendrimers. She has many publications in reputed national and international journals. To date, under her supervision, 19 students have been awarded PhDs and six students have been awarded MPhils. At present, six students are currently working under her supervision and one student has submitted their PhD thesis. She is a reviewer and an editor of various international journals. |
Introduction
Polymer chemistry has been studied for a long time but no one speculated that the discovery of an incredible tree-like molecule was about to change the direction of the research in polymer science. Flory introduced a three-dimensional structured polymer which has a range of molecular sizes in systems.1 The synthesis of highly branched polymers was made possible by reacting a monomer with one functional group with two or more other reactants.2 Between 1978 and 1985, the “repeating-step principle” and “time sequence propagation” technique gave birth to new topological Starburst polymers. In 1978, Vogtle and his coworkers first reported the “repeating-step principle” and constructed the first cascade of non-cyclic and non-skid-chain-like cyclic polyaza compounds.3 This was followed closely and independently by the “time-sequenced propagation” technique. The new topological dendritic architecture was prepared in the Dow Company between 1979 and 1985 by D. A. Tomalia and coworkers.4 In 1985, this star dendritic polymer was first named Starburst dendrimer. It is also called poly(amidoamine) because it has several amide and amine functional groups.5 Dendrimers are three-dimensional macromolecules characterized by a three-dimensional nanoscale architecture with a globular shape, highly branched, monodispersed, low polydispersity, high functionality, symmetric, and vast numbers of cavities.6 The three-dimensional structure of the PAMAM dendrimer generation 2.0 is shown in Fig. 1. This nanostructured dendritic polymer was perhaps one of the most elite evolutionary molecules optimized to manifest the interfaces between the earliest and modern polymeric chemistry, which realigned the research in a seminal perspective in polymer science.7 Around the same time, Newkome et al. independently reported the synthesis of tree-like monocascade spheres called ‘arborols,’ with polar groups facing outward in three-dimensional space, which was related to micelle approaches.8
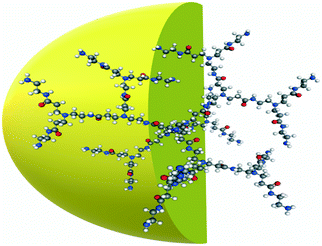 |
| Fig. 1 A schematic representation of the 3D structure of the PAMAM dendrimer G 2.0. | |
Due to their unique properties, dendrimers have emerged in the form of star-shaped molecules, and they have potential future applications in various fields. Now, several dendrimers are commercialized in the market, such as poly(amidoamine) (PAMAM), polyethylene oxide (PEO), polypropylene imine (PPI), polyethyleneimine (PEI), and polyethylene glycol (PEG).9 The Starburst dendrimers have been explored substantially in various fields, such as biomedical,10 optical sensing,11 nanocarriers,12 drug delivery systems,13 gene therapy,14 imaging and contrast agents,15 catalysis,16 and waste water remediation.17 The numerous applications of the PAMAM dendrimer stimulated the synthesis of discrete organic and inorganic hybrids with the PAMAM dendrimer based on their distinct properties. In this review article, we discuss all the aspects regarding the progressive development in organic/inorganic hybrids with the PAMAM dendrimer and their many applications in diverse areas. The article emphasizes the advances in the synthesis of dendrimers and their relevant applications in various fields to meet the needs of mankind. In this article, a detailed description is provided of the advanced synthetic approaches to synthesize the numerous inorganic and organic hybrids of the dendrimer.
Traditional synthetic approaches
The precise synthetic approach to prepare a new molecule should be reflected in the yield of the product obtained. The constructive strategies to obtain a high yield are very important in synthesis.
Several methodologies have been developed to prepare dendrimers. The major approaches for synthesizing dendrimers can be categorized into two methodologies, viz. divergent and convergent methods.18
Divergent method
Dendrimer synthesis starts from the interior core to the outward periphery. The divergent strategy is based on the iterative repeating-step technique, which involves the repeated step-wise coupling of reactants in large excess. The divergent methodology was first employed in the preparation of cascade molecules and then developed by Tomalia and Newkome to prepare high-molecular-weight dendrimers.9 One of the best examples of divergent synthesis is poly(amidoamine) (PAMAM) dendrimer with an ethylenediamine (EDA) core, which was synthesized by Tomalia et al. through two iterative steps, i.e., Michael addition of amines to methyl acrylate followed by amidation of the resulting methyl ester with a large excess.18 Divergent growth proceeds with the repetitive coupling of monomers and activation steps. The core is accompanied by reactive end groups. The monomer molecules are added to the peripheral end functionalities of the core molecules and a new generation is obtained with new functionalities. This gives the branching sites on the core. This step is named the coupling step. The coupling step is followed by the activation step. In the activation step, the end groups on the monomers are transformed or deprotected into other reactive functionalities.19
The joining of monomers to the core molecule and the activation of the peripheral functionalities on the monomer result in new generations of dendrimer.20,21 However, this is facilitated by the use of large excess of reagents/reactants in each step and thus the reaction goes to completion. The repetition of both the steps results in the formation of the new layer of functionalities, which gives a new generation on each repetition (Fig. 2).22,23 The main advantage of the divergent method is its speed of the formation of various generations of dendrimer. Furthermore, the reaction can be stopped at any step. Dendrimers synthesized by the divergent method are obtained in a high yield. This method enables the preparation of high-molecular-weight dendrimers. Thus, it is possible to obtain higher-generation dendrimers with ease with this reaction. However, one thing must be noted: the purity of the dendrimer decreases as the generation increases due to unexpected side reactions occurring during the synthesis. Divergently prepared dendrimers are impractical to purify, which might be due to structural similarities between the by-product and the main product.24–28
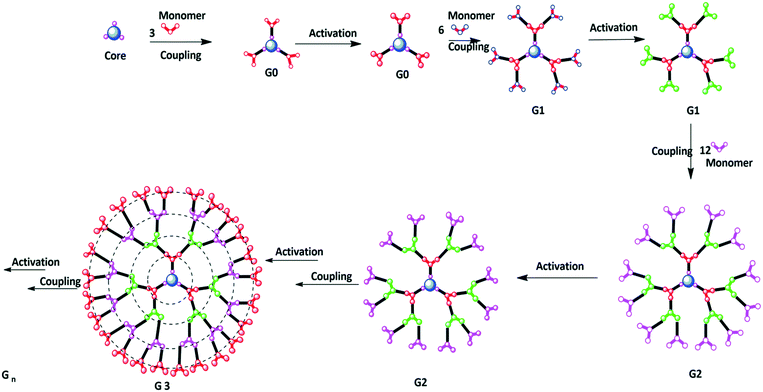 |
| Fig. 2 A schematic representation of dendrimer synthesis by a divergent approach. | |
Convergent approach
In dendrimer synthesis, the convergent approach is a good alternative method. To synthesize lower-molecular-weight dendrimers, the convergent approach is supposed to be one of the best methods to be implemented. This method was first pioneered by Fréchet and Hawker in 1989–1990.29 Parallel to the divergent method, it is also considered a milestone in the field of dendrimer chemistry. The controlled topology in the molecule, purity of the product, and very few reactive steps are the main aspects of the convergent synthesis. One need not consider the higher yield of the product if one is interested in the purity of the dendrimer. Many articles have been published depicting the synthetic aspects of the divergent method. The convergent approach is a versatile tool and an alternative to the divergent approach.23 The convergent approach is based on synthesis from the periphery to the core. The structural aspects of the periphery are supposed to be predetermined and the synthesis is started by coupling surface functionalities to the monomer units. In the convergent synthesis of dendrimers, the predetermined molecular structure has surface functionality (Sf) as well as a reactive functional group (Fr). This molecular structure is coupled to the monomer, which includes a protected functional group (Fp) and two reactive sites. The coupling is then followed by the activation of the protected functional group. After activation, the protected functional group is converted to the reactive functional group. The resultant moiety is again condensed to the fresh monomer molecule. These sequential steps are repeated until the formation of dendritic wedges called dendrons. The dendrons have one reactive functional group. The resultant dendrons are then coupled to the polyfunctional core molecule through the focal point. The final product obtained is then characterized as a dendrimer. Thus, the convergent approach is based on the iterative synthesis of dendrons, which are finally coupled to the suitable core molecule.29,30 This approach of outward to inward synthesis of dendrimers is called the convergent approach and is illustrated in Fig. 3.
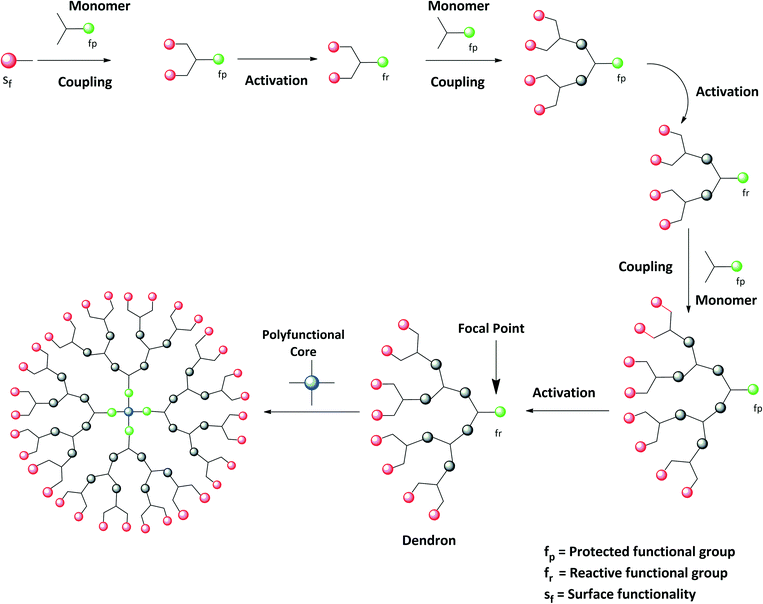 |
| Fig. 3 A schematic representation of dendrimer synthesis by a convergent approach. | |
In contrast to divergent synthesis, there are very few changes required to complete the reactions. Thus, one need not use large amounts of reagents to carry out the synthesis. There is functional versatility in the convergent method. The dendrons can be modified at their focal point in the growth steps to obtain different dendrimers.31 However, one consider the practicality for commercialization of the dendrimers. To date, many kinds of dendrimers have been commercialized using the major practical applicability of the divergent method. Thus, it can be said that although the convergent method has unique applicability in synthetic chemistry, it is not suitable for commercial applications. The extensive use of the convergent synthetic method enabled the preparation of internally modified PAMAM dendrimers,32 GalNAc conjugated dendrimers,33 carbohydrate dendrimers,34,37 three-component reaction dendrimers,35 monodisperse dendrimers based on the 1,3,5-trisubstituted benzene,36,38 platinum–acetylide dendrimers,39 symmetrical and unsymmetrical dendrimers,40 azide-functionalized PAMAM dendrons,41 siloxane dendrimers,42etc.
Advanced synthetic approaches
Dendrimer chemistry did not stop with the traditional synthetic approaches, i.e., divergent and convergent synthesis. Over the years, several methods have been developed to synthesize dendrimers. One might rely upon the divergent and convergent methods, but as requirements develop, new methodology has been developed. Thus, groundbreaking advances have taken place in dendrimer synthesis. In this review article, we will briefly look at some of the other important synthetic approaches.
Double-stage convergent method
In addition to the traditional synthesis of dendrimers, some other methods have been devised for fast construction of higher-molecular-weight, monodispersed, globular dendrimers with more potent functionalities at the periphery. The amalgamation of the convergent and divergent methods was found to be useful for fabricating more functional dendrimers.20,23,24 This method is known as a double-stage convergent method. In this method, one part involves the construction of a monodisperse macromolecule with a number of surface functional groups by the divergent method, which is called the hyper core, and the synthesis of dendronic monomers by the convergent method. In the other part, the convergent dendrons are coupled at their focal point to the multifunctional hyper core to prepare big globular dendritic macromolecules.43,45 Large structures can be obtained while keeping the reaction steps to the minimum. There is huge scope for researchers to obtain more tailored and functional dendrimers. The synthesis of phenylacetylene dendrimers of the fourth generation,44 dendritic polyethers,45,46 functionalized dendritic aliphatic polyesters based on 2,2-bis(hydroxymethyl)propionic acid,47 aramid dendrons,48 and amphiphilic dendrons (G1–G4)49 has been carried out.
Double exponential method
The “double exponential growth” method was first implemented by Moore et al.50 The concept has emerged as one of the synthetic methods for the construction of dendrimers with exponential growth.23,24 In this method, the dendrimer generation is doubled exponentially. The method employs the fully protected trifunctional starting material of AB2 type for the synthesis of monomer units for convergent and divergent dendrimers. One should be familiar with the chemistry of protection and deprotection. The starting molecule is composed of three branching units—two branching sites for coupling Bc, and one functional group unit Af as a focal point. The double exponential growth proceeds with the selective deprotection of the two coupling sites Bc in one molecule with deprotection of functional group Af in another molecule and coupling of activated branching units at the focal point to two coupling sites of another molecule to obtain the second-generation monodendrons. These sequential steps are repeated to get the fourth-generation dendronic monomer.50,51 A schematic representation of the double exponential growth method is given in Fig. 4. This method was employed by Frechet et al.,52 Sharpless,53 and Zanini and Roy54 in the preparation of dendrimers.
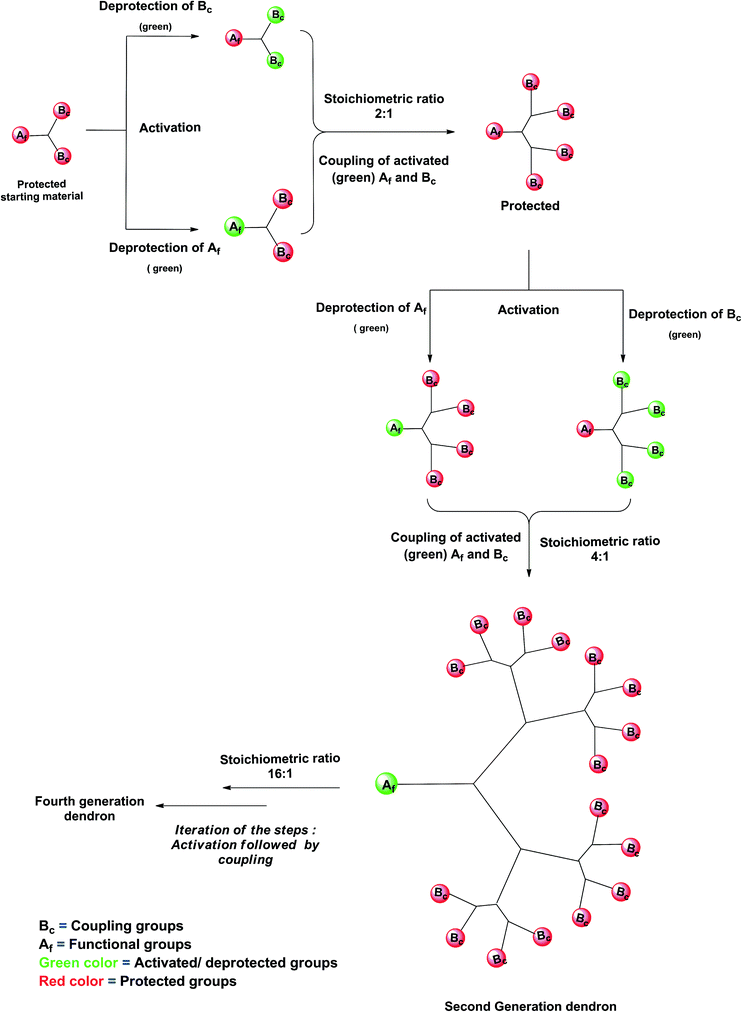 |
| Fig. 4 A schematic representation of the double exponential growth method. | |
Solid-phase synthesis
Solid-phase synthesis started when peptides were synthesized using a solid support by Merrifield.55 Solid-phase synthesis usually includes the polymeric material attached to solid beads as a support using the linker X. In general, the substrate A is attached to the functional group F (e.g., –NH2) (already fixed to the polymeric support) through a linker. The reaction proceeds with the iterative addition of the new substrates B, C, and so on via a linker. But the reaction has to stop soon after the addition of substrate B, the covalent bond undergoes hydrolytic cleavage.
In dendrimer synthesis, the initiator core is permanently attached to the solid support (e.g., polyamine), the monomer is attached to the initiator core via a linker (core–linker conjugate). Thus, repeating addition of the monomer gives large dendritic molecules.56–58 In the recent trend, commercially available polystyrene resin beads have been used as the polymeric material for the solid support in dendrimer synthesis. Generally, in the solid-phase synthesis, the reagents need to be in large excess to get the best yield of the product. Thus, the impurities present in the product are difficult to separate, and the cost for recycling the solid support is quite high. Solid-phase synthesis is represented schematically in Fig. 5.
