DOI:
10.1039/D1SC05913K
(Edge Article)
Chem. Sci., 2022,
13, 111-117
Reusable Co-nanoparticles for general and selective N-alkylation of amines and ammonia with alcohols†
Received
27th October 2021
, Accepted 26th November 2021
First published on 29th November 2021
Abstract
A general cobalt-catalyzed N-alkylation of amines with alcohols by borrowing hydrogen methodology to prepare different kinds of amines is reported. The optimal catalyst for this transformation is prepared by pyrolysis of a specific templated material, which is generated in situ by mixing cobalt salts, nitrogen ligands and colloidal silica, and subsequent removal of silica. Applying this novel Co-nanoparticle-based material, >100 primary, secondary, and tertiary amines including N-methylamines and selected drug molecules were conveniently prepared starting from inexpensive and easily accessible alcohols and amines or ammonia.
Introduction
In organic synthesis, carbon–nitrogen bond forming reactions represent a valuable synthetic toolbox, which allows direct access to all kinds of amines.1–11 In general, amines are indispensable fine and bulk chemicals, widely used as precursors and intermediates for many daily life products.3–5,12–14 In addition, amine/nitrogen moieties constitute integral parts of most drugs and biomolecules and play a key role for their activities.13,14 Because of their importance a plethora of methods have been developed to create C–N bonds. Traditionally, stoichiometric nucleophilic substitution reactions and reductive aminations with (over)stoichiometric amounts of metal hydrides prevailed in this area.3–5 In recent decades and today, the focus for C–N bond formation is more on catalytic methodologies due to improved selectivity control and waste minimization. Among these methods, alcohol aminations (N-alkylation with alcohols; Scheme 1)8–12 as well as hydroaminations of olefins6 are especially interesting due to their intrinsic atom efficiency. In particular, amination of alcohols via so-called borrowing hydrogen (BH) or hydrogen auto-transfer methodology7–11 offers the following advantages: (a) utilization of alcohols as the starting materials, which are inexpensive and easily accessible including bio-based feedstocks; (b) no need for any external/additional hydrogen as the alcohol itself serves as the hydrogen donor; and (c) high step- and atom-economy as water is the only by-product.
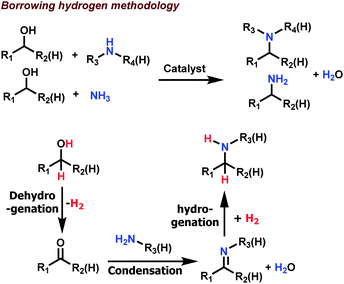 |
| Scheme 1 Borrowing hydrogen methodology for carbon–nitrogen bond formation from alcohols. | |
In Scheme 1, the generally accepted mechanism for amination of alcohols is shown: first the alcohol undergoes catalytic dehydrogenation to give the corresponding aldehyde (or ketone), which is then condensed with the amine to form the respective imine. Finally, this imine is hydrogenated to yield the desired amine product.
A variety of molecularly-defined catalysts have been successfully developed for catalytic N-alkylation of amines with alcohols;15–21 however, in recent years there is a special focus on 3d metal complexes.16–21 While many of these homogeneous systems offer broad substrate scope and general applicability, their drawbacks are stability and difficult recycling. On the other hand, heterogeneous catalysts22 for hydrogen borrowing reactions including supported Ni,23–27 Fe-,28 and Cu-based27,29–32 systems are stable, but have been scarcely applied for advanced organic synthesis. Thus, the development of heterogeneous catalysts, which are stable, inexpensive and at the same time exhibit both generality and high selectivity for hydrogen barrowing reactions is still desirable.
In recent years, we and others have explored metal complexes with nitrogen-containing ligands and metal organic frameworks (MOFs) with different linkers as suitable precursors for the preparation of supported metal nanoparticles.33–38 After controlled pyrolysis, well dispersed metal nitrogen carbon structures are formed. The resulting materials were successfully applied for many hydrogenation,33 oxidation,34 and reductive amination reactions.35,36 However, to the best of our knowledge such materials have not been explored for N-alkylation of amines with alcohols. In this respect, herein we report novel supported cobalt-nanoparticles as a general hydrogen borrowing catalyst for the preparation of amines. The optimal nanocatalyst is prepared by pyrolysis of a templated material obtained in situ from cobalt–phenanthroline complex and commercially available colloidal silica (LUDOX® HS-40; 40 wt% suspension in H2O) and subsequent removal of silica content. Aromatic, heterocyclic, and aliphatic secondary and tertiary amines can be conveniently and selectively prepared starting using various alcohols. Further, this novel catalyst is also active and selective for the amination of alcohols with ammonia.