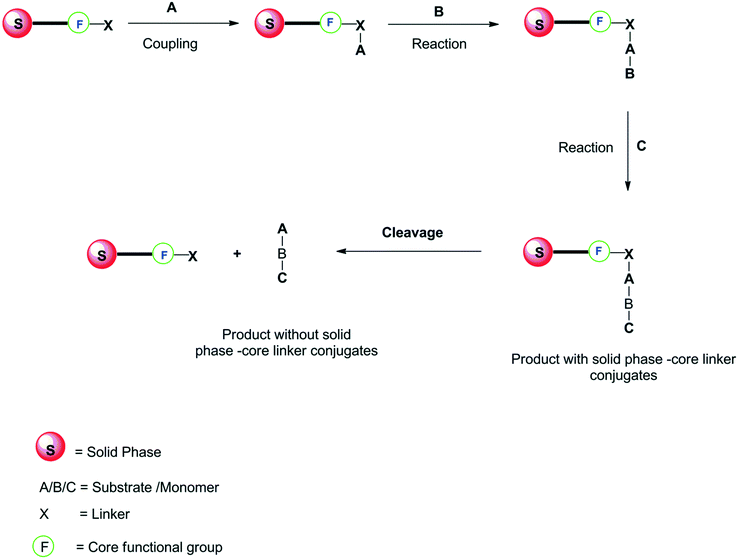 |
| Fig. 5 A schematic representation of solid phase synthesis. | |
The solid-phase synthesis method has been employed in the preparation of PAMAM dendrimers,56,58 peptide dendrimers,57 aryl-ether dendrimers,59 second-generation polyproline dendrimers,60etc.
Orthogonal synthesis
The concept of orthogonal synthesis of dendrimers was first implemented by Spindler and Frechet to synthesize the regular poly(ether-urethane) dendrimer by selecting the AB2 and CD2 monomers 3,5-diisocyanatobenzyl chloride and 3,5-dihydroxybenzyl alcohol, respectively.61 Thereafter, this strategy became one of the alternative synthetic methods for synthesizing lower and higher-generation dendrimers (Fig. 6).
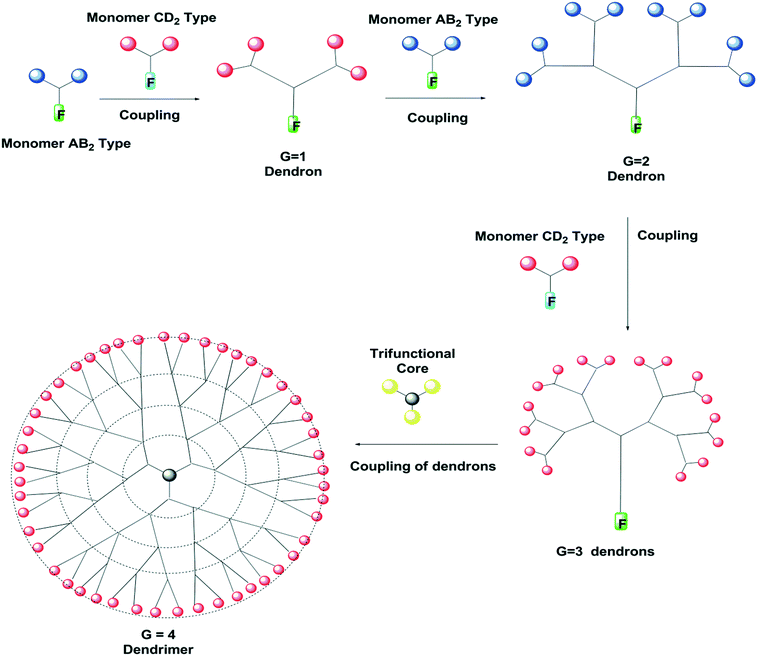 |
| Fig. 6 A schematic representation of the convergent orthogonal synthesis of dendrimers. | |
In this method, first two chemoselective AB2 and CD2 monomers need to be selected. The selection of the monomer should be done so that it contains the functional group at the focal point that must react with the peripheral groups of another monomer. This approach reduces the reaction and purification steps and does not require activation steps. The focal point functional group Af of the AB2 reacting monomer couples with the peripheral groups D of the CD2 monomer and vice versa. These synthetic reaction steps add the layer of the new generation each time. Orthogonal synthesis proceeds either divergently or convergently.23,30,62–65 Using orthogonal synthesis, the lower generation G3 poly(ether-urethane) dendron was first synthesized by Spindler and Frechet61 and the higher-generation G4 poly(alkyne ester) dendrons were synthesized by Zimmerman et al.66 Yu et al. were first to report the synthesis of homogenous dendrons67 and the construction of nonlinear optical (NLO) dendrimers was developed after this.68
Click chemistry
The concept of assembling small building blocks of molecular frames in one pot is one of the finest breakthroughs in the field of chemistry. This modern synthetic strategy69 has been developed to give stereo-specific macromolecules, high yields up to 100%, and an easily separable product that requires little effort for the purification, providing synthesis of the macromolecular frames in a short time. This has enabled researcher to implement this chemistry for small- and large-scale production. The molecular blocks are joined together through the heteroatoms via C–X–C linkage.
This approach is called click chemistry and was introduced by Sharpless et al.70–74 This novel concept was implemented for dendrimer synthesis by coupling the small portions together to obtain a large molecule.23
Fokin et al. were the first to implement the click chemistry route for the synthesis of dendrimers. The triazole dendrimer was prepared by coupling of azides and alkynes and catalyzed by Cu(I) with a high yield and excellent purity.75,76 Later, many researchers have synthesized dendrimers using the click chemistry route, making it an unsurpassed alternative synthetic strategy for modern methods. The highly efficient synthesis of multivalent unsymmetrical dendrimers containing mannose-binding units and coumarin fluorescent units,77 the synthesis of Ru(II)–carbohydrate dendrimers,78 and the synthesis of polyglycerol- and porphyrin-core dendrimers79 are some of the significant prominent results achieved using click chemistry. Click chemistry is widely studied for use in Cu(I) catalyzed azide–alkyne cycloaddition (CuAAC), Diels–Alder cycloaddition, thiol-yne click reactions, etc.22,80 In this article, we summarize some of the methods to elaborate the concepts of the click reactions to witness the actual chemistry involved in the synthesis of dendrimers.
Cu(I)-Catalyzed azide–alkyne cycloaddition (CuAAC) reactions.
CuAAC reactions are a kind of Huisgen 1,3-dipolar cycloaddition of azides and terminal alkynes. The CuAAAc reaction is characterized by the regioselectivity in the product, which involves the condensation of the organic azide molecules with terminal alkynes in the presence of the Cu(I) catalyst to yield regioisomeric 1,4-disubstituted 1,2,3-triazoles. The general scheme for the formation of triazole heterocycles is shown in Fig. 7.81–83
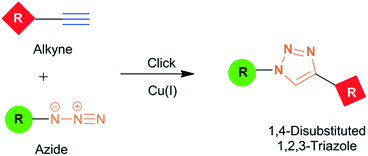 |
| Fig. 7 Cu(I) assisted Huisgen [3 + 2] type cycloaddition of alkyne and azide to form 1,4-disubstituted 1,2,3-triazole heterocycle from alkyne and azide. Reproduced from ref. 81 with permission of Royal Society of Chemistry, copyright 2008. | |
Agrahari et al. in their article have demonstrated the significance of the CuAAC reaction to generate a new dendritic architecture. It offers the design of dendrimers but can also be used to modify the periphery of the dendrimers. The CuAAC click reactions involve the linking of dendrons with acetylene and organic azides as terminal moieties. Fig. 8 shows the click reaction between the dendrimer core 18 with acetylene as the terminal moiety and a galactosylated dendron with an azide moiety. In the reaction, six molecules of azide dendron attach to the dendrimer core 18, forming a thiazole ring, and finally the galactosylated dendrimer is obtained.84
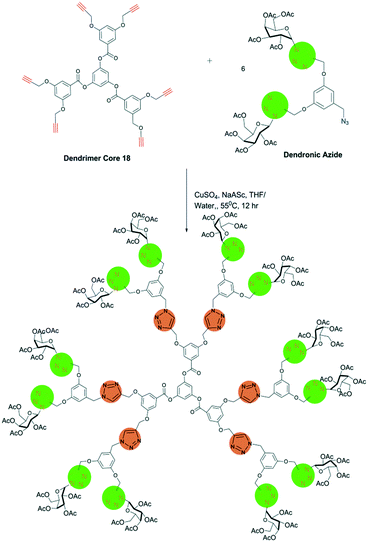 |
| Fig. 8 Synthesis of galactosylated dendrimer using CuAAC click reaction. Reproduced from ref. 84 with permission from Royal Society of Chemistry, copyright 2020. | |
Diels–Alder click reactions.
The Diels–Alder reaction is a simple [4 + 2] cycloaddition reaction between an electron-rich diene and an electron-deficient dienophile that creates a stable adduct. With the increasing interest in the field and to enhance the selectivity, versatility, productivity, and purity of the product, click reactions have been developed. The basic mechanism of the Diels–Alder and retro DA reaction is schematically represented in Fig. 9.
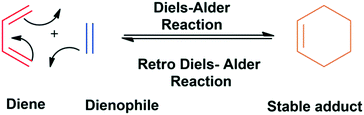 |
| Fig. 9 A schematic representation of the mechanism of Diels–Alder/retro Diels–Alder reactions. | |
The Diels–Alder cycloaddition click chemistry involves the cohesion of molecules with diene and dienophile groups to form the adduct. This methodology allows the construction of a big framework from small molecules in a very simple way. The molecules fulfilling the criteria of the Diels–Alder cycloaddition reaction give a stable adduct. This concept has been utilized in the synthesis of dendrimers of different categories. The development of the Diels–Alder click reaction concept was a milestone in the generation of large multifunctional polymers with potential applications in various fields. It has been used to construct dendritic polymer hybrids that have shown remarkable potential for application in different areas of science. The efficient and reliable click methodology has marked its importance in the designing of large dendritic polymers, dendritic conjugates, and hybrids (Fig. 10).81,85,86
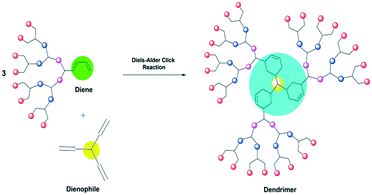 |
| Fig. 10 A schematic representation of the Diels–Alder click reaction in dendrimer synthesis. | |
For example, Sanyal et al.87 have explored the Diels–Alder click reaction to design segment block dendrimers. They used dendrons containing a furan moiety as the diene and maleimide as the dienophile. The D–A click reaction generates two bicycloadduct isomers of as products, i.e., the exo and endo isomers.
The furan group of the diene and the maleimide group of the dienophile exist at the focal point and hence the dendrimer produced consists of a furan–maleimide bicycloadduct as the core of the dendrimer. A schematic representation of the formation of the segment block dendrimer containing a furan–maleimide bicycloadduct core is shown in Fig. 11.87
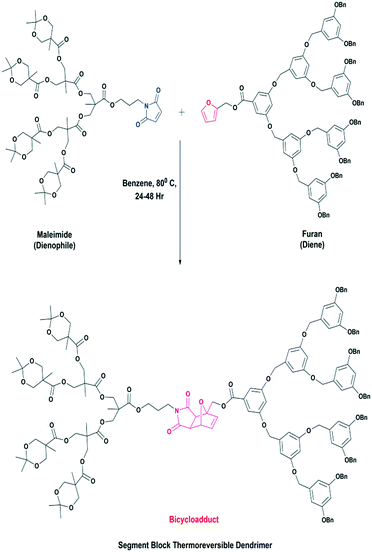 |
| Fig. 11 A schematic representation of the formation of a segment block dendrimer containing a furan–maleimide bicycloadduct core. Reproduced with permission from ref. 87. Copyright 2008 American Chemical Society. | |
Thiol-ene click reactions.
Polymer chemistry has acquainted me with the new concept of click chemistry to design complex molecules. This methodology is based on the reaction of a thiol moiety and an alkene to generate the next molecule of interest. Thiol-ene reactions in base and radical forms are considered to be the most promising, commanding and versatile methods to construct complex molecular frameworks, tailoring the peripheral functionalities and conjugating new functional groups to the molecular scaffold. The formation of complex molecules via the thiol-ene click method is illustrated schematically in Fig. 12.88
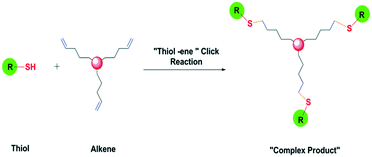 |
| Fig. 12 A schematic representation of the general mechanism of the thiol-ene click reaction. | |
The product obtained in the radical thiol-ene reaction initiated by photo-irradiation was found to be advantageous in terms of high yield, fast reaction rate and rapid conversion. This unique photo-initiation ability of the radical thiol-ene reaction method has made the click reaction more potent and promising (Fig. 13).72
 |
| Fig. 13 The synthesis of the [G4]-(OH)48 dendrimer and its modification using the thiol–ene reaction. Reproduced with permission from ref. 63. Copyright 2008 American Chemical Society. | |
Killops et al. elaborated the synthetic applicability of the thiol-ene click reaction in a better way by describing the synthesis of the [G4]-(OH)48 dendrimer and its modification. The synthesis was initiated by the photo-initiator 2,2-dimethoxy-2-phenylacetophenone with the starting materials of 2,4,6-triallyloxy-1,3,5-triazine as the tris-alkene core and 1-thioglycerol as the monomer. The reaction was carried out at room temperature with UV irradiation. The yield was excellent and it was a solvent-free thiol-ene click reaction.63 Thus, increasing complexity of the molecules and synthesis processes can be achieved with ease now and made feasible by thiol-ene click reactions.
Moreover, the advances in the synthetic methods for dendrimers have disclosed new more classes of hybrid dendrimers. Some of them have been commercialized in the market, fulfilling global demand. Some important dendrimers have been discovered, such as PPI dendrimers,89 PAMAM dendrimers,4,5 glycodendrimers,90 porphyrin dendrimers,91 metallodendrimers,92 polyether dendrimers,93 hybrid dendrimers,94 tacto dendrimers,95 siloxanes, and carbosiloxane dendrimers,96 peptide dendrimers,97 and chiral dendrimers.98
One-pot synthesis method
The synthesis of the hyperbranched dendritic architecture requires multi-step reactions. To lessen the purification steps and reduce the reaction time, one of the better choices is the one-pot synthesis method. The one-pot multi-step reaction can be classified into two important categories, namely non-tandem reactions (NTRs) and tandem reactions (TRs).24
In the NTRs approach, a series of chemical reactions occurs in a consecutively controlled way, i.e., one at a time, while in the TRs approach, the reactions are independent of each other and occur simultaneously. A schematic representation of the tandem and non-tandem approaches is shown in Fig. 14.24,99 In dendrimer synthesis, some parallel side reactions proceed, which results in the formation of the low-molecular-weight dendrons as a by-product. Thus, the purity of the dendrimer is compromised. To avoid this type of nuisance in the synthesis, the one-pot approach is found to be one of the only strategies that furnishes the desired product with considerable purity. One-pot synthesis can be used in the synthesis of dendrimers as well as post-modification of the dendritic framework.100
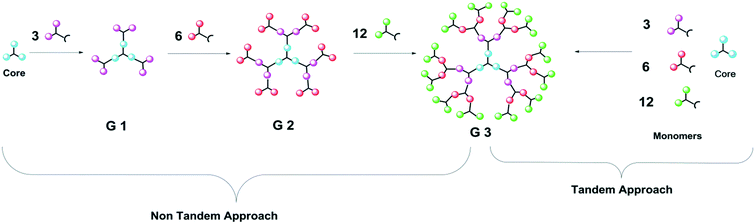 |
| Fig. 14 A schematic representation of tandem and non-tandem approaches. | |
For example, Virboul et al. modified a polycationic dendrimer with sulfonate-tethered NHC complexes. By using a one-pot strategy, the NHC complex was anchored non-covalently to the polycation dendrimer. The one-pot procedure was successfully used in the preparation of the NLO dendrimer of the fourth generation. The dendrimer was synthesized in two one-pot procedures and the yield obtained was 49.2%.101
Recently, to evaluate the efficiency and applicability of the one-pot synthesis approach, Garcia-Gallego et al. synthesized the fourth-generation dendrimer from the bis-MPA monomer. It was constructed using the tris(2-aminoethyl)amine (TREN) route through a non-tandem approach. High monodispersity and an excellent production yield was obtained in a very short period of time (2 h 20 min). The synthetic route is shown in Fig. 15(b).102
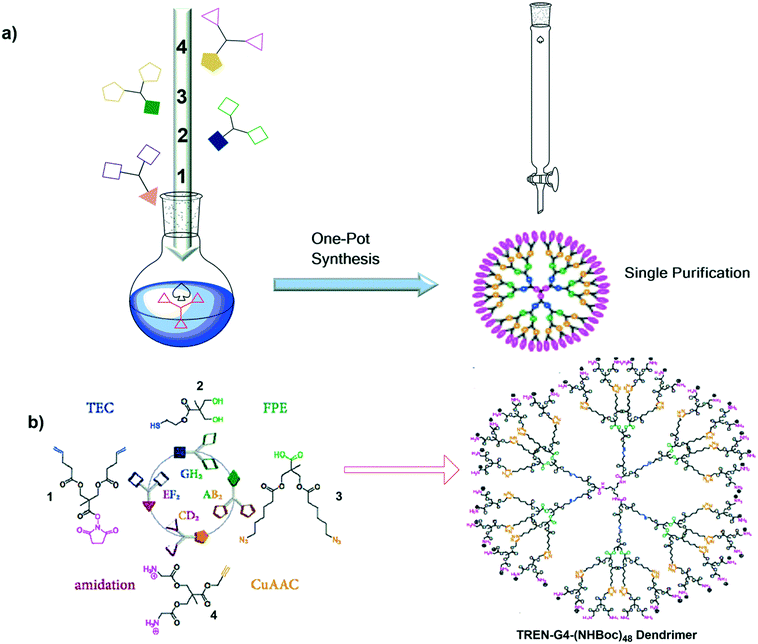 |
| Fig. 15 a) One-pot synthesis and b) a schematic representation of the formation of the TREN-G4-(NHBoc)48 dendrimer using the TREN route through a non-tandem reaction approach. Reproduced with permission from ref. 102. Copyright 2020 American Chemical Society. | |
Purification of dendrimers
There is very little literature available on the purification of dendrimers. However, it is very important for a chemist to be comfortable with the purification methods. In the synthesis of dendrimers, each sequential step requires the removal of defective dendritic molecules from the product. Therefore, before the synthesis of the next generation it becomes very essential to check the purity of the product obtained. The dendrimer synthesis is accompanied by the yield of undesired dendritic molecules, which might be the consequence of the non-ideal dendritic growth. This could be attributed to the incomplete Michael addition reaction, retro-Michael reaction, intramolecular cyclization and solvolysis of peripheral functionalities. This results in the polydispersity of the dendrimer, which affects the properties of the dendrimer.5
Most commonly, the divergent method has been applied for the synthesis of dendrimers. To obtain the monodisperse dendrimer, excess reagent is added to prevent the non-ideal growth of dendrimers. In the synthesis of the PAMAM dendrimer, an excess of EDA dissolved in methanol is added to the reaction mixture to stop the intramolecular cyclization and incomplete Michael addition reactions. The excess methanol and methyl acrylate volatiles are removed by rotary evaporator under reduced pressure and the excess of the EDA is eradicated by 9
:
1 toluene–methanol azeotropic distillation. The leftover toluene is eliminated by azeotropic distillation with methanol and the remaining methanol is removed by rotary evaporator under reduced pressure. In the course of the procedure, the temperature of the water bath is maintained at 30 °C. The procedure is repeated until a viscous yellow oily product is obtained.103–105
An advanced method of purification was introduced by Rundel et al. for the purification of half-generation ester-terminated PAMAM dendrimers. In this technique, a polyimide nanofiltration membrane (STARMEM 122) was employed for purification, permitting the microfluidic-based non-stop pressure-driven purification of the ester-terminated dendrimer. This technique has the advantage of not requiring additional buffers and solvents.106 Parallel to this method, the dialysis membrane technology has another advantage for dendrimer purification. It minimizes the polydispersity and enhances the number-average molecular weight (NAMW) of the polymeric materials. Mullen et al. used this method to purify the G5 PAMAM dendrimer. The methanol was removed under reduced pressure and the G5 dendrimer was lyophilized and obtained in dry form. Aliquots of the dendrimer were subjected to the dialysis through a 10
000 MWCO membrane of size 14 inches accompanied with dialysis tubing sealed at both ends. The dendrimer underwent exchange with nanopure water 9 times over 3 days. After the purification was completed, the dendrimer was collected.107
The trailing/defective fragments need to be separated from the product. After the removal of the excess solvent and reagents under vacuum, the product is purified by column chromatography using a suitable mobile phase, typically a mixture of polar and non-polar solvents in varying proportions. The proportions are varied according to the nature of the product. A suitable mobile phase can be chosen by performing thin-layer chromatography, which helps to identify the required solvent system. Mobile phases that might be used for the column chromatography include chloroform and methanol (4
:
1), dichloromethane and methanol (9
:
1), etc. After the elution of the product, the solvent is evaporated by rotary evaporator and the purified product is collected.108
Characterization
Many superior techniques have been developed and improved to explore the various structural aspects and properties of dendrimers. The scientific advances in dendrimer chemistry simultaneously provoked the improvement of dendrimer characterization techniques. The introduction of a novel molecule into the scientific database needs the accurate structural elucidation of the molecule. Moreover, many spectroscopic techniques have been developed to envisage the structure and properties of dendrimers. Different analytical tools and techniques are used for dendrimer characterization, including spectroscopic techniques, microscopy, scattering techniques, chromatography, and electro-analytical techniques.109,110
The synthesized dendrimers are evaluated for various characteristic properties of the molecule to satisfy the scientific outlook. Certain parameters that support the structural elucidation of the molecule are:
1. Chemical composition
2. Molar mass
3. Dimensions
4. Structural defects
5. Internal groups and end groups
6. Structure
The above parameters can be determined using several combinations of spectroscopic, microscopic, chromatographic, and electro-analytical techniques. The important techniques for characterization are summarized in Table 1.