Results and discussion
Preparation and catalytic evaluation of Co-nanoparticles
At the start of this project, we prepared several potential cobalt catalysts via co-precipitation of Co(NO3)2·6H2O in the presence of di- and tri-dentate nitrogen ligands (1,10-phenanthroline (phen; L1), 2,2′-bipyridine (bpy; L2), 2,2'; 6′,2′′-terpyridine (tpy; L3), 2,6-bis(2-benzimidazolyl)pyridine (bbp; L4)) and colloidal silica (LUDOX® HS-40; 40 wt% suspension in H2O) (Fig. 1, steps 1 and 2). After pyrolysis of the templated material containing cobalt–nitrogen complexes on silica under argon at 800 °C (Fig. 1, step 3), the residual silica was removed via treatment with ammonium hydrogen fluoride (NH4HF2) (Fig. 1, step 4). The details of the synthetic procedure are described in SI. The resulting cobalt materials are represented as Co@NC-T-L, where T and L denotes pyrolysis temperature and ligand, respectively.
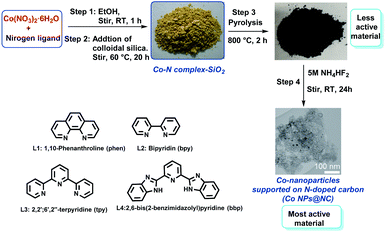 |
| Fig. 1 Preparation of novel cobalt nanoparticles supported on N-doped carbon. | |
The activities and selectivities of these Co-materials (Co@NC-T-L) were initially evaluated for the N-alkylation of aniline 1 with benzyl alcohol 2 to prepare N-benzylaniline 3 in presence of 1 equivalent of t-BuOK at 140 °C for 24 h in toluene (Fig. 2; Table S1†). Under these conditions all the prepared catalysts from different Co–nitrogen complexes showed good to excellent activities and selectivities for the benchmark reaction (Fig. 2; Table S1,† entries 1–4). Among the prepared materials, Co@NC-800-L1 was found to be the best and quantitative yield of the desired product 3 was obtained (Fig. 2; Table S1,† entry 1). Comparing the materials prepared at different pyrolysis temperature (400–1000 °C) revealed 800 °C as the best one (Fig. 2; Table S1,† entries 1, 5–7).
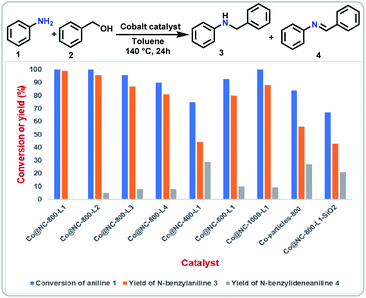 |
| Fig. 2
N-Alkylation of aniline with benzyl alcohol: Testing of cobalt catalysts. Reaction conditions: 0.5 mmol aniline, 1 mmol benzyl alcohol, 15 mg catalyst (0.86 mol% Co), 0.5 mmol t-BuOK (1 equiv.), 2 mL toluene, 140 °C, 24 h. Conversions and yields are based on aniline and determined by GC using n-hexadecane standard. | |
The material prepared using the cobalt nitrate precursor, without any ligand, was found to be less active and produced 56% of the desired product (Fig. 2; Table S1,† entry 8). Similarly, the pyrolyzed material with silica (Co@NC-800-L1-SiO2) exhibited lower activity and provided 43% yield of 3 (Fig. 2; Table S1,† entry 9). Applying Co@NC-800-L1, the model reaction is also promoted in the absence of base and gave 57% of N-benzylaniline 3 (Table S1,† entry 10), while without cobalt catalyst only minor amounts of 3 are formed (Table S1,† entry 11), which is in accordance with previous observations.39,40 Further, different solvents, bases, and other reaction parameters such as temperature, amount of catalyst and reaction time were evaluated for the model reaction (Tables S2–S4†). Next, we compared the activity of our optimal catalyst with selected previously reported catalysts for alcohol aminations. As shown in Table S5,† Co@NC-800-L1 worked more efficiently and required less amount of catalyst loading than the compared systems.
Characterization of Co-based materials
The optimal catalyst (Co@NC-800-L1) and a less active material (Co-particles-800; without ligand) as well as a recycled (Co@NC-800-L1-R) catalyst were characterized using X-ray powder diffraction (XRD), scanning transmission electron microscopy (TEM), high resolution transmission electron microscopy (HRTEM), high-angle annular dark-field scanning TEM (HAADF-STEM), X-ray spectroscopy (EDS) and X-ray photoelectron spectroscopy (XPS). The X-ray diffraction pattern of the most active catalyst (Co@NC-800-L1) showed peaks at 2θ = 44.40° and 2θ = 51.6°, which are assigned to metallic cobalt nanoparticles of fcc cobalt (JCPDS 15-0806) and hcp cobalt (JCPDS 05-0727)41 (Fig. S1†). The broad peak observed in the range of 2θ = 20–30.8° belongs to reflections of graphitized carbon (Fig. S1†). In the XRD of the recycled catalyst sample, Co@NC-800-L1-R, identical cobalt species similar to the fresh catalyst are detected. In the less active sample (Co-particles-800), which was prepared without any ligand, the formation of cobalt silicate phases is observed (Fig. S2†).
TEM analysis of Co@NC-800-L1 showed the formation of small cobalt nanoparticles with a spherical morphology and the size up to ∼12 nm (Fig. 3–c). Further, HAADF-STEM (Fig. 3d) and elemental mapping (Fig. 3e–j) confirmed that Co-nanoparticles are dispersed uniformly on N-doped carbon sheets. The existence of a small quantity of Si might be resulted from silica precursor, which was used during catalyst preparation.
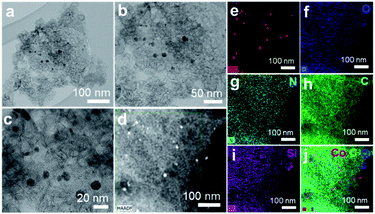 |
| Fig. 3 a–c) TEM images of Co@NC-800-L1, (d) HAADF-STEM image, corresponding element maps showing the distribution of (e) cobalt (red), (f) oxygen (blue), (g) nitrogen (cyan), (h) carbon (green), (i) silica (purple) and (j) cobalt/carbon/oxygen together (red/green/blue). | |
According to TEM images of the recycled catalyst, nanoparticle size and shape of cobalt species remained intact inside the framework (Fig. S4†), while images of the less active sample (Co-particles-800; prepared without ligand) displayed the cobalt species anchored and distributed over the remaining silica surface (Fig. S5†).
The chemical composition at the surface of the most active catalyst, Co@NC-800-L1 was investigated by X-ray photoelectron spectroscopy. In the C 1s spectra, five peaks are observed (Fig. 4a). Two peaks at ∼284.8, and ∼285.76 eV are assigned for the sp2-hybridized graphite-like carbon and the sp3-hybridized carbon. The other three peaks at ∼286.71, ∼287.94 and 289.18 eV are associated to the surface oxygen groups including C–O, C
O and C
O–C, respectively.42
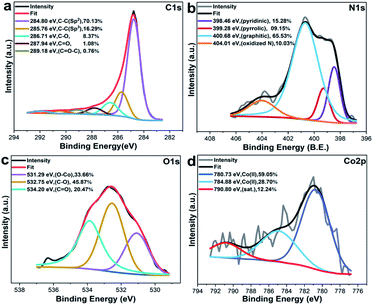 |
| Fig. 4 High resolution X-ray photoelectron spectra (HR-XPS) for Co@NC-800-L1. (a) C 1s, (b) N 1s, (c) O 1s, and (d) Co 2p region. | |
The N 1s spectra (Fig. 4b) displayed four peaks fitted with binding energies at 398.46, 399.28, 400.68 and 404.01 eV corresponding to pyridinic, pyrrolic, graphitic and oxidized N atoms, respectively.43 The O1s spectra (Fig. 4c) contributed three peaks at 531.29, 532.75 and 534.20 eV, which correspond to Co–O bonds, C–O, and C
O bonds.44 The binding energy at 532.75 eV is usually reported for partially hydrated oxides groups (such as –OH and –COOH) bonded with carbon.45 In the cobalt region, Co 2p3/2 peaks located at 780.73 eV (50.05%) and 784.88 eV (28.70%) are attributed to Co(II) species46 with 790.80 eV satellite peak (Fig. 4d). In the recycled catalyst, Co@NC-800-L1, identical cobalt species have been observed with similar binding energies and oxidation state like in the case of the fresh catalyst demonstrating the catalyst is highly stable (Fig. S6†).