Table 1 Techniques employed for dendrimer characterization
a. Spectroscopic techniques |
Technique |
Benefits |
Ref. |
UV-vis spectroscopy |
Detection of the conjugates and functional moieties present |
111–112
|
Infra-red spectroscopy |
Detection of the internal and end functional groups present |
112–114
|
NMR spectroscopy |
Elucidation of the structure and dynamics of molecules in solution |
115–118
|
Raman spectroscopy |
More sensitive to study bonding nature of the functional groups/metal ions |
119–121
|
Mass spectroscopy |
Determination of molecular weight, structural defects/deviations |
122–126
|
X-ray photoelectron spectroscopy (XPS) |
Determination of the surface elemental and chemical composition |
127–129
|
X-ray diffraction |
Determination of 3D structural properties of dendrimeric materials |
109, 130–132 |
Electron paramagnetic resonance (EPR) spectroscopy |
To evaluate the dynamic motion of the end groups/to determine the surface structure of dendrimers |
133–135
|
b. Scattering techniques |
Technique |
Benefits |
Ref. |
Laser light scattering (LLS) |
Determines the size and shape of the dendrimer |
136–137
|
Small-angle neutron scattering (SANS) |
Determines the radius of gyration, molecular mass and position of end groups |
136, 138–140 |
Small-angle X-ray scattering (SAXS) |
To determine the dimensions, radius of gyration, and segment density distribution inside the dendrimer |
136, 140–141 |
c. Microscopic techniques |
Technique |
Benefits |
Ref. |
Scanning electron microscopy (SEM) |
Determination of surface morphology |
142–144
|
Transmission electron microscopy (TEM) |
Determination of size and shape |
142–144
|
Atomic force microscopy (AFM) |
Provides 3D surface profile and projection |
145–147
|
d. Electro-analytical techniques |
Technique |
Benefits |
Ref. |
Electrophoresis (PAGE) |
Evaluation of purity and homogeneity of several types of water-soluble dendrimers |
148–150
|
Electrochemistry |
Determination of the presence of electro-active groups in the dendrimers |
151–152
|
e. Chromatographic techniques |
Technique |
Benefits |
Ref. |
HPLC/UPLC |
Characterization of polydispersity and monitoring the typical surface modification and transformation |
107, 153 |
Size exclusion chromatography (SEC)/gel permeation chromatography |
Determination of molar mass, dimension, and structural defects of dendrimers |
107, 154–155 |
Poly(amido amine) dendrimers
The PAMAM dendrimer has now emerged as one of the most versatile molecules in polymer chemistry and it has been commercialized as Starburst as a brand in the global market. The availability of PAMAM in the global market has provided a boon for researchers as it is the finest architecture in multidimensional research. The first dendritic polymer that was synthesized possessed implausible properties, such as globular shape, tree-like branching, nanoarchitecture, monodisperse, multifunctional with initiator core of ethylenediamine, and a vast number of voids. This dendritic architecture was named Starburst dendrimer. PAMAM dendrimer has three components, an initiator core, an interior shell that has repeating branching units, and the outer surface that has peripheral groups (Fig. 16 and 17). The dendrimers have size ranging from 1 nm to 100 nm in diameter for generation zero (G0) to generation ten (G10). This megamer is a dense macromolecule prepared from the reiterative abiotic pathway, which includes exhaustive Michael addition followed by amidation. In the reaction, an ammonia or ethylenediamine core can be used and acrylic ester namely methyl acrylate has been found to be the best monomer to carry out the synthesis.5,6,18
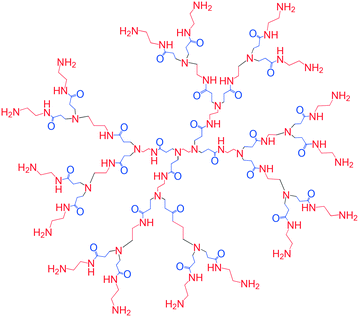 |
| Fig. 16 The structure of a PAMAM dendrimer. | |
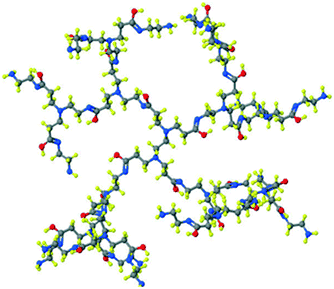 |
| Fig. 17 The 3D structure of a PAMAM dendrimer (G1.0). | |
The microwave-assisted synthesis of PAMAM dendrimer was carried out on a silica surface and the product was characterized. In this method, the results showed that the reaction time required for the synthesis of poly(amidoamine) dendrimer was significantly reduced.156 The half-generation ester-terminated PAMAM dendrimer with an ethylene diamine core was surface modified to obtain water-soluble PAMAM–Tris dendrimer. The synthesis of this dendrimer was carried out in both conventional and microwave-assisted methods. The microwave-assisted synthesis of PAMAM–Tris dendrimer was found to be 3–4 times faster as compared to conventional synthesis. The yield of the products was 90–96%.157 PAMAM dendrimers with a porphyrin core were synthesized using microwave assistance and were characterized by spectroscopic methods.158 Molecular dynamics (MD) simulation investigation revealed the different structural aspects of the PAMAM dendrimer. The MD simulation studies confirm the results produced by other scattering techniques. At higher generations (g > 5) of the PAMAM dendrimer, the density distribution structure changes to a spherical homogeneous compact structure.159 PAMAM dendrimers with an EDA core from generations 1 to 11 have been investigated in the gas phase for atomistic study by MD simulation while considering the other aspects of structural parameters, such as radius of gyration, shape tensor, asphericity, monomer density distribution, spatial arrangement of branch points, terminal group distribution, energy per monomer, molecular surface area, solvent accessible surface area, molecular volume as a function of generation, and the fractal dimensions. It has been possible to study and compare the structures of PAMAM dendrimers up to generation 11.160 Moreover, conformational changes in the PAMAM dendrimer were observed due to effects of pH and solvent. The dendrimer swells in the solvent by a significant percentage while decreasing the pH of the solvent from higher to lower increases the radius of gyration.161,162 The atomistic force-based MD study of PAMAM dendrimer from generation 1 to 7 in explicit water solution provided remarkable results. It was found that PAMAM dendrimer has a very compact globular structures with constant density.163,164Table 2 shows some atomistic parameters of PAMAM dendrimers from generation G1 to G10.13,160,161
Table 2 An atomistic model of PAMAM dendrimers (–NH2 terminated) of different generations showing the numbers of primary and tertiary nitrogens13,160,161
Generation |
Molecular weight (g) |
Number of atoms |
Number of primary nitrogens |
Number of tertiary nitrogens |
Diameter (nm) |
0 |
517 |
84 |
4 |
2 |
1.5 |
1 |
1430 |
228 |
8 |
6 |
2.2 |
2 |
3256 |
516 |
16 |
14 |
2.9 |
3 |
6909 |
1092 |
32 |
30 |
3.6 |
4 |
14 215 |
2244 |
64 |
62 |
4.5 |
5 |
28 826 |
4548 |
128 |
126 |
5.4 |
6 |
58 048 |
9156 |
256 |
254 |
6.7 |
7 |
116 493 |
18 372 |
512 |
510 |
8.1 |
8 |
233 383 |
36 804 |
1024 |
1022 |
9.7 |
9 |
467 162 |
73 668 |
2048 |
2046 |
11.4 |
10 |
934 720 |
147 396 |
4096 |
4094 |
13.5 |
Major synthetic approaches
As already discussed in the traditional synthetic approaches to dendrimers, the PAMAM dendrimer also involves the repetition and activation of the steps after each addition of the monomer unit. Thus, there are two conventional synthetic approaches to prepare the PAMAM dendrimer, i.e., the divergent approach and the convergent approach. In this article, some of the significant synthesis approaches are discussed.
Divergent approach
PAMAM dendrimer synthesis involves raw molecules such as methyl acrylate and core ammonia or ethylene diamine molecule. The synthesis starts from inside core to periphery by iterative steps of the coupling and activation of the monomer. Poly(amidoamine) (PAMAM) dendrimers with an ethylenediamine (EDA) core were synthesized first by Tomalia and his coworkers165 using divergent methodology through two iterative steps, i.e., Michael addition of amines to methyl acrylate followed by amidation of the resulting methyl ester with a large excess. The divergent method exploits the excess reagents.19 The growth of the PAMAM dendrimer proceeds with the repetitive coupling of methyl acrylate followed by activation steps. The amino (–NH2) functionalities present at the periphery are coupled with the methyl acrylate and activated to obtain the next full generation. The reaction of the methyl acrylate with the core molecule (ammonia or EDA) to obtain the half-generation ester-terminated groups is called Michael addition while the reaction of the half-generation PAMAM dendrimer with ethylenediamine (EDA) to yield the full generation PAMAM dendrimer with amine-terminated groups is known as an amidation reaction. The divergent synthesis of the PAMAM dendrimer uses a large excess of the EDA to overcome the side reactions and reduce the defected dendritic molecules (Fig. 18).5,18,166 The synthesis of PAMAM dendrimer divergently suffers from the generation of structurally defective fragments of PAMAM dendrimers. The formation of these byproducts might be due to incomplete Michael reaction, retro-Michael reaction, intramolecular cyclization, and dimerization during the amidation.122
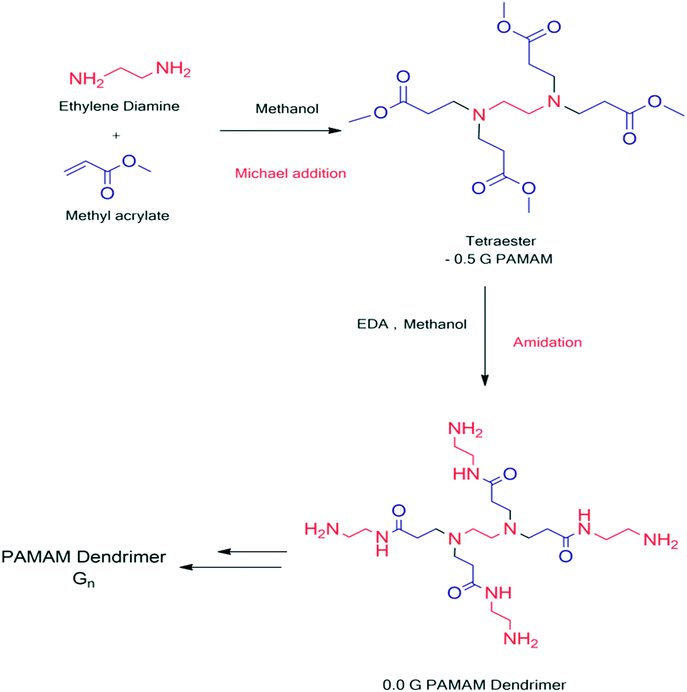 |
| Fig. 18 A schematic representation of the divergent synthesis of the PAMAM dendrimer of ‘n’ generation (Gn). | |
Convergent and click synthesis approach
The convergent synthesis of PAMAM is considered to be an efficient approach to synthesize the PAMAM dendrimer. The method was first introduced by Fréchet and Hawker in the year 1990.29 In 2001, Grayson and Frechet30 systematically discussed the synthesis of the dendrimers using a convergent approach. It showed that convergent synthesis led to the production of the dendrimers with a high level of impurities, unlike the divergent approach. The synthesis starts from outward to inward. A controlled structure of the PAMAM dendrimer could be obtained by this approach. The convergent approach was implemented for the synthesis of PAMAM dendrimers by Christensen et al. from dendrons of PAMAM. This convergent method, moreover, has been exploited by many researchers to produce PAMAM dendrimers by using dendrimers up to generations 1–3 that were prepared divergently via the use of repetitive amide linkages and deprotecting amines. Following the formation of the dendrons, they were coupled to the core having carboxylic acids groups to obtain the complete PAMAM dendrimer.32 Lee and coworkers successfully synthesized PAMAM dendrimers using the convergent method. The scheme involves the synthesis of PAMAM dendrons divergently with propargylamine as the focal point. The iterative two-step reaction involves Michael addition and amidation reactions. In the synthesis of PAMAM dendrons with a propargyl amine focal point, propargyl amine reacted with methyl acrylate in methanol solvent to obtain ester-terminated dendrons. The ester-terminated dendrons undergo amidation in the presence of a large excess of ethylenediamine. These two steps are repeated to get the desired controlled structure of amine-terminated dendrons. The dendrons are attached to the core molecule by using click chemistry. The reaction between alkyne-functionalized PAMAM dendrons with azides was found to involve interesting chemistry. It involves coupling the propargyl-functionalized PAMAM dendrons to an azide moiety. The alkynes and azides undergo Husgen [2 + 3] dipolar cycloaddition. The reaction was catalyzed by copper(I) species, allowing a click reaction to obtain 1,4-regiospecific 1,2,3-triazole core convergent PAMAM dendrimers.40,41
Chromophoric cores have been used to explore the chromogenic properties of the PAMAM dendrimer.167 Thus, the fusion of convergent synthesis and click chemistry of PAMAM dendrimer is considered to be a milestone in synthetic chemistry. It has many advantages and involves interesting chemistry (Fig. 19).40
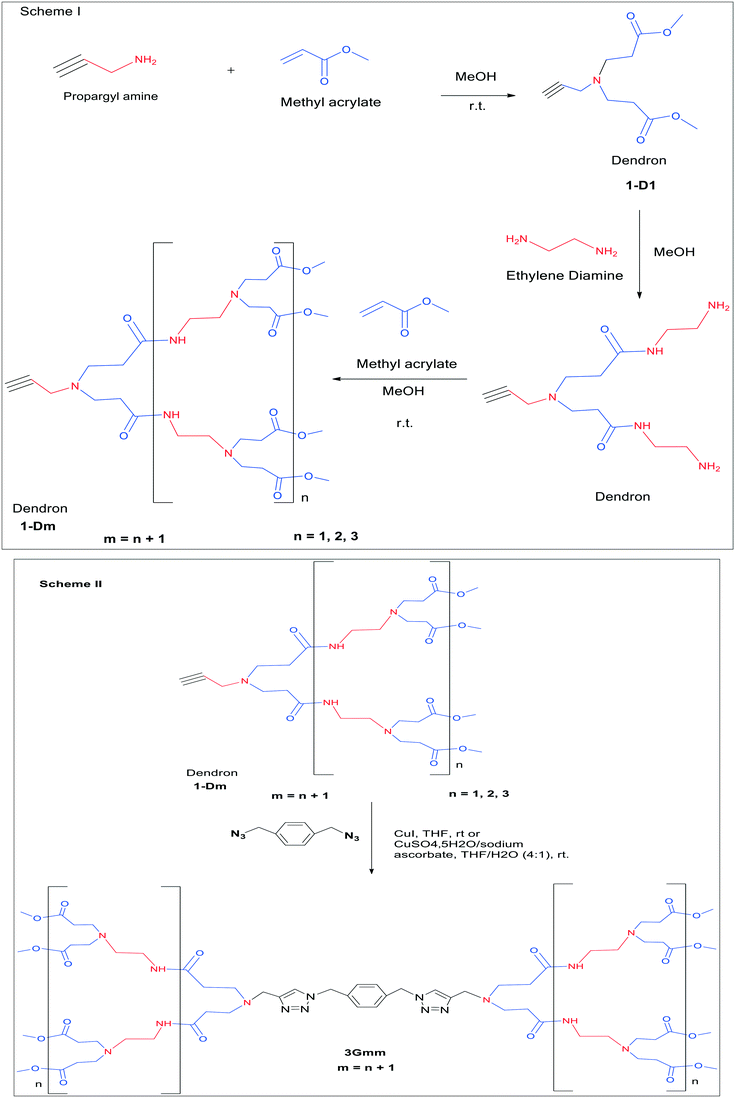 |
| Fig. 19 Scheme I the divergent synthesis of the dendron 1-Dm from methyl acrylate and EDA. Scheme II the formation of the dendrimer 3Gmm using a combination of a convergent method and click chemistry. Reproduced with permission from ref. 40. Copyright 2006 American Chemical Society. | |
Solid-phase synthesis
The solid-phase synthesis of PAMAM dendrimers is a versatile method that involves starting the synthesis on a solid support.
Typically, polymeric resin beads are used as the solid support for dendrimer synthesis. In 1998, Bradley and his coworkers attempted to synthesize poly(amidoamine) dendrimer on a solid support. The resulting PAMAM dendrimers had good homogeneity and were synthesized proficiently and conveniently. The solid-phase synthesis of PAMAM was started with the deprotection of the core polyamine linker conjugates with hydrazine. Methyl acrylate in methanol was reacted with the solid support resin for 12 h to obtain the half-generation dendrimer after removal of excess reagent. The half-generation dendrimer was then reacted with 1,3-diamino propane. The reaction mixture was kept for 24 h to obtain the amine-terminated full generation. The synthesis was based on Tomalia's iterative two-step synthesis of PAMAM dendrimesr.56,58 Huang et al. applied the new strategy to develop a PAMAM dendrimer characterized by inverse amide linkages. Hence, it was called the inverse PAMAM dendrimer synthesis. In this method, oxime resin was used as the solid support with AB2 block monomers for the solid-phase synthesis of a dendrimer. They introduced the AB2 type building block (3-(bis(3-((tert-butoxycarbonyl)amino)propyl)amino)propanoic acid) molecule, which has a free carboxylic acid group and a protected amino group. The solid-phase synthesis of the PAMAM dendron was started with the coupling of resin with a protruded oxime group and an AB2 type building block (Fig. 20).168,169
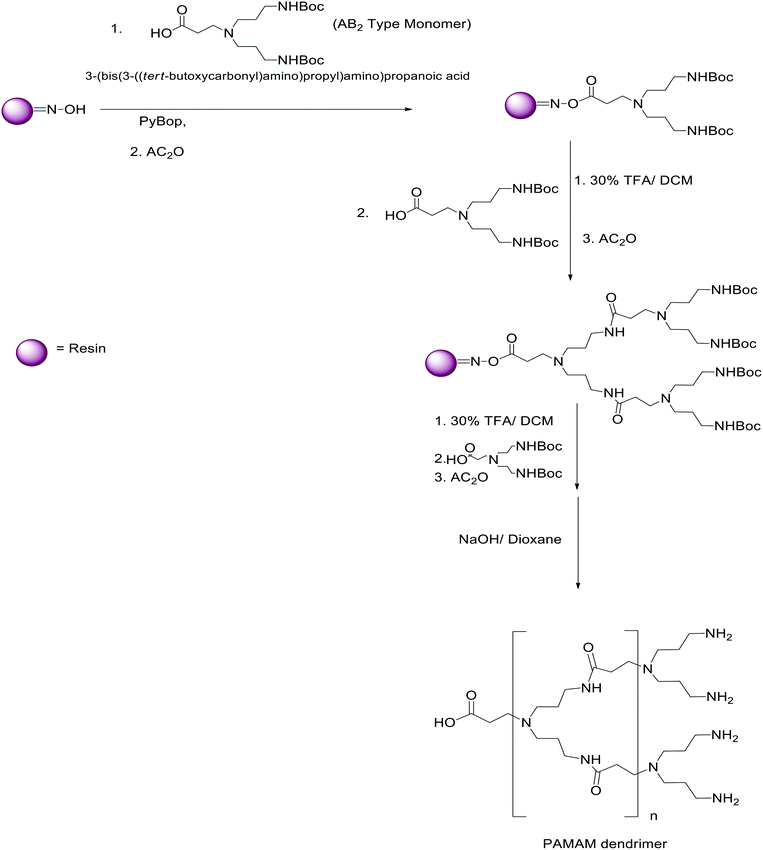 |
| Fig. 20 The solid-phase synthesis of the PAMAM dendrimer. Reproduced from ref. 168 with permission from the Royal Society of Chemistry, copyright 2013. | |
Applications of PAMAM dendrimer hybrids
Dendrimers have been significantly used in various fields. Dendrimer researchers have been synthesizing PAMAM dendrimers and hybrids as per their needs in different fields. The dendrimers have been designed not only for biological applications170 but also for environmental171 and industrial applications.172 This has enabled the dendrimer to reveal its superior ability as a multifunctional capping agent for metal nanoparticles in bioimaging, drug delivery, and sensor applications.173
Nanoscale architectures have been designed by using PAMAM dendrimers, which are notably used in the biomedical fields for molecular imaging purposes, non-viral gene delivery, etc.174 The surface amino (–NH2) groups of the PAMAM dendrimer of generations 0, 2, and 3 were functionalized by complex [Ru(EDTA)(H2O)], then H2O ligands were replaced by the NO group by nitrosylation. The reactivity of the species before and after was studied.175 A new kind of iron oxide nanoparticle has been synthesized by using PAMAM dendrimer as a coating material. The PAMAM dendrimer was functionalized with lipid molecules (C12). The synthesis of iron oxide nanoparticles with a broad diameter range based on the PAMAM dendrimer was used to study the modulated structural and magnetic properties along with some biomedical applications. These new dendrimer-stabilized NPs were used to study the optimization of the relaxometry performance of the molecule.176
Thus, dendrimer chemistry never stopped here. Many publications have been reported to consolidate noteworthy applications. Relative advances have been made and new methodologies have been developed. Nevertheless, the negatively charged 5,6-carboxyfluorescein (CF) probe was attached to the positively charged PAMAM dendrimer of generation G3 to G7. The thermodynamic binding aspect was ascertained using absorbance, fluorescence measurement, and isothermal calorimetric titration.177 Diverse nanoparticles could be synthesized from metals, metal oxide, and metal halides. The important role played by the poly(amidoamine) dendrimer was to stabilize the synthesized nanoparticles.178 Thus, PAMAM dendrimers and their hybrids have many multipurpose applications in nearly all specified areas. Hence, it as one of the great tasks to researchers around the world to develop a more advanced methodology to explore its relevance in diverse areas. This would confer a better platform to exploit dendrimer chemistry extensively to enhance the applicative measurements in all fields. Thus, very important applications are discussed in this review.
Gene therapy
Gene therapy is a promising technology for treating several life-threatening diseases, like AIDS, cancer, or some inherited diseases. The molecular transformation in genetic materials could lead to disorders.179 The rectification of the genetic disorders could be possible, somehow, if the genetic material could be transferred very efficiently into the cytoplasm and the nucleus of the cell without producing any type of toxicity in the cell. Functionalized PAMAM dendrimers have been found to be promising as non-viral gene vectors to transfect genes at the molecular level. It has provided one of the most efficient platforms for gene delivery.180,181
Non-viral vectors are a safe way to transfect genes in cells. This was being achieved by employing liposomes and some cationic polymers. They are good for in vitro delivery but produce some toxicity. The unique properties, such as non-immunogenicity, low cytotoxicity, and cationic nature, of PAMAM and its hybrids make them outstanding non-viral gene vectors. Polyanionic DNA interacts electrostatically with the cationic functionalized PAMAM dendrimer.180,182 Figueroa et al. synthesized a gold nanoparticle–poly(amidoamine) dendrimer G 4.0 conjugate (AuPAMAM conjugate) as an efficient non-viral vector for transfecting plasmid DNA. The AuPAMAM conjugate was prepared from PAMAM G 4.0 dendrimer crosslinked to the carboxylic acid (–COOH) terminals of the AuNPS by using 1-ethyl-3-[3-dimethylaminopropyl]carbodiimide hydrochloride (EDC) and N-hydroxy sulfosuccinimide (Sulfo-NHS) reagents (Fig. 21).183 Thus, PAMAM dendrimers are the most promising nanocarriers for efficient drug delivery and gene delivery.184 The KRTR peptides were derived from the influenza B virus and conjugated with PAMAM dendrimer of generation 3 (G 3.0). This combination reduced the cytotoxicity of the PAMAM dendrimer and showed efficient transfection ability.185 PAMAM dendrimers of generations 1 to 5 were prepared divergently with polyethylene oxide (Jeffamine T) as a core molecule. The synthesized Jeffamine-cored PAMAM dendrimers (JCPDs) were complexed with the plasmid DNA of the HeLa cell line. JCPD was evaluated as an efficient reagent for transfection. The results were encouraging and showed the effectiveness of JCPD for gene delivery and it can be used as a non-viral gene vector186 A graphene–oleate–poly(amidoamine) dendrimer hybrid was synthesized. The graphene was functionalized with oleic acid and PAMAM dendrimer was attached to the surface of the oleic acid-functionalized graphene with covalent linkages. The synthesized graphene–oleate–PAMAM hybrids showed good biocompatibility and great gene transfection capacity.187
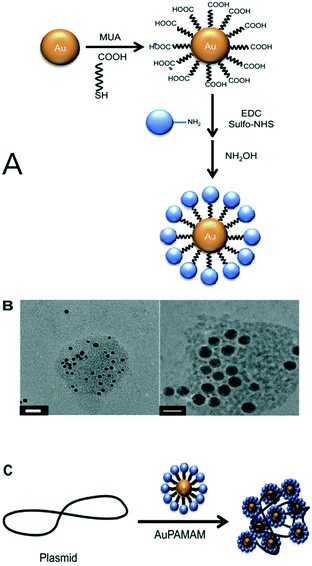 |
| Fig. 21 (A) A schematic representation of the synthesis of AuPAMAM NPs via the original pH 6 method. (B) TEM micrographs of generation 4 AuPAMAM (AuG4) complexed with GFP plasmid at a 1 : 30 MR. DNA was stained with uranyl acetate. The scale bar represents 20 nm. (C) A schematic illustration showing the complexation of AuPAMAM NPs with plasmid DNA. Reproduced from ref. 183, copyright 2013, with permission from Elsevier. | |
Drug delivery
The aim of a drug delivery vector is the controlled release of drugs at a specific target. Therefore, the carrier of the drug to the target points must possess some ideal characteristics. Any biomedical vector should be non-toxic, non-immunogenic, and have structural control over the drug, good scaffolding, and suitable cellular linkage, endocytosis, and intracellular trafficking.188 In the biomedical field, the treatment of many chronic diseases, such as cancer and acquired diseases, requires advanced technology. Thus, drug release at the target becomes a very important task. Due to the globular shape, the interior cavities, and a vast number of terminal groups present in the PAMAM dendrimer, it can form stable complexes with drugs, antibodies, DNA, etc. The dendrimer can be used as a versatile carrier for the delivery of drugs. It has a superior ability to solubilize hydrophobic drugs. PAMAM dendrimer is non-toxic with low cytotoxicity and is biodegradable, non-immunogenic, and multifunctional. These superior properties enabled various applications in the biomedical field, such as drug delivery, DNA transfection, and tumor diagnosis and treatment.10
PAMAM dendrimer conjugates have been evaluated for the potential ability for the delivery of drugs in tumor-induced mice. The PAMAM dendrimer up to the fourth generation was synthesized. The surface groups were tailored with folic acid and folic acid–PEG–NHS (N-hydroxysuccinimide) conjugates. The study showed that folate–PEG–PAMAM dendrimer conjugates were very efficient and safe for drug delivery in mice (Fig. 22–24).189
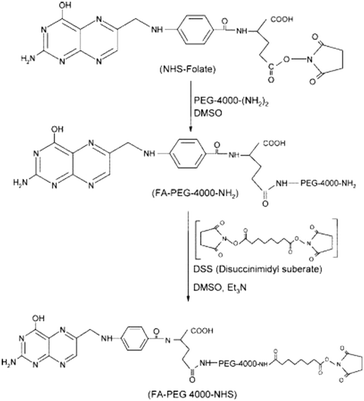 |
| Fig. 22 The synthesis of an NHS–PEG4000–folic acid conjugate from an NHS–folic acid conjugate. Reproduced with permission from ref. 189. Copyright 2008 American Chemical Society. | |
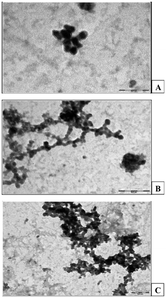 |
| Fig. 23 Electron microscopic photographs of (A) 4.0 G PAMAM dendrimers; (B) FA–PAMAM (folic acid–dendrimer conjugates); and (C) FA–PEG–PAMAM (folic acid–PEG–dendrimer conjugates). Reproduced with permission from ref. 189. Copyright 2008 American Chemical Society. | |
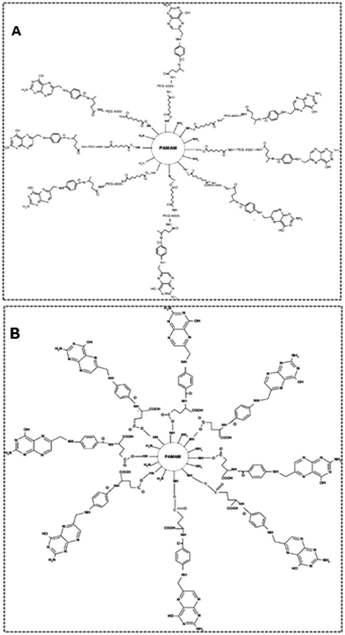 |
| Fig. 24 (A) A chemical model structure of the folic acid–PEG4000–4.0G PAMAM dendrimer. (B) A chemical model structure of the folic acid-conjugated 4.0G PAMAM dendrimer. Reproduced with permission from ref. 189. Copyright 2008 American Chemical Society. | |
Understanding the bonding and nature of the interactions between the drug delivery system and the drug determines the controlled release at the target site. Polyethylene glycol (PEG) was conjugated with fourth-generation (G4) PAMAM dendrimers. The behavior of methotrexate (MTX) drug was studied with PEGylated and non-PEGlyted PAMAM dendrimers. The drug methotrexate (MTX) was coupled to both PEGlyted and non-PEGlyted PAMAM dendrimer via amide linkages to form MTX–G4–PEG and MTX–G4, respectively. The covalent interaction of the drug and the dendrimer was found to be more effective. Thus, the MTX–G4–PEG hybrid has shown a greater ability for targeted drug delivery.190 The delivery of drugs at the target site has been reported in many articles, but the carrier molecules might have been engineered with properties that do not lead to side-effects from the therapy.