Stability, recycling, and reusability of the Co@NC-800-L1 catalyst
For any given heterogeneous catalyst its stability and reusability are important aspects to allow for cost-efficient applications. To prove these features, we performed recycling experiments for the model reaction under two different reaction conditions (24 h, complete conversion and 5 h, around 60% conversion). As shown in Fig. 5, Co@NC-800-L1 exhibited high stability and was recycled and reused up to 7 times without significant deactivation.
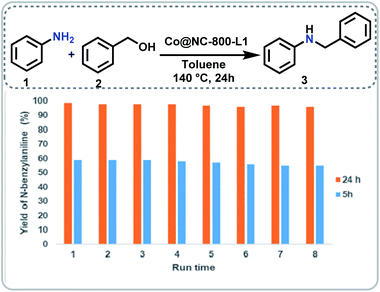 |
| Fig. 5 Stability and recycling of Co@NC-800-L1 catalyst for the synthesis of N-benzylaniline. Reaction conditions: 1 mmol aniline, 2 mmol benzyl alcohol, 30 mg catalyst (0.86 mol% Co), 1 mmol t-BuOK, 3 mL toluene, 140 °C, 24 h and 5 h. Conversions and yields are based on aniline and determined by GC using n-hexadecane standard. | |
N-Alkylation of aniline with different alcohols
With an optimal catalyst (Co@NC-800-L1) in hand, we explored its general applicability for the synthesis of primary, secondary, and tertiary amines including N-methylated products. First, we investigated the scope of alcohols for the N-alkylation of aniline. As shown in Scheme 2, various benzylic, heterocyclic, and aliphatic primary alcohols as well as secondary alcohols reacted well with aniline and gave the corresponding N-benzyl anilines in up to 87% yield (Scheme 2; products 5–14). Ether-, thioether-, hydroxy-, nitrile-, nitro-, halide (F, Cl), and silyl-containing benzyl alcohols reacted smoothly and provided a variety of functionalized secondary amines (Scheme 2; products 15–23). Next, different heterocyclic alcohols such as nicotinyl, furfuryl, and piperonyl alcohol, as well as 2-thiophenemethanol, tetrahydroquinoline-, benzodioxine-, and morpholine-based alcohols were coupled with aniline to provide the corresponding heterocyclic secondary amines in up to 89% yield (Scheme 2; products 24–31). Notably, diamination of 2,6-pyridinedimethanol proceeded well and both alcohol groups reacted to give 87% of product 32. Compared to benzylic alcohols, aliphatic alcohols are less reactive and thus more difficult to aminate. Nevertheless, our catalyst allowed for amination of such substrates too, albeit at higher temperature in the presence of 2.8 mol% catalyst (Scheme 2; products 33–40). Under these conditions linear and cyclic aliphatic alcohols gave up to 87% of N-alkylated amines. In addition, allylic amines are selectively produced in up to 77% yield without significant hydrogenation of the C–C double bond. Similar selectivity is observed for perillyl alcohol, a naturally occurring monoterpene derivative (Scheme 2; product 40). Apart from primary alcohols, even more demanding reaction of secondary alcohols with aniline provided the corresponding amines in up to 84% (Scheme 2; products 41–46). Such aminations are particularly challenging because the reduction of corresponding imine is more difficult. As an example, the amination of estrone, an important steroid derivative, gave the desired product in 60% yield (Scheme 2; product 47).