Catalysis
The synthesis of novel molecules is supposed to be unconventional on a laboratory or industrial scale. The activation energy of the individual molecule plays a pivotal role in organic synthesis. To carry out a reaction with a high yield, one often needs to use harsh conditions. An option to eliminate the harsh conditions is using a catalyst. Dendrimers are emerging as a promising architecture that has solved many problems relevant to catalysis in reactions. The unique properties of the PAMAM dendrimer were studied well and exploited as a heterogeneous catalyst to construct various molecules in organic or inorganic synthesis. Composites of the PAMAM dendrimers are important for catalysis. The metal complexes of second-generation poly(amidoamine) dendrimer with 16 amido ethanol groups and metal ions (Ag+, Cu2+, Zn2+, Fe2+, and Fe3+) were synthesized. The investigations regarding the coordination chemistry of the metal ions with the PAMAM dendrimer of these inorganic hybrids of PAMAM dendrimer were carried out by two techniques, viz. electron capture dissociation (ECD) and collision-induced dissociation (CID). The results showed that both core tertiary amines of the PAMAM dendrimer are coordinated to Fe3+ and Cu2+ ions whereas the single-core tertiary amine has coordinated to Ag+ ion. However, some of the metal ions, viz. Zn2+ and Fe2+ did not show any coordination with the PAMAM core.191
The higher-generation poly(amidoamine) dendrimer was embedded in the crosslinked poly(2-hydroxyethyl methacrylate) polymeric matrix. The embedded PAMAM dendrimer was used as the template for the formation of organic/inorganic hybrid nanoclusters with metal salts. The inorganic nanoclusters were obtained by reducing gold, silver, and platinum salts on the dispersed PAMAM dendrimer template. The newly formed organic/inorganic nanoclusters were found to be promising in terms of their colloidal properties, showing interesting optical and catalytic properties.192 Meanwhile, PAMAM dendrimers are now becoming popular in catalysis to synthesize various compounds. The aminopropyl silica-supported immobilized phosphino-PAMAM dendrimer (G0–G4) was complexed with Pd(PPh2)Cl2. The synthesized dendrimers were used in the catalysis of the carbonylation reaction of iodoarenes in methanol. The product was obtained at a high yield and the catalyst was recovered. Thus, PAMAM dendrimers have extensive uses in the field of catalysis.193 The Starburst dendrimer is mostly accepted as the template for the synthesis of nanocomposites. The poly(amidoamine) dendrimer was complexed with Cu2+ ions and then reduced with a suitable reducing agent to zero oxidation state to form zero-valent copper nanoclusters of the PAMAM dendrimers. The coordinating of the copper with the PAMAM could be studied.194 People suffering from organophosphate poisoning could be treated with the antidote synthesized from the functionalization of peripheral amino groups of PAMAM dendrimer with the class of oximes. When pyridinium aldoxime (PAM) was treated with fifth-generation poly(amidoamine) dendrimers, then oxime-conjugated PAMAM dendrimer hybrids were obtained. The conjugate was used to treat organophosphate poisoning by showing catalytic scavenging activity toward the organophosphates. Thus, the synthesized oxime–PAMAM G-5 dendrimer could be used as a scavenger of organophosphates.195 A fourth-generation PAMAM dendrimer was grafted onto silica and Mn(II) salen complex was immobilized on the periphery of the dendrimer.
A study has been done to investigate the effects of loading Mn(II) salen complexes on higher and lower generations of dendrimer, and the catalytic activity of Mn(II) salen-immobilized PAMAM-silica dendrimers for the epoxidation of olefins was explored. The higher fourth-generation dendrimer was found to be more catalytically active than the lower generation PAMAM.196
PAMAM dendrimers of generations 3, 4, and 5 were tailored with 4-carbomethoxypyrrolidone to yield PAMAM–Pyr hybrid dendrimers. The modified PAMAM–Pyr dendrimers of generations 3–5 were employed to stabilize silver nanoparticles (AgNPs). The newly synthesized efficient nanocomposites called PAMAM–Pyr dendrimer-stabilized silver nanocomposites (Ag-PPDNCs) were evaluated for catalytic activity in the reduction of 4-nitrophenol (4-NP) into 4-aminophenol (4-AP). The results were striking and the dendrimers were found to be competent for the catalytic reduction of 4-NP to 4-AP (Fig. 25–27).197
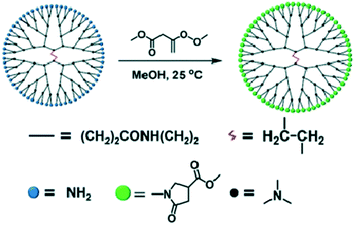 |
| Fig. 25 The modification of a PAMAM dendrimer with 4-carbomethoxycarbonylpyrrolidone. Reproduced from ref. 197 with permission from the Royal Society of Chemistry, copyright 2019. | |
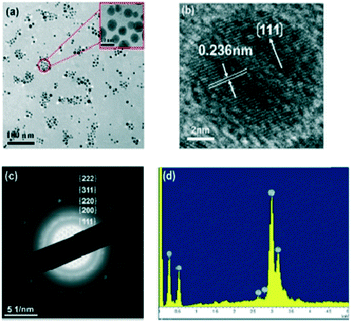 |
| Fig. 26 (a) A TEM image, (b) a HRTEM image, (c) the SAED pattern and (d) an EDS image of Ag–PPDNCs prepared with G4-Pyr. Reproduced from ref. 197 with permission from the Royal Society of Chemistry, copyright 2019. | |
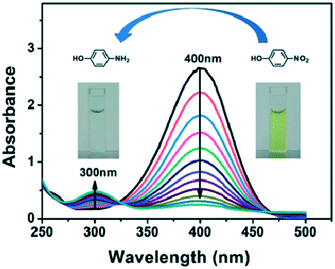 |
| Fig. 27 UV-vis spectra of the catalytic reduction of 4-nitrophenol using Ag–PPDNCs (G4-Pyr) as the catalyst. Reaction conditions: 4-NP = 1.275 × 10−2 M, NaBH4 = 0.2 M, Ag–PPDNCs (G4-Pyr) = 0.44 mM, T = 25 °C. Reproduced from ref. 197 with permission from the Royal Society of Chemistry, copyright 2019. | |
The use of dendrimers in all the relevant areas has been reported so far. The dendrimers have varying sizes, globular shapes, and the capacity to provide a large surface area. Thus, dendrimers are used as organocatalysts that can be recycled and reused.198
Controlled release of agrochemicals
Nowadays, nanotechnology has emerged as a promising tool for the controlled release of agrochemicals. Agrochemical delivery carriers show the properties of biodegradability, low toxicity, low cost, high reproducibility, easy and fast preparation and characterization, water uptake, and reversible properties.199 The nanocarrier-based delivery systems are not confined only to drug delivery but can also be used to increase the productivity of the crops as well. The tools rely on the development of targeted pesticide delivery systems to maximize efficacy and reduce the environmental impact of agrochemicals.200 The distinct properties of polymers could potentially be used in the encapsulation of molecular frames. In recent years, micro- and nano-encapsulation have emerged for better design and release of agrochemicals.201 To enhance the applicability and efficiency of agrochemical delivery in crops, PAMAM dendrimers are promising polymers that could be an alternative for the delivery of agrochemicals. Bunderson et al. used a mixture of PAMAM dendrimers to administer fertilizers through the leaves and roots of the plant.202 Liu et al. reported the controlled delivery of thiamethoxam, a hydrophobic pesticide, by water-soluble fluorescent PDI-cored nanosized cationic dendrimers containing hydrophobic dendritic polyesters and peripheral amines.203
Waste water remediation
The growth in industrial sectors and increased habitation in towns and villages lead to increased water pollution. Effluent from industry is composed of harmful ingredients, such as inorganic metals ions, organic dyes, and many deteriorating by-products, whereas household sewage contains microflora that are responsible for causing disease. The adsorption of poly(amidoamine) dendrimer on the silica oxide surface can be used to study various aspects, such as pH, ionic strength, and dendrimer generations.204 The poly(amidoamine) dendrimer is known as an excellent chelating agent for the removal of Co(II) ions. PAMAM can form stable chelation with Co(II) ions in solution. This could be accomplished by the use of a DOE–RSM-based PAMAM dendrimer-enhanced ultrafiltration (PAMAM-DEUF) process.205
Harmful organic or inorganic dyes can be removed from the water by nanocomposites of PAMAM dendrimers. The PAMAM/CuS/AA nanocomposites are used for the adsorption of the Isma fast acid yellow G dye from the wastewater. The nanocomposites were synthesized by the gamma irradiation technique. Based on the Langmuir adsorption isotherm, Isma fast acid yellow G dye has shown significant adsorption by PAMAM/CuS/AA nanocomposites.206
Wastewater containing different hazardous chemicals can be cleaned using nanocomposites of PAMAM dendrimers. Aromatic hydrocarbons, such as naphthalene, anthracene or phenanthrene, could be removed efficiently. It was accomplished by synthesizing the higher-generation PAMAM dendrimer (up to G10). The PAMAM G10 dendrimer was synthesized on magnetic nanoparticles and then end groups were modified with benzaldehyde molecules. These MNP-PAMAM dendrimers with benzaldehyde as the peripheral groups were used to remove naphthalene from wastewater. A high adsorption capacity of about 93–100% was observed for the removal of naphthalene from the wastewater.207 PAMAM dendrimers of generations 0, 1, and 2 were grafted on a thin-film composite-forward osmosis (TFC-FO) membrane to treat wastewater to remove ammonia selectively and study the antifouling capacity of the TFC-FO membrane. The PAMAM dendrimer-grafted TFC-FO membrane showed superior ability to remove ammonia from the wastewater and enhance the antifouling capacity of the membrane. The characterization and mechanism of osmosis are demonstrated in Fig. 28 and 29.208
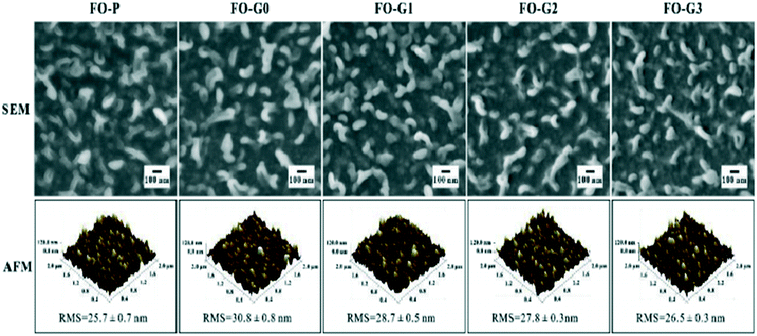 |
| Fig. 28 SEM and AFM images of the top surfaces of pristine and PAMAM dendrimer-grafted TFC-FO membranes. Reproduced from ref. 208, copyright 2019, with permission from Elsevier. | |
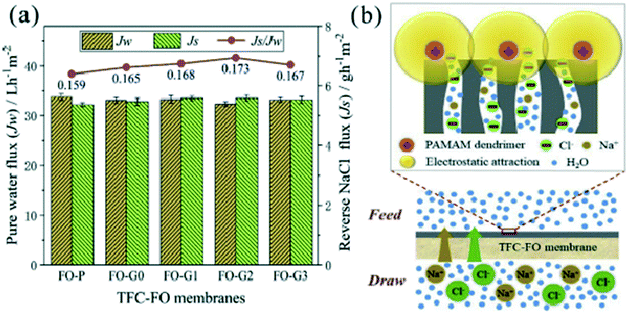 |
| Fig. 29 (a) Osmotic performances of pristine and PAMAM dendrimer-grafted TFC-FO membranes. (b) A schematic diagram of NaCl transport through the PA layer of the PAMAM dendrimer-grafted TFC-FO membrane with ultrapure water as the feed solution. Reprinted from ref. 208, copyright 2019, with permission from Elsevier. | |
The experimental results showed that Cu(II) forms the most stable coordination sphere with the tertiary nitrogen of the ethylenediamine core and the oxygen of the carbonyl side of the amide group. At neutral pH, the binding energy for the complexation of PAMAM G0 with Cu(II), Ni(II), and Zn(II) ions has the order Cu(II) > Ni(II) > Zn(II).209 Zhou et al. studied the adsorption phenomenon for the uptake of Hg(II) ions from wastewater. They synthesized Schiff's bases of PAMAM dendrimers supported over ferri–ferro magnetic nanoparticles. The surface amino (–NH2) groups of the PAMAM dendrimers were capped with salicylaldehyde. The salicylaldehyde Schiff's bases of PAMAM dendrimers (Fe3O4@SIO2-PAMAM-S) of generations 0, 1, and 2 were evaluated for the adsorption isotherm for adsorption of Hg(II) ions from aqueous solution. The results showed that Fe3O4@SIO2-G2.0-S has the best capacity to adsorb Hg(II) ions from aqueous solution while Fe3O4@SIO2-G0.0-S has the least capacity. The comparative study of the adsorption capacity of the Schiff's bases is shown in Table 3 (Fig. 30 and 31).210
Table 3 Adsorption isotherm parameters for Hg(II)210
Adsorbent |
T (°C) |
Langmuir model |
Freundlich model |
q
m
|
K
L
|
R
L
2
|
K
F
|
n
|
R
F
2
|
Fe3O4@SiO2-G0-S |
15 |
0.74 |
196.86 |
0.9834 |
4.28 |
2.0765 |
0.8698 |
25 |
0.91 |
265.86 |
0.9690 |
6.27 |
2.0574 |
0.8828 |
35 |
1.21 |
375.57 |
0.9798 |
13.13 |
1.8641 |
0.9342 |
Fe3O4@SiO2-G1.0-S |
15 |
1.13 |
366.09 |
0.9795 |
4.53 |
3.1267 |
0.9523 |
25 |
1.36 |
506.97 |
0.9753 |
5.65 |
3.0115 |
0.9697 |
35 |
1.72 |
652.42 |
0.9942 |
9.02 |
2.5434 |
0.9663 |
Fe3O4@SiO2-G2.0-S |
15 |
2.94 |
449.33 |
0.9926 |
38.18 |
1.8127 |
0.9524 |
25 |
3.02 |
578.77 |
0.9922 |
36.24 |
1.9207 |
0.9303 |
35 |
3.11 |
733.38 |
0.9966 |
30.57 |
2.1134 |
0.9622 |
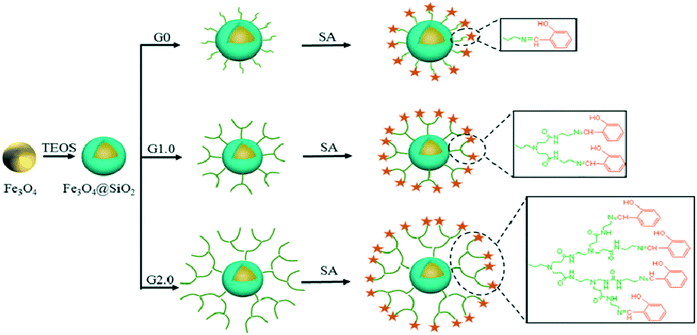 |
| Fig. 30 A schematic representation of the synthesis of Fe3O4@SIO2-G0.0-S ∼ Fe3O4@SIO2-G2.0-S. Reproduced from ref. 210, copyright 2020, with permission from Elsevier. | |
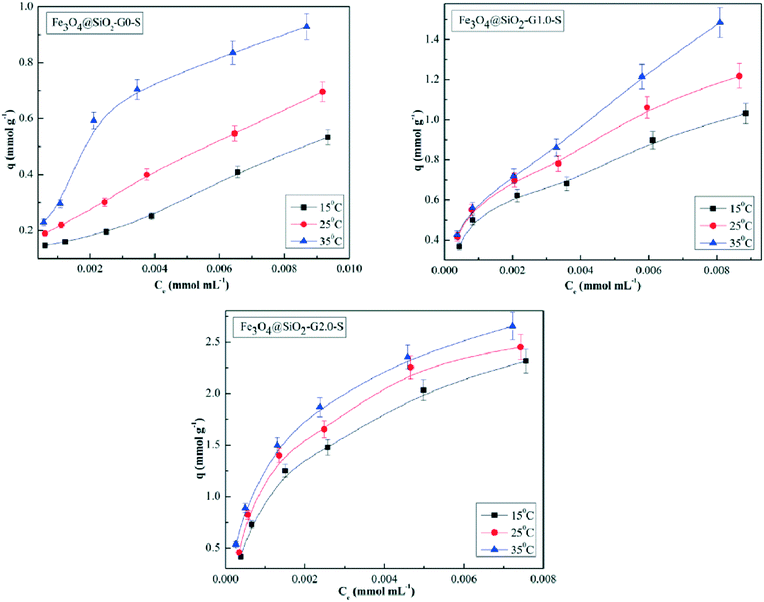 |
| Fig. 31 A graphical representation of the adsorption isotherm plots for Hg(II). Reproduced from ref. 210, copyright 2020, with permission from Elsevier. | |
Biosensing
The PAMAM dendrimer provides a major platform in the construction of various types of biosensors. The PAMAM dendrimer is known for its unusual features, like three-dimensional globular shape, controlled topology, excellent surface functionality, hydrophilicity, and monodispersity.6 The use of sensing technology in the biomedical field is becoming important, and future challenges relating to sensor platforms should be countered. The sensing capabilities of compounds should be monitored with the aim of obtaining incredible sensitivity, enhanced target specificity, reduced non-linearity, physicochemical stability, and reproducibility.211
The unique properties of PAMAM dendrimers make them a wise choice for use in biosensors.212 The PAMAM dendrimer is used in the immobilization of biorecognition receptor elements (such as DNA, antibodies, enzymes) on the transducer part of the electrode.213 The role of the PAMAM dendrimer is shown in Fig. 32.