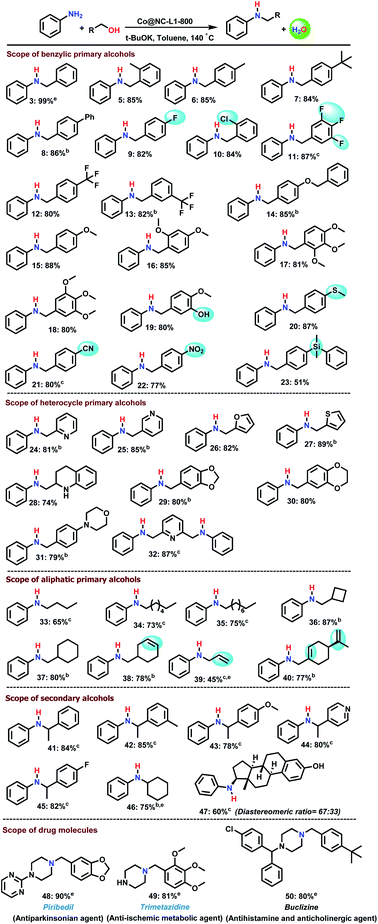 |
| Scheme 2 Co-nanoparticles catalyzed N-alkylation of aniline with different alcoholsa. a Reaction conditions: 0.5 mmol aniline, 1 mmol alcohol, 15 mg catalyst (0.86 mol% Co), 0.5 mmol t-BuOK (1 equiv.), 2 mL toluene, 140 °C, isolated yields. b same as ‘‘a’’ with 30 mg catalyst (1.7 mol% Co). c same as [a] with 50 mg catalyst (2.8 mol% Co) at 160 °C, d same as ‘‘c’’ at 150 °C. e GC yields. | |
Finally, the usefulness of this Co-based borrowing hydrogen methodology is showcased for the preparation of selected drug molecules, such as Piribedil, Trimetazidine, and Buclizine (Scheme 2, products 48–50). To prepare these molecules, piperazine and N-substituted piperazines were reacted with piperonyl alcohol, 2,3,4-trimethoxybenzyl alcohol and 4-tert-butyl benzyl alcohol under previously optimized conditions. In general, these and related drug molecules are prepared by reductive amination reactions using specific catalysts in presence of molecular hydrogen or sodium borohydride. In addition, certain products have been synthesized utilizing nucleophilic substitution reactions of corresponding amines and halogenated compounds. Advantageously, the here presented Co-catalysed BH-methodology does not require additional reducing agents and not produces any amount of halide waste.
N-Alkylation of different amines with benzylic alcohols: scope of amines
Next, we explored the scope of amines and a series of substituted and functionalized N-benzylanilines was prepared including sterically hindered derivatives (Scheme 3, products 51–55). In addition, F−, Cl−, Br-, and CF3−substituted as well as methoxy-, hydroxy-, and ester-containing substrates provided the corresponding products in up to 86% yield (Scheme 3; products 56–66). Likewise, benzylic, araliphatic and aliphatic primary amines reacted well to give N-alkylated products in up to 82% (Scheme 3, products 71–81). With respect to secondary amines, tetrahydroquinoline- and piperazine-based amines were N-benzylated smoothly to tertiary amines (Scheme 3, products 82 and 83).
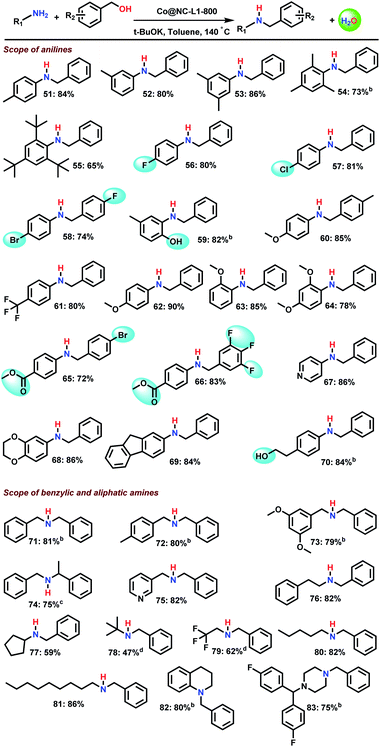 |
| Scheme 3
N-Alkylation of different amines with benzyl alcohol using Co-nanoparticlesa. a Reaction conditions: 0.5 mmol amine, 1 mmol alcohol, 0.5 mmol t-BuOK (1 equiv.), 2 mL toluene, 140 °C, 24 h, isolated yields. b same as ‘‘a’’ with 30 mg catalyst (1.7 mol% Co). c same as ‘‘a’’ with 50 mg catalyst (2.8 mol% Co) at 160 °C. d GC yields. | |
N-Methylation of amines using methanol
A special class of N-alkylamines are N-methylated products, which play a vital role as biologically active and pharmaceutically relevant compounds. Compared to traditional reagents such as methyl iodide and dimethyl sulfate, methanol constitutes a more benign methylation source, which is abundant, inexpensive and produces water as the only by-product. As shown in Scheme 4, Co@NC-800-L1 catalyst is also active and highly selective towards mono-N-methylation.
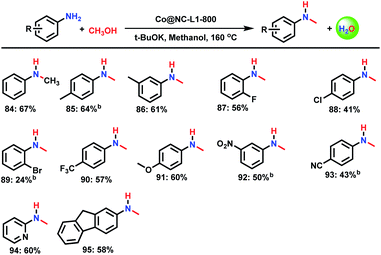 |
| Scheme 4 Co-catalysed synthesis of N-methylamines using methanola. a Reaction conditions: 0.5 mmol amine, 60 mg catalyst (3.5 mol% Co), 2 mL methanol, 1 mmol t-BuOK, 160 °C, 24 h, isolated yields. b GC yields. | |
Synthesis of primary amines from alcohols and ammonia
The successful synthesis of secondary and tertiary amines inspired us to test the applicability of our Co-catalyst for the amination of alcohols with ammonia to obtain primary amines. In general, this reaction is difficult and often secondary or tertiary amines or mixtures are formed due to the higher reactivity of the in situ-formed primary amines compared to ammonia. Nevertheless, Co@NC-800-L1 catalysed the synthesis of primary amines using gaseous ammonia (Scheme 5). More specifically, 13 simple, halogenated, and functionalized benzylic as well as heterocyclic primary amines were prepared in up to 70% yield.
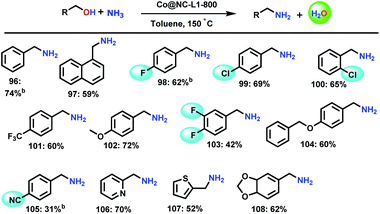 |
| Scheme 5 Co-nanoparticles catalysed synthesis of primary amines from alcohols and ammoniaa. a Reaction conditions: 0.5 mmol alcohol, 5–7 bar NH3, 60 mg catalyst (3.5 mol% Co), 0.5 mmol t-BuOK, 2 mL toluene, 24 h, isolated yields. b GC yields. | |
Conclusions
Here, we present a novel heterogeneous catalyst based on stable and reusable cobalt nanoparticles supported on N-doped carbon for the general and selective synthesis of amines from alcohols by borrowing hydrogen methodology. The optimal catalytic material is prepared by the pyrolysis of a Co–nitrogen complex-SiO2 templated material, which is generated by mixing cobalt nitrate, 1,10-phenanthroline, and colloidal silica, and subsequent removal of the later. Applying these nanoparticles many functionalized and structurally diverse primary, secondary, and tertiary amines including N-methylamines and selected drug molecules can be conveniently prepared starting from inexpensive and easily available alcohols and amines or ammonia.
Data availability
All experimental data associated with this work is available in the ESI.†
Author contributions
RVJ and MB supervised the project. ZM, RVJ and MB planned and developed the project, and designed the experiments. ZM prepared and developed catalysts and performed all catalytic experiments. BZ and XL, co-performed catalytic experiments and reproduced the results. RGM, MBG, MP and RZ performed catalysts characterization. RVJ, ZM and MB wrote the paper. RGM, MBG, and RZ co-wrote the paper.
Conflicts of interest
There are no conflicts to declare.