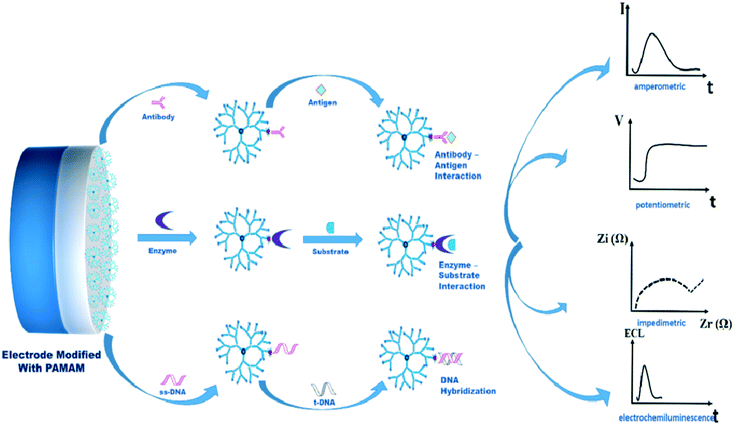 |
| Fig. 32 The role of the PAMAM dendrimer in the bio-sensing mechanism. Reproduced from ref. 213, copyright 2015, with permission from Elsevier. | |
A biosensor is an analytical device that records a response after the biochemical reaction between the analyte biological substance and the bio-receptor substance used in the biosensor (Fig. 33).214
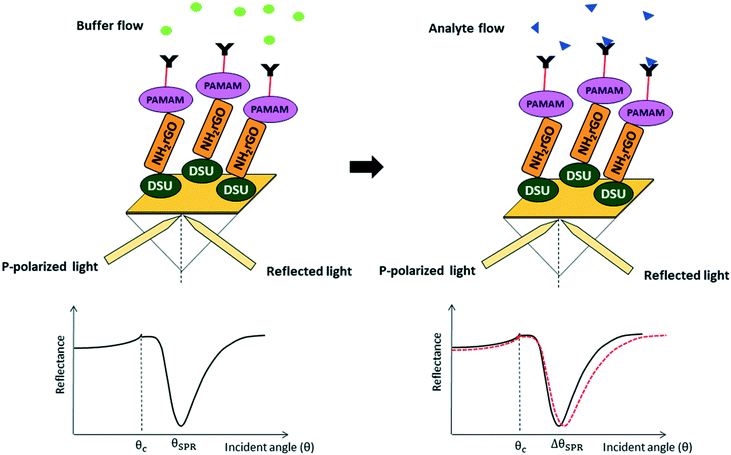 |
| Fig. 33 A schematic demonstration of SPR signals obtained before and after the analyte flow. Reproduced from ref. 223 with permission from Springer Nature, copyright 2020. | |
The construction of advanced biosensors with improved analytical properties is becoming a major task. Researchers and scientists have been targeting the design and fabrication of biosensors to achieve high selectivity and greater sensitivity. The construction of the sensor is accompanied by electrode modification and efficient immobilization of an enzyme on the surface of the electrode. Thus, immobilization is an essential stage in the fabrication that mainly involves encapsulation, adsorption, entrapment, cross-linking and covalent binding.215 The sensitivity of the biosensors depends upon the rate of electronic migration between the electrode (collector) and the electrolyte. It can be achieved by using modified conducting polymers and designed nanocomposites.
The metallic conductivity of conducting polymers and nanocomposites accompanied with nano-fillers dispersed over the matrix shows their interfacial characteristics to encourage electronic transportation and hence enhance the electrochemical sensitivity of biosensors.216 The modified PAMAM dendrimer confers a large polymeric surface area to immobilize a vast number of biorecognition elements. Senel et al. synthesized an amperometric glucose biosensor based on modified PAMAM dendrimers up to the third generation. Dendronized polypyrrole copolymer was used as a conducting film onto which GOx enzyme was immobilized by covalent linkages. It provided high enzymatic loading and lifetime stability to the electrode.217
Liu et al. constructed a PAMAM dendrimer-based H2O2 biosensor, as an amperometric biosensor for the detection of hydrogen peroxide concentration. The gold electrode was surface engineered with cystamine molecules. The PAMAM dendrimer (G4) was immobilized on the cystamine-modified gold electrode by cross-linking with glutaraldehyde. The amine-terminated PAMAM/cystamine-modified Au electrode was dipped in gold colloids. This led to the implantation of the nano-Au monolayer onto the PAMAM dendrimer. In the final step, horseradish peroxidase (HRP) was immobilized on the nano-Au monolayer supported by the amine groups of the PAMAM dendrimer (G4). The newly constructed H2O2 biosensor was evaluated for the determination of the hydrogen peroxide concentration in real samples. The results were satisfactory and showed the sensitivity, stability and reproducibility of the H2O2 biosensor.218 Zhu et al. have developed a gold (Au) electrode anchored with mercaptoacetic acid (RSH) ending with carboxylic acid groups on the surface. The Au-RSH electrode was then modified with poly(amidoamine) dendrimer (G4). The amine-terminated PAMAM dendrimer was attached to the Au-RSH by forming amide linkages (–CONH–) with the terminal –COOH groups of the mercaptoacetic acid. The peptide chemistry was used to anchor the PAMAM dendrimer (G4) onto the thiol. The biorecognition receptor element (DNA probe) was immobilized on the PAMAM-modified Au electrode. The immobilization of DNA probes has become possible using the surface amino groups of PAMAM dendrimers and the phosphate groups of the oligonucleotides at the 5′ end through forming phosphoramidite bonds. The PAMAM-modified Au electrode biosensor was evaluated for the measurement of ssDNA hybridization with daunomycin (DNR) as an indicator. The PAMAM-modified Au electrode has shown enhanced sensitivity, selectivity, and chemical stability for the measurement of DNA hybridization.219 Amperometric biosensors have been constructed by using hybrids of the PAMAM dendrimer. The pyrrole-cored PAMAM dendrimer217 and ferrocene-cored PAMAM dendrimer220 were synthesized divergently. Glucose oxidase (GOx) was covalently immobilized onto the pyrrole-PAMAM dendrimer and ferrocene-PAMAM dendrimer to construct an amperometric glucose biosensor.
The cystamine-PAMAM dendrimer was coated over the gold electrode for the immobilization of the amino-modified ssDNA aptamers. This biosensor based on the aptamer was used for the detection of the food contaminant mycotoxin aflatoxin B1 (AFB1).221 Elancheziyan and Senthilkumar developed an electrochemical biosensor for the detection of hydrogen peroxide.222 They synthesized the gold nanoparticles embedded in the cavities of the poly(amidoamine) dendrimer.
The non-ionic immobilization of hemoglobin (Hb) onto the periphery of the PAMAM dendrimer has been carried out. The Hb/PAMAM-AuNPs biosensor gave outstanding results with an excellent detection range from 20 to 950 μM.222 Biosensing is becoming a booming technology in the biomedical field. The development and design of electrodes coated with various biomarkers using PAMAM dendrimers as a template for immobilization have been considered.
Many countries are facing the problem of dengue disease. The delayed diagnosis of the dengue virus may lead to deaths but early diagnosis may help to save the lives of many people. Recently, Omar et al. devised an SPR sensor for the detection of the dengue virus type 2 E protein. a graphene oxide-PAMAM dendrimer nanocomposite was immobilized on the gold surface of the sensor for the detection of DENV-2 E protein. This SPR sensor showed excellent sensitivity for the DENV-2 E protein at a very low concentration, i.e., 0.08 pM of the analyte (Fig. 34).223 The emerging capability of the biosensing technology for the detection of analytes at the very lowest concentration is promising for the biomedical field. This would allow the early diagnosis of many diseases and play a vital role in saving the lives of many individuals.
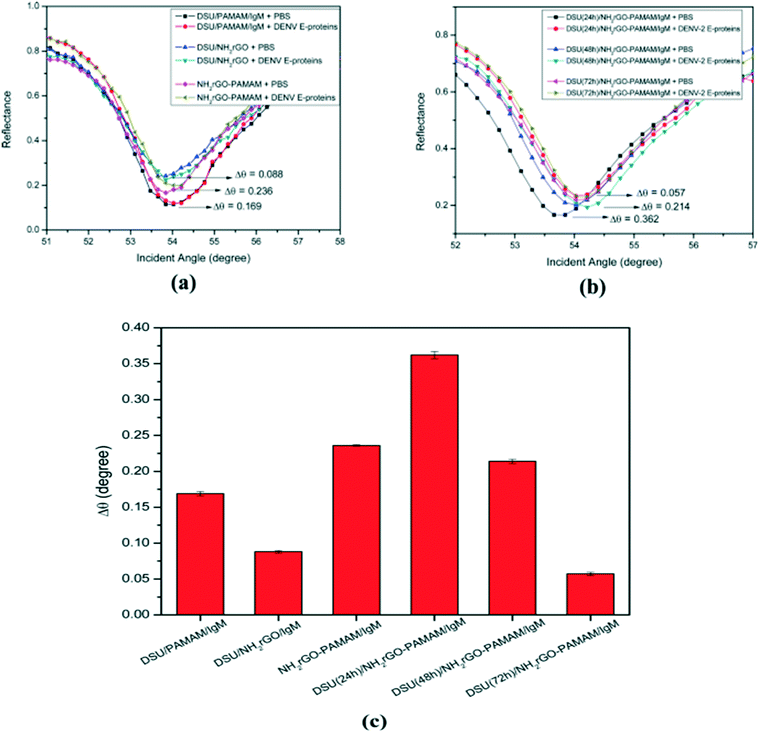 |
| Fig. 34 The detection of 100 pM DENV-2 E-protein (a) on different types of composite sensor layers and (b) with different incubation times for self-assembly. (c) The results presented in a bar graph. Reproduced from ref. 223 with permission from Springer Nature, copyright 2020. | |
Imaging
Magnetic resonance imaging is a promising technique that can be used to obtain a three-dimensional anatomical scan of the human body. This technique requires paramagnetic or superparamagnetic materials that show efficient magnetic properties. Gd(III) ion is paramagnetic and is considered one of the better choices as a contrast agent for imaging. The properties such as multifunctionality, enormous cavities, biocompatibility, and the huge number of peripheral groups make the PAMAM dendrimer act as a template in imaging. It encapsulates the Gd(III) ions and extends the lifetime in the blood to increase the effectiveness.12 A study has shown that as the generations of the PAMAM dendrimer increase, the relaxivity of the contrast agent also increases.224
The direct use of the PAMAM dendrimer may cause some cytotoxicity in the body. The PAMAM dendrimer as a template for the contrast agent could be manipulated to low cytotoxicity by modifying the surface functionalities. The Gd(III) nanoparticles encapsulated by PAMAM dendrimer were surface-functionalized with polyethylene glycol (PEG) chains to reduce the cytotoxicity and enhance the paramagnetic properties.225,226 Thus, the promising development of bioimaging has opened doors to enhanced sensitivity in tomography and more reliable results.
Almasi et al. synthesizes a PET/MR dual-modal imaging agent by using the functionalized PAMAM dendrimer. The acetylated PAMAM dendrimer was coated on iron oxide NPs. The iron oxide NPs coated with acetylated PAMAM dendrimer G 4.0 were surface engineered with gallium-68 radio-labelled S-2-(4-isothiocyanatobenzyl)-1,4,7,10-tetraazacyclododecane tetraacetic acid (p-SCN-Bn-DOTA). This imaging agent was applied for tumor diagnosis.227 Thus, the PAMAM dendrimer has boundless significance in bioimaging in the biomedical field. It would be encouraging to expand this technique to overcome existing challenges and to develop cost-effective, sophisticated, and precise tools.
Conclusions
This review has summarized the varied synthetic strategies involved in fabricating dendrimeric composites. Today the rising interest in the dendrimeric field has led to various formulations and functionalizations involving the basic skeleton of dendrimers. The uniquely designed 3D morphologies and properties of PAMAM dendrimeric frameworks attained through new breakthroughs are utilized in tailoring promising surface functionalities in the fundamental assemblies of dendrites. Efforts have been made to generate composites based on the poly(amidoamine) dendrimeric skeleton. The illustration of dendrimeric composites with different frameworks is feasible by means of modern spectroscopic and advanced microscopic techniques. Assorted characterization techniques enable scientists to advance novel synthetic methodologies other than the usual divergent and convergent approaches for designing diverse dendritic composites. Classically designed and strategically synthesized organic and inorganic doped composites find significant usage in several fields, including the biomedical sciences, water remediation, catalysis, and electronics. PAMAM dendrimeric composites are extensively utilized in the biomedical sector as gene/drug carriers, for cell/tissue delivery, in bioimaging for diagnostic analysis, and in the treatment of numerous diseases. To date, dendrimers have been explored in many fields, such as in the biomedical, pharmaceutical, environmental, and electronic industries, with a bright future in nanomedicine, drug delivery, gene transfer, drug design, catalysis, and biosensing.
This overview aims to offer a comprehensive summary and it covers a lot of ground-breaking research relating to the synthesis and functional applicability of emerging dendritic hybrids/composites. It may be too specialized to be of significant general interest to the numerous global researchers and scientists working in the field of S&T, but the wide perspective view and scope may support the advance of certain innovative strategies for the synthesis and widespread application of dendrimeric composites. Nevertheless, the poor synthesis efficiency of dendrimers sometimes restricts their native applications, as synthesis is too laborious, slow, and expensive. Also, subsequent generations can only be obtained over long periods of time, since the density, steric hindrance, and complexity are found to increase with the generation number, leading to hurdles in terms of gaining the pure product effortlessly. Similarly, higher generations of dendrimers are less reactive and it is quite problematic to tune dendrimeric composites.
Dendrimeric synthesis also induces some side reactions that limit the quality and purity of the products. However, progressive advancement over the past four decades in the field of dendrimers has helped to develop certain advanced artificial techniques to overshadow all such hurdles and problems. Traditional divergent/convergent methods can be tuned proficiently to propel advanced synthetic approaches, including click chemistry, solid-phase synthesis, one-pot synthesis, and double exponential methods, and these are a boon to researchers for amalgamating organic and inorganic moieties to fabricate well-designed dendrimeric products to cater to the needs of today and demands of tomorrow. Thus, dendrimeric exploitation is of great interest in current nanoscience and technology fields to take advantage of the opportunities for innovative paths. As a result, synthetic approaches must be developed to advance any future prospects.
With the successful implementation of advanced synthetic techniques combined with nanotechnology, there is some scope for developing enriched synthesis and purification methods for making many nanocomposites including dendrite frameworks for specific applications, like the treatment of deadly diseases, pollutant removal, gene transfer, and drug delivery. The numerous challenges in the domain of dendrimers offer an opportunity for researchers to improve the future of humankind.
Conflicts of interest
There are no conflicts to declare.
Acknowledgements
The authors thank the faculty of Post Graduate Teaching Department of Chemistry, R.T.M. Nagpur University Nagpur for providing all research support.
References
- P. J. Flory, J. Am. Chem. Soc., 1941, 63, 3084–3091 Search PubMed.
- P. J. Flory, J. Am. Chem. Soc., 1952, 74, 2718–2723 CrossRef CAS.
- E. Buhleier, W. Wehner and F. Vogtle, Synthesis, 1978, 2, 155–158 CrossRef.
-
D. A. Tomalia and J. R. Dewald, US Pat., 4507466, 1985 Search PubMed.
- D. A. Tomalia, H. Baker, J. Dewald, M. Hall, G. S. Martin, J. Roeck, J. Ryder and P. Smith, Polym. J., 1985, 17, 117–132 CrossRef CAS.
- K. Barbara and M. Bryszewska, Acta Biochim. Pol., 2001, 48, 199–208 CrossRef.
- M. Elsabahy, G. S. Heo, S. M. Lim, G. Sun and K. L. Wooley, Chem. Rev., 2015, 115, 10967–11011 CrossRef CAS PubMed.
- G. R. Newkome, Z. Yao, G. R. Baker and V. K. Gupta, J. Org. Chem., 1985, 50, 2003–2004 CrossRef CAS.
- E. Abbasi, S. F. Aval, A. Akbarzadeh, M. Milani, H. T. Nasrabadi, S. W. Joo, Y. Hanifehpour, K. Nejati-Koshki and R. Pashaei-Asl, Nanoscale Res. Lett., 2014, 9, 247 CrossRef.
- N. T. Pourianazar, P. Mutlu and U. Gunduz, J. Nanopart. Res., 2014, 16, 2342 CrossRef.
- E. Sorsaka, J. V. Valha, S. K. Urekb and A. Lobnik, Analyst, 2014, 00, 1–11 Search PubMed.
- S. L. Mekuria, T. A. Debele and H. C. Tsai, RSC Adv., 2016, 6, 63761–63772 RSC.
- B. K. Nanjwadea, H. M. Bechraa, G. K. Derkar, F. V. Manvi and V. K. Nanjwadea, Eur. J. Pharm. Sci., 2009, 38, 185–196 CrossRef.
- J. Yang, Q. Zhang, H. Chang and Y. Cheng, Chem. Rev., 2015, 115, 5274–5300 CrossRef CAS.
- Z. Qiaoa and X. Shi, Prog. Polym. Sci., 2015, 44, 1–2 CrossRef.
- D. Astruc and F. Chardac, Chem. Rev., 2001, 101, 2991–3023 CrossRef CAS PubMed.
- M. Sajid, M. K. Nazal, Ihsanullah, N. Baig and A. M. Osman, Sep. Purif. Technol., 2018, 191, 400–423 CrossRef CAS.
- D. A. Tomalia and J. M. J. Fréchet, J. Polym. Sci., Part A: Polym. Chem., 2002, 40, 2719–2728 CrossRef CAS.
-
J. Sebestik, M. Reinis and J. Jezek, in Biomedical Applications of Peptide, Glyco- and Glycopeptide Dendrimers, and Analogous Dendrimeric Structures, Springer, Vienna, 2012, ch. 6 Synthesis of Dendrimers: Convergent and Divergent Approaches, pp. 55–81 Search PubMed.
- Z. Lyu, L. Ding, A. Y. T. Huang, C. L. Kao and L. Peng, Mater. Today Chem., 2019, 13, 34–48 CrossRef CAS.
-
N. Feuerbacher and F. Vögtle, in Dendrimers. Topics in Current Chemistry, Iterative Synthesis in Organic Chemistry, Springer, Berlin, Heidelberg, 1998, vol. 197, pp. 1–18 Search PubMed.
- O. A. Matthews, A. N. Shipway and J. F. Stoddart, Prog. Polym. Sci., 1998, 23, 1–56 CrossRef CAS.
- M. Sowinska and Z. U. Lipkowska, New J. Chem., 2014, 38, 2168 RSC.