Acknowledgements
We gratefully acknowledge the European Research Council (EU project 670986-NoNaCat), Deutsche Forschungsgemeinschaft (DFG; Project 447724917) and the State of Mecklenburg-Vorpommern for financial and general support. We thank the analytical team of the Leibniz-Institut für Katalyse e.V. for their excellent service. The authors from Palacky University gratefully acknowledge the support by the Operational Program Research, Development and Education – European Regional Development Fund (project no. CZ.02.1.01/0.0/0.0/16_019/0000754) and by the ERDF project “Development of pre-applied research in nanotechnology and biotechnology” (project no. CZ.02.1.01/0.0/0.0/17_048/0007323) of the Ministry of Education, Youth and Sports of the Czech Republic. RZ acknowledges the support from the Czech Science Foundation, project No. 19-27454X. We gratefully acknowledge Ondřej Tomanec from Palacky University Olomouc for HRTEM measurements. Zhuang Ma and Xinmin Li thank the Chinese Scholarship Council (CSC) for financial support.
Notes and references
- J. Bariwal and E. Van der Eycken, Chem. Soc. Rev., 2013, 42, 9283–9303 RSC.
- P. Ruiz-Castillo and S. L. Buchwald, Chem. Rev., 2016, 116, 12564–12649 CrossRef CAS.
- T. Irrgang and R. Kempe, Chem. Rev., 2020, 120, 9583–9674 CrossRef CAS.
- K. Murugesan, T. Senthamarai, V. G. Chandrashekhar, K. Natte, P. C. Kamer, M. Beller and R. V. Jagadeesh, Chem. Soc. Rev., 2020, 49, 6273–6328 RSC.
- O. I. Afanasyev, E. Kuchuk, D. L. Usanov and D. Chusov, Chem. Rev., 2019, 119, 11857–11911 CrossRef CAS.
- T. E. Muller, K. C. Hultzsch, M. Yus, F. Foubelo and M. Tada, Chem. Rev., 2008, 108, 3795–3892 CrossRef PubMed.
- A. Corma, J. Navas and M. J. Sabater, Chem. Rev., 2018, 118, 1410–1459 CrossRef CAS.
- T. Irrgang and R. Kempe, Chem. Rev., 2018, 119, 2524–2549 CrossRef.
- B. G. Reed-Berendt, D. E. Latham, M. B. Dambatta and L. C. Morrill, ACS Cent. Sci., 2021, 7, 570–585 CrossRef CAS PubMed.
- G. Guillena, D. J. Ramon and M. Yus, Chem. Rev., 2010, 110, 1611–1641 CrossRef CAS PubMed.
-
F. Shi and X. Cui, Catalytic amination for N-alkyl amine synthesis, Elsevier., 2018 Search PubMed.
-
S. A. Lawrence, Amines: synthesis, properties and applications, Cambridge University Press, 2004 Search PubMed.
-
A. Ricci, Amino group chemistry: from synthesis to the life sciences, John Wiley & Sons., 2008 Search PubMed.
-
https://njardarson.lab.arizona.edu/sites/njardarson.lab.arizona.edu/files/Top%20200%20Pharmaceuticals%20By%20Retail%20Sales%202020V3.pdfTop 200 Pharmaceuticals by Retail Sales in 2020.
-
(a) D. Pingen, C. Müller and D. Vogt, Angew. Chem., Int. Ed., 2010, 49, 8130–8133 CrossRef CAS PubMed;
(b) M. H. S. Hamid, C. L. Allen, G. W. Lamb, A. C. Maxwell, H. C. Maytum, A. J. Watson and J. M. Williams, J. Am. Chem. Soc., 2009, 131, 1766–1774 CrossRef CAS;
(c) T. T. Dang, B. Ramalingam, S. P. Shan and A. M. Seayad, ACS Catal., 2013, 3, 2536–2540 CrossRef CAS;
(d) J. J. A. Celaje, X. Zhang, F. Zhang, L. Kam, J. R. Herron and T. J. Williams, ACS Catal., 2017, 7, 1136–1142 CrossRef CAS;
(e) B. Emayavaramban, P. Chakraborty, E. Manoury, R. Poli and B. Sundararaju, Org. Chem. Front., 2019, 6, 852–857 RSC;
(f) C. Gunanathan and D. Milstein, Angew. Chem., 2008, 120, 8789–8792 CrossRef.
- T. Yan, B. L. Feringa and K. Barta, Nat. Commun., 2014, 