- M. V. Walter and M. Malkoch, Chem. Soc. Rev., 2012, 41, 4593–4609 RSC.
- U.-M. Wiesler, A. J. Berresheim, F. Morgenroth, G. Lieser and K. Mullen, Macromolecules, 2001, 34, 187–199 CrossRef CAS.
- I. Sohail, I. A. Bhatti, A. Ashar, F. M. Sarim, M. Mohsin, R. Naveed and A. Nazir, J. Mater. Res. Technol., 2020, 9, 498–506 CrossRef CAS.
- D. A. Tomalia, A. M. Naylor and W. A. Goddard, Angew. Chem., Int. Ed. Engl., 1990, 29, 138–175 CrossRef.
-
D. A. Tomalia and P. R. Dvornic, in Polymeric materials encyclopedia, ed. J. C. Salamone, CRC press, New York, 1996, vol. 3 ( D–E), pp. 1814–1830 Search PubMed.
- C. J. Hawker and J. M. J. Fréchet, J. Am. Chem. Soc., 1990, 112, 7638–7647 CrossRef CAS.
- S. M. Grayson and J. M. J. Frechet, Chem. Rev., 2001, 101, 38 CrossRef PubMed.
- J. W. Lee, J. H. Kim and B.-K. Kim, Tetrahedron Lett., 2006, 47, 2683–2686 CrossRef CAS.
- M. Pittelkow and J. B. Christensen, Org. Lett., 2005, 7, 1295–1298 CrossRef CAS.
- B. Thomas, C. Pifferi, G. C. Daskhan, M. Fiore, N. Berthet and O. Renaudet, Org. Biomol. Chem., 2015, 13, 11529–11538 RSC.
- P. R. Ashton, S. E. Boyd, C. L. Brown, N. Jayaraman, S. A. Nepogodiev and J. F. Stoddart, Chem. – Eur. J., 1996, 2, 1115–1128 CrossRef CAS.
- J. A. Jee, L. A. Spagnuolo and J. G. Rudick, Org. Lett., 2012, 14, 3292–3295 CrossRef CAS PubMed.
- T. M. Miller and T. X. Neenan, Chem. Mater., 1990, 2, 346–349 CrossRef CAS.
- P. R. Ashton, S. E. Boyd, C. L. Brown, N. Jayaraman and J. F. Stoddart, Angew. Chem., Int. Ed. Engl., 1997, 36, 732–735 CrossRef CAS.
- E. W. Kwock, T. X. Neenan and T. M. Miller, Chem. Mater., 1991, 3, 775–777 CrossRef CAS.
- K. Onitsuka, M. Fujimoto, H. Kitajima, N. Ohshiro, F. Takei and S. Takahashi, Chem. – Eur. J., 2004, 10, 6433–6446 CrossRef CAS PubMed.
- J. W. Lee, B.-K. Kim, H. J. Kim, S. C. Han, W. S. Shin and S.-H. Jin, Macromolecules, 2006, 39, 2418–2422 CrossRef CAS.
- J. W. Lee, J. H. Kim, B.-K. Kim, J. H. Kim, W. S. Shin and S.-H. Jin, Tetrahedron, 2006, 62, 9193–9200 CrossRef CAS.
- A. Morikawa, M. Kakimoto and Y. Imai, Macromolecules, 1992, 25, 3247–3253 CrossRef CAS.
- K. L. Wooley, C. J. Hawker and J. M. J. Frechet, J. Am. Chem. Soc., 1991, 113, 4252–4261 CrossRef CAS.
- Z. Xu, S. M. Kahr, K. L. Walker, C. L. Wilkins and J. S. Moore, J. Am. Chem. Soc., 1994, 116, 4537–4550 CrossRef CAS.
- G. L'Abbe, B. Forier and W. Dehaen, Chem. Commun., 1996, 2143–2144 RSC.
- B. Forier and W. Dehaen, Tetrahedron, 1999, 55, 9829–9846 CrossRef CAS.
- H. Ihre, A. Hult, J. M. J. Fréchet and I. Gitsov, Macromolecules, 1998, 31, 4061–4068 CrossRef CAS.
- Y. Ishida, M. Jikei and M. Kakimoto, Macromolecules, 2000, 33, 3202–3211 CrossRef CAS.
- Z. F. Shi, W.-Y. Chai, P. An and X. P. Cao, Org. Lett., 2009, 11, 4394–4397 CrossRef CAS PubMed.
- T. Kawaguchi, K. L. Walker, C. L. Wilkins and J. S. Moore, J. Am. Chem. Soc., 1995, 117, 2159–2165 CrossRef CAS.
- B. S. Balaji and M. R. Lewis, Chem. Commun., 2009, 4593 RSC.
- J. M. J. Frechet, I. Gitsov, R. B. Grubbs, C. J. Hawker, M. Hemni, M. Leduc, E. Sanford and K. Yui, Polym. Mater.: Sci. Eng., 1995, 73, 271 CAS.
- H. T. Cheang, C. T. Chen, T. Kondo, G. Siuzdak and K. B. Sharpless, Angew. Chem., Int. Ed. Engl., 1996, 35, 182 CrossRef.
- D. Zanini and R. Roy, J. Org. Chem., 1996, 61, 7348 CrossRef CAS PubMed.
-
(a) R. B. Merrifield, J. Am. Chem. Soc., 1963, 85, 2149–2154 CrossRef CAS;
(b) R. B. Merrifield, Angew. Chem., Int. Ed. Engl., 1985, 24, 799–810 CrossRef.
- N. J. Wells, A. Basso and M. Bradley, Biopolymers, 1998, 47, 381–396 CrossRef CAS.
- C. Rao and J. P. Tam, J. Am. Chem. Soc., 1994, 116, 6975–6976 CrossRef CAS.
- V. Swali, N. J. Wells, G. J. Langley and M. Bradley, J. Org. Chem., 1997, 62, 4902–4903 CrossRef CAS.
- A. Basso, M. Bradley, B. Evans and N. Pegg, Chem. Commun., 2001, 697–698 RSC.
- G. Sanclimens, L. Crespo, E. Giralt, M. Royo and F. Albericio, Biopolymers, 2004, 76, 283–297 CrossRef CAS PubMed.
- R. Spindler and J. M. J. Frechet, J. Chem. Soc., Perkin Trans. 1, 1993, 913–918 RSC.
- P. Goyal, K. Yoon and M. Weck, Chem. – Eur. J., 2007, 13, 8801–8810 CrossRef CAS PubMed.
- K. L. Killops, L. M. Campos and C. J. Hawker, J. Am. Chem. Soc., 2008, 130, 5062–5064 CrossRef CAS PubMed.
- H. F. Gaertner, F. Cerini, A. Kamath, A.-F. Rochat, C. A. Siegrist, L. Menin and O. Hartley, Bioconjugate Chem., 2011, 22, 1103–1114 CrossRef CAS PubMed.
- N. Kottari, Y. M. Chabre, T. C. Shiao, R. Rej and R. Roy, Chem. Commun., 2014, 50, 1983 RSC.
- F. Zeng and S. C. Zimmerman, J. Am. Chem. Soc., 1996, 118, 5326–5327 CrossRef CAS.
- S. K. Deb, T. M. Maddux and L. Yu, J. Am. Chem. Soc., 1997, 119, 9079–9080 CrossRef CAS.
- W. Wu, Z. Xu, W. Xiang and Z. Li, Polym. Chem., 2014, 5, 6667–6670 RSC.
- M. Arseneault, C. Wafer and J. F. Morin, Molecules, 2015, 20, 9263–9294 CrossRef CAS PubMed.
- H. C. Kolb, M. G. Finn and K. B. Sharpless, Angew. Chem., Int. Ed., 2001, 40, 2004–2021 CrossRef CAS.
- J. E. Moses and A. D. Moorhouse, Chem. Soc. Rev., 2007, 36, 1249–1262 RSC.
- C. E. Hoyle and C. N. Bowman, Angew. Chem., Int. Ed., 2010, 49, 1540–1573 CrossRef CAS PubMed.
- W. H. Binder and R. Sachsenhofer, Macromol. Rapid Commun., 2007, 28, 15–54 CrossRef CAS.
- B. M. Rosen, G. Lligadas, C. Hahn and V. Percec, J. Polym. Sci., Part A: Polym. Chem., 2009, 47, 3931–3939 CrossRef CAS.
- P. Wu, A. K. Feldman, A. K. Nugent, C. J. Hawker, A. Scheel, B. Voit and V. V. Fokin, Angew. Chem., Int. Ed., 2004, 43, 3928–3932 CrossRef CAS PubMed.
- D. Astruc, L. Liang, A. Rapakousiou and J. Ruiz, Acc. Chem. Res., 2011, 45, 630–640 CrossRef PubMed.
- P. Wu, M. Malkoch, J. N. Hunt, R. Vestberg, E. Kaltgrad, M. G. Finn and C. J. Hawker, Chem. Commun., 2005, 5775 RSC.
- R. Kikkeri, X. Liu, A. Adibekian, Y. H. Tsai and P. H. Seeberger, Chem. Commun., 2010, 46, 2197 RSC.
- S. L. Elmer, S. Man and S. C. Zimmerman, Eur. J. Org. Chem., 2008, 2008, 3845–3851 CrossRef PubMed.
- W. Xi, T. F. Scott, C. J. Kloxin and C. N. Bowman, Adv. Funct. Mater., 2014, 24, 2572–2590 CrossRef CAS.
- G. Franc and A. Kakkar, Chem. Commun., 2008, 5267–5276 RSC.
- F. Himo, T. Lovell, R. Hilgraf, V. V. Rostovtsev, L. Noodleman, K. B. Sharpless and V. V. Fokin, J. Am. Chem. Soc., 2005, 127, 210–216 CrossRef CAS PubMed.
- V. Castro, H. Rodriguez and F. Albericio, ACS Comb. Sci., 2016, 18, 1 CrossRef CAS PubMed.
- A. K. Agrahari, A. S. Singh, R. Mukherjee and V. K. Tiwari, RSC Adv., 2020, 10, 31553 RSC.
- M. A. Tasdelen, Polym. Chem., 2011, 2, 2133 RSC.
- G. Hizal, U. Tunca and A. Sanyal, J. Polym. Sci., Part A: Polym. Chem., 2011, 49, 4103–4120 CrossRef CAS.
- M. M. Kose, G. Yesilbag and A. Sanyal, Org. Lett., 2008, 10, 2353–2356 CrossRef CAS PubMed.
- A. B. Lowe, Polym. Chem., 2010, 1, 17–36 RSC.
- I. A. Olayiwola, B. Mamba and U. Feleni, Mater. Chem. Phys., 2020, 241, 122641 Search PubMed.
- Y. Chabre and R. Roy, Curr. Top. Med. Chem., 2008, 8, 1237–1285 CrossRef CAS PubMed.
- W. Maes and W. Dehaen, Eur. J. Org. Chem., 2009, 2009, 4719–4752 CrossRef.
- S.-H. Hwang, C. D. Shreiner, C. N. Moorefield and G. R. Newkome, New J. Chem., 2007, 31, 1192 RSC.
- J. Nithyanandhan and N. Jayaraman, J. Org. Chem., 2002, 67, 6282–6285 CrossRef CAS PubMed.
- V. Darcos, A. Duréault, D. Taton, Y. Gnanou, P. Marchand, A. M. Caminade and F. Leising, Chem. Commun., 2004, 2110–2111 RSC.
- P. M. Welch and C. F. Welch, Macromolecules, 2009, 42, 7571–7578 CrossRef CAS.
- H. Lang and B. Luhmann, Adv. Mater., 2001, 13, 1523 CrossRef CAS.
- K. Sadler and J. P. Tam, Rev. Mol. Biotechnol., 2002, 90, 195–229 CrossRef CAS PubMed.
- B. Romagnoli and W. Hayes, J. Mater. Chem., 2002, 12, 767–799 RSC.
- P. Lundberg, C. J. Hawker, A. Hult and M. Malkoch, Macromol. Rapid Commun., 2008, 29, 998 CrossRef CAS.
-
M. Malkoch and S. García-Gallego, in Dendrimer Chemistry: Synthetic Approaches Towards Complex Architectures, Royal Society of Chemistry, London, UK, 2020, ch.1 Introduction to Dendrimers and Other Dendritic Polymers, pp. 1–20 Search PubMed.
- M. A. N. Virboul, M. Lutz, M. A. Siegler, A. L. Spek, G. V. Koten and R. J. M. Klein Gebbink, Chem. – Eur. J., 2009, 15, 9981–9986 CrossRef CAS PubMed.
- S. Garcia Gallego, O. C. J. Andrén and M. Malkoch, J. Am. Chem. Soc., 2020, 142, 1501–1509 CrossRef CAS PubMed.
-
J. M. J. Fréchet and D. A. Tomalia, Dendrimers and Other Dendritic Polymers, John Wiley & Sons Ltd, 2001, Laboratory Synthesis of Poly(amidoamine)(PAMAM) Dendrimers, pp. 587–604 Search PubMed.
- I. J. Majoros, C. R. Williams, D. A. Tomalia and J. R. Baker, Macromolecules, 2008, 41, 8372–8379 CrossRef CAS PubMed.
- P. B. Sherly Mole, S. George, A. M. Shebitha, V. Kannan, S. Mathew, K. K. Asha and K. Sreekumar, Tetrahedron, 2019, 130676 CrossRef.
- J. T. Rundel, B. K. Paul and V. T. Remcho, J. Chromatogr. A, 2007, 1162, 167–174 CrossRef CAS PubMed.
- D. G. Mullen, A. Desai, M. A. Van Dongen, M. Barash, J. R. Baker and M. M. Banaszak Holl, Macromolecules, 2012, 45, 5316–5320 CrossRef CAS PubMed.
- I. Das, H. J. Borah, D. Sarma and S. Hazarika, J. Macromol. Sci., Part A: Pure Appl. Chem., 2018, 5, 1–8 Search PubMed.
- A. Caminade, R. Laurent and J. Majoral, Adv. Drug Delivery Rev., 2005, 57, 2130–2146 CrossRef CAS PubMed.
- V. Biricova and A. Laznickova, Bioorg. Chem., 2009, 37, 185–192 CrossRef CAS PubMed.
- S. Pande and R. M. Crooks, Langmuir, 2011, 27, 9609–9613 CrossRef CAS PubMed.
- M. S. Refat, A. M. El-Didamony and I. Grabchev, Spectrochim. Acta, Part A, 2007, 67, 58–65 CrossRef PubMed.
- M.-C. Popescu, D. Filip, C. Vasile, C. Cruz, J. M. Rueff, M. Marcos and G. Singurel, J. Phys. Chem. B, 2006, 110, 14198–14211 CrossRef CAS PubMed.
- V. L. Furer, A. E. Vandyukov, J. P. Majoral, A. M. Caminade and V. I. Kovalenko, J. Mol. Struct., 2009, 919, 366–372 CrossRef CAS.
- K. X. Moreno and E. E. Simanek, Macromolecules, 2008, 41, 4108–4114 CrossRef CAS PubMed.
- M. V. Gomez, J. Guerra, V. S. Myers, R. M. Crooks and A. H. Velders, J. Am. Chem. Soc., 2009, 131, 14634–14635 CrossRef CAS PubMed.
- M. V. Gomez, J. Guerra, A. H. Velders and R. M. Crooks, J. Am. Chem. Soc., 2009, 131, 341–350 CrossRef CAS PubMed.
- D. Banerjee, M. A. C. Broeren, M. H. P. van Genderen, E. W. Meijer and P. L. Rinaldi, Macromolecules, 2004, 37, 8313–8318 CrossRef CAS.
- V. L. Furer, J. P. Majoral, A. M. Caminade and V. I. Kovalenko, Polymer, 2004, 45, 5889–5895 CrossRef CAS.
- H. T. Temiz, I. H. Boyaci, I. Grabchev and U. Tamer, Spectrochim. Acta, Part A, 2013, 116, 339–347 CrossRef CAS PubMed.
- T. A. Saleh, M. M. Al-Shalalfeh and A. A. Al-Saadi, Sci. Rep., 2016, 6, 32185 CrossRef CAS PubMed.
- J. Peterson, V. Allikmaa, J. Subbi, T. Pehk and M. Lopp, Eur. Polym. J., 2003, 39, 33–42 CrossRef CAS.
- L. Zhou, D. H. Russell, M. Zhao and R. M. Crooks, Macromolecules, 2001, 34, 3567–3573 CrossRef CAS.
- B. Baytekin, N. Werner, F. Luppertz, M. Engeser, J. Brüggemann, S. Bitter and C. A. Schalley, Int. J. Mass Spectrom., 2006, 249–250, 138–148 CrossRef.
- C. A. Schalley, B. Baytekin, H. T. Baytekin, M. Engeser, T. Felder and A. Rang, J. Phys. Org. Chem., 2006, 19, 479–490 CrossRef CAS.
- J. R. Lloyd, P. S. Jayasekara and K. A. Jacobson, Anal. Methods, 2016, 8, 263–269 RSC.
- C. Demathieu, M. M. Chehimi, J. F. Lipskier, A.-M. Caminade and J. P. Majoral, Appl. Spectrosc., 1999, 53, 1277–1281 CrossRef CAS.
- M. R. Knecht, M. G. Weir, V. S. Myers, W. D. Pyrz, H. Ye, V. Petkov and R. M. Crooks, Chem. Mater., 2008, 20, 5218–5228 CrossRef CAS.
- D. R. Baer, D. J. Gaspar, P. Nachimuthu, S. D. Techane and D. G. Castner, Anal. Bioanal. Chem., 2010, 396, 983–1002 CrossRef CAS PubMed.
- J. W. Kriesel, S. König, M. A. Freitas, A. G. Marshall, J. A. Leary and T. D. Tilley, J. Am. Chem. Soc., 1998, 120, 12207–12215 CrossRef CAS.
- V. Petkov, V. Parvanov, D. Tomalia, D. Swanson, D. Bergstrom and T. Vogt, Solid State Commun., 2005, 134, 671–675 CrossRef CAS.
- Z. Pan, M. Xu, E. Y. Cheung, K. D. M. Harris, E. C. Constable and C. E. Housecroft, J. Phys. Chem. B, 2006, 110, 11620–11623 CrossRef CAS PubMed.
- M. F. Ottaviani, B. Sacchi, N. J. Turro, W. Chen, S. Jockusch and D. A. Tomalia, Macromolecules, 1999, 32, 2275–2282 CrossRef CAS.
- M. F. Ottaviani, M. Cangiotti, A. Fattori, C. Coppola, S. Lucchi, M. Ficker and J. B. Christensen, J. Phys. Chem. B, 2013, 117, 14163–14172 CrossRef CAS PubMed.
- H. J. Han, K. B. Sebby, D. J. Singel and M. J. Cloninger, Macromolecules, 2007, 40, 3030–3033 CrossRef CAS.
- X. Wang, L. Guerrand, B. Wu, X. Li, L. Boldon, W.-R. Chen and L. Liu, Polymer, 2012, 4, 600–616 Search PubMed.
- F. Zeng, S. C. Zimmerman, S. V. Kolotuchin, D. E. Reichert and Y. Ma, Tetrahedron, 2002, 58, 825–843 CrossRef CAS.
- R. C. Hedden and B. J. Bauer, Macromolecules, 2003, 36, 1829–1835 CrossRef CAS.
- D. Pötschke, M. Ballauff, P. Lindner, M. Fischer and F. Vögtle, Macromolecules, 1999, 32, 4079–4087 CrossRef.
- N. C. Beck Tana, L. Baloghb, S. F. Trevinoa, D. A. Tomalia and J. S. Lin, Polymer, 1999, 40, 2537–2545 CrossRef.
- T. J. Prosa, B. J. Bauer, E. J. Amis, D. A. Tomalia and R. Scherrenberg, J. Polym. Sci., Part B: Polym. Phys., 1997, 35, 2913–2924 CrossRef CAS.
- X. Shi, K. Sun, L. P. Balogh and J. R. Baker, Nanotechnology, 2006, 17, 4554–4560 CrossRef CAS.
- L. M. Bronstein and Z. B. Shifrina, Chem. Rev., 2011, 111, 5301–5344 CrossRef CAS PubMed.
- C. Li, D. Li, J. Zhou and S. Feng, Polym. Bull., 2006, 58, 963–968 CrossRef.
- Y. Gu, H. Xie, J. Gao, D. Liu, C. T. Williams, C. J. Murphy and H. J. Ploehn, Langmuir, 2005, 21, 3122–3131 CrossRef CAS PubMed.
- R. Pericet-Camara, G. Papastavrou and M. Borkovec, Langmuir, 2004, 20, 3264–3270 CrossRef CAS PubMed.
- J. Martinovic, A.-M. Chiorcea-Paquim, V. C. Diculescu, J. Van Wyk, E. Iwuoha, P. Baker and A.-M. Oliveira-Brett, Electrochim. Acta, 2008, 53, 4907–4919 CrossRef CAS.
- A. Sharma, D. K. Mohanty, A. Desai and R. Ali, Electrophoresis, 2003, 24, 2733–2739 CrossRef CAS PubMed.
- X. Shi, A. K. Patri, W. Lesniak, M. T. Islam, C. Zhang, J. R. Baker and L. P. Balogh, Electrophoresis, 2005, 26, 2960–2967 CrossRef CAS PubMed.
- H. M. Brothers II, L. T. Piehler and D. A. Tomalia, J. Chromatogr. A, 1998, 814, 233–246 CrossRef.
- R. Juárez, R. Gómez, J. L. Segura and C. Seoane, Tetrahedron Lett., 2005, 46, 8861–8864 CrossRef.
- C. M. Cardona and A. E. Kaifer, J. Am. Chem. Soc., 1998, 120, 4023–4024 CrossRef CAS.
- C. A. Cason, S. A. Oehrle, T. A. Fabré, C. D. Girten, K. A. Walters, D. A. Tomalia and H. A. Bullen, J. Nanomater., 2008, 2008, 1–7 CrossRef.
- H. G. Barth, C. Jackson and B. E. Boyes, Anal. Chem., 1994, 66, 595–620 CrossRef PubMed.
- P. L. Dubin, S. L. Edwards, J. I. Kaplan, M. S. Mehta, D. Tomalia and J. Xia, Anal. Chem., 1992, 64, 2344–2347 CrossRef CAS PubMed.
- C. Zhang, P. Su, M. Umar Farooq, Y. Yang, X. Gao and E. Hongjun, React. Funct. Polym., 2010, 70, 129–133 CrossRef CAS.
- A. S. Erturk, M. U. Gürbüz, M. Tülü and A. E. Bozdoğan, RSC Adv., 2015, 5, 60581–60595 RSC.
- R. E. H. Ramirez, I. V. Lijanova, N. V. Likhanova and O. O. Xometl, J. Inclusion Phenom. Macrocyclic Chem., 2015, 84, 49–60 CrossRef.
- E. Canetta and G. Maino, Nucl. Instrum. Methods Phys. Res., Sect. B, 2004, 213, 71–74 CrossRef CAS.
- P. K. Maiti, T. Çagın, G. Wang and W. A. Goddard, Macromolecules, 2004, 37, 6236–6254 CrossRef CAS.
- P. K. Maiti, T. Çagın, S. T. Lin and W. A. Goddard, Macromolecules, 2005, 38, 979–991 CrossRef CAS.
- Y. Liu, V. S. Bryantsev, M. S. Diallo and W. A. Goddard III, J. Am. Chem. Soc., 2009, 131, 2798–2799 CrossRef CAS PubMed.
- M. Han, P. Chen and X. Yang, Polymer, 2005, 46, 3481–3488 CrossRef CAS.
- B. K. Nanjwade, H. M. Bechra, G. K. Derkar, F. V. Manvi and V. K. Nanjwade, Eur. J. Pharm. Sci., 2009, 38, 185–196 CrossRef CAS PubMed.
- D. A. Tomalia, H. Baker, M. Hall, G. Kallos, S. Martin, J. Ryder and P. Smith, Macromolecules, 1986, 19, 2466–2468 CrossRef CAS.
- D. A. Tomalia, V. B. Hall, M. Hall and D. M. Hedstrand, Macromolecules, 1987, 20, 1164–1167 CrossRef CAS.
- J. W. Lee, H. J. Kim, S. C. Han, J. H. Kim and S. H. Jin, J. Nanosci. Nanotechnol., 2008, 8, 4635–4639 CrossRef CAS PubMed.
- A. Y. T. Huang, C. H. Tsai, H. Y. Chen, H. T. Chen, C. Y. Lu, Y. T. Lin and C. L. Kao, Chem. Commun., 2013, 49, 5784–5786 RSC.
- C. L. Kao, A. Y. T. Huang and H. T. Chen, Macromol. Rapid Commun., 2017, 38, 1700062 CrossRef PubMed.
- C. C. Lee, J. A. MacKay, J. M. J. Frechet and F. C. Szoka, Nat. Biotechnol., 2005, 23, 1517–1526 CrossRef CAS PubMed.
- G. Ghasemzadeh, M. Momenpour, F. Omidi, M. R. Hosseini, M. Ahani and A. Barzegari, Front. Environ. Sci. Eng., 2014, 8, 471–482 CrossRef CAS.
- E. De Jesús and J. C. Flores, Ind. Eng. Chem. Res., 2008, 47, 7968–7981 CrossRef.
- S. R. Barman, A. Nain, S. Jain, N. Punjabi, S. Mukherji and J. Satija, J. Mater. Chem. B, 2018, 6, 2368–2384 RSC.
- Y. Fan, W. Sun and X. Shi, Small Methods, 2017, 1, 1700224 CrossRef.
- P. G. Z. Benini, B. R. McGarvey and D. W. Franco, Nitric Oxide, 2008, 19, 245–251 CrossRef CAS PubMed.
- A. Boni, G. Bardi, A. Bertero, V. Cappello, M. Emdin, A. Flori and V. Piazza, Nanoscale, 2015, 7, 7307–7317 RSC.
- M. Bonizzoni, S. R. Long, C. Rainwater and E. V. Anslyn, J. Org. Chem., 2012, 77, 1258–1266 CrossRef CAS PubMed.
- L. M. Bronstein and Z. B. Shifrina, Nanotechnol. Russ., 2003, 4, 576–608 CrossRef.
- X. Guo and L. Huang, Acc. Chem. Res., 2011, 45, 971–979 CrossRef PubMed.
- M. E. Davis, Curr. Opin. Biotechnol., 2002, 13, 128–131 CrossRef CAS PubMed.
- D. Shcharbin, A. Shakhbazau and M. Bryszewska, Expert Opin. Drug Delivery, 2013, 10, 1687–1698 CrossRef CAS PubMed.
- J. D. Eichman, A. U. Bielinska, J. F. Kukowska-Latallo and J. R. Baker, Pharm. Sci. Technol. Today, 2000, 3, 232–245 CrossRef CAS PubMed.
- E. R. Figueroa, A. Y. Lin, J. Yan, L. Luo, A. E. Foster and R. A. Drezek, Biomaterials, 2014, 35, 1725–1734 CrossRef CAS PubMed.
- F. Abedi-Gaballu, G. Dehghan, M. Ghaffari, R. Yekta, S. Abbaspour-Ravasjani, B. Baradaran and M. R. Hamblin, Appl. Mater. Today, 2018, 12, 177–190 CrossRef PubMed.
- J. Lee, S. Lee, Y. E. Kwon, Y. J. Kim and J. S. Choi, Macromol. Res., 2019, 27, 360–368 CrossRef CAS.
- Z. Aydin, F. Akbas, M. Senel and S. N. Koc, J. Biomed. Mater. Res., Part A, 2012, 100, 2623–2628 CrossRef PubMed.
- X. Liu, D. Ma, H. Tang, L. Tan, Q. Xie, Y. Zhang, M. Ma and S. Yao, ACS Appl. Mater. Interfaces, 2014, 6, 8173–8183 CrossRef CAS PubMed.
- R. Esfand and D. A. Tomalia, Drug Discovery Today, 2001, 6, 427–436 CrossRef CAS PubMed.
- P. Singh, U. Gupta, A. Asthana and N. K. Jain, Bioconjugate Chem., 2008, 19, 2239–2252 CrossRef CAS PubMed.
- Y. Y. Jiang, G. T. Tang, L. H. Zhang, S. Y. Kong, S. J. Zhu and Y. Y. Pei, J. Drug Targeting, 2010, 18, 389–403 CrossRef CAS PubMed.
- M. A. Kaczorowska and H. J. Cooper, J. Am. Soc. Mass Spectrom., 2009, 20, 674–681 CrossRef CAS PubMed.
- F. Grohn, G. Kim, B. J. Bauer and E. J. Amis, Macromolecules, 2001, 34, 2179–2185 CrossRef.
- S. Antebi, P. Arya, L. E. Manzer and H. Alper, J. Org. Chem., 2002, 67, 6623–6631 CrossRef CAS PubMed.
- L. Balogh and D. A. Tomalia, J. Am. Chem. Soc., 1998, 120, 7355–7356 CrossRef CAS.
- S. Bharathi, P. T. Wong, A. Desai, O. Lykhytska, V. Choe, H. Kim and S. K. Choi, J. Mater. Chem. B, 2014, 2, 1068 RSC.
- J. Bua, Z. M. A. Judeh, C. B. Ching and S. Kawi, Catal. Lett., 2003, 85, 3–4 Search PubMed.
- D. Yang, R. Zhang, T. Zhao, T. Sun, X. Chu, S. Liu, E. Tang and X. Xu, Catal. Sci. Technol., 2019, 9, 6145–6151 RSC.
- A. M. Caminade, A. Ouali, M. Keller and J. P. Majoral, Chem. Soc. Rev., 2012, 41, 4113 RSC.
-
F. A. Aouada and M. R. de Moura, in Nanotechnologies in Food and Agriculture, Springer International Publishing, Switzerland, 2015, vol. 5, pp. 103–118 Search PubMed.
- P. L. Chariou, O. A. Ortega-Rivera and N. F. Steinmetz, ACS Nano, 2020, 14, 2678–2701 CrossRef CAS PubMed.
-
T. Sinha, P. Bhagwatwar, C. Krishnamoorthy and R. Chidambaram, Polymers for Agri-Food Applications, ed. T. Gutiérrez, Springer, Cham., Switzerland AG, 2019, pp. 5–28 Search PubMed.
-
L. D. Bunderson, B. L. Hunter and K. J. Isaacson, Patent Application Publication, US2020021636716/734021A1, 2020.
- X. Liu, B. He, Z. Xu, M. Yin, W. Yang, H. Zhang, J. Cao and J. Shen, Nanoscale, 2015, 7, 445–449 RSC.
- B. P. Cahill, G. Papastavrou, G. J. M. Koper and M. Borkovec, Langmuir, 2008, 24, 465–473 CrossRef CAS PubMed.
- A. S. Ertürk, J. Iran. Chem. Soc., 2018, 15, 1685–1698 CrossRef.
- M. F. Abou Taleb, A. El-Trass and S. El-Sigeny, Polym. Adv. Technol., 2015, 26, 994–1002 CrossRef CAS.
- S. Aliannejadi, A. H. Hassani, H. A. Panahi and S. M. Borghei, Microchem. J., 2018, 145, 767–777 CrossRef.
- X. Bao, Q. Wu, W. Shi, W. Wang, H. Yu, Z. Zhu and F. Cui, Water Res., 2019, 150, 1–10 CrossRef PubMed.
- M. B. Camarada, M. Zuniga, J. Alzate-Morales and L. S. Santos, Chem. Phys. Lett., 2014, 616–617, 171–177 CrossRef CAS.
- Y. Zhou, L. Luan, B. Tang, Y. Niu, R. Qu, Y. Liu and W. Xu, Chem. Eng. J., 2020, 398, 125651 CrossRef CAS.
- E. Sorsak, J. V. Valh, S. K. Urek and A. Lobnik, Analyst, 2015, 140, 976–989 RSC.
- J. Satija, V. V. R. Sai and S. Mukherji, J. Mater. Chem., 2011, 21, 14367 RSC.
- E. B. Bahadır and M. K. Sezginturk, Talanta, 2016, 148, 427–438 CrossRef PubMed.
-
A. Turner, G. Wilson and I. Kaube, in Biosensors: Fundamentals and Applications, Oxford University Press, Oxford, New York, 1987, vol. 1, p. 770 Search PubMed.
- H. Karimi-Maleh, F. Karimi, M. Alizadeh and A. L. Sanat, Chem. Rec., 2020, 20, 1–12 CrossRef PubMed.
- M. Prasad, H. Mohan, Manjit, Ravina, B. Brar, S. Mohammad and C. S. Pundir, Curr. Top. Med. Chem., 2020, 20, 1029–1041 CrossRef PubMed.
- M. Şenel and C. Nergiz, Synth. Met., 2012, 162, 688–694 CrossRef.
- Z.-M. Liu, Y. Yang, H. Wang, Y.-L. Liu, G.-L. Shen and R.-Q. Yu, Sens. Actuators, B, 2005, 106, 394–400 CrossRef CAS.
- N. Zhu, Y. Gu, Z. Chang, P. He and Y. Fang, Electroanalysis, 2006, 18, 2107–2114 CrossRef CAS.
- M. Şenel, C. Nergiz and E. Çevik, Sens. Actuators, B, 2013, 176, 299–306 CrossRef.
- G. Castillo, K. Spinella, A. Poturnayová, M. Šnejdárková, L. Mosiello and T. Hianik, Food Control, 2015, 52, 9–18 CrossRef CAS.
- M. Elancheziyan and S. Senthilkumar, Appl. Surf. Sci., 2019, 495, 143540 CrossRef CAS.
- N. A. S. Omar, Y. W. Fen, J. Abdullah, Y. Mustapha Kamil, W. M. E. M. M. Daniyal, A. R. Sadrolhosseini and M. A. Mahdi, Sci. Rep., 2020, 10, 2374 CrossRef CAS PubMed.
- L. H. Bryant, M. W. Brechbiel, C. Wu, J. W. M. Bulte, V. Herynek and J. A. Frank, J. Magn. Reson. Imaging, 1999, 9, 348–352 CrossRef PubMed.
- C. Kojima, B. Turkbey, M. Ogawa, M. Bernardo, C. A. S. Regino, L. H. Bryant and H. Kobayashi, Nanomed.: Nanotechnol., Biol. Med., 2011, 7, 1001–1008 CrossRef CAS PubMed.
- S. L. Mekuria, T. A. Debele and H.-C. Tsai, ACS Appl. Mater. Interfaces, 2017, 9, 6782–6795 CrossRef CAS PubMed.
- T. Almasi, N. Gholipour, M. Akhlaghi, A. M. Kheirabadi, S. M. Mazidi, S. H. Hosseini, P. Geramifar, D. Beiki, N. Rostampour and D. S. Gahrouei, Int. J. Polym. Mater. Polym. Biomater., 2020, 1–13 Search PubMed.
|
This journal is © The Royal Society of Chemistry 2022 |
Click here to see how this site uses Cookies. View our privacy policy here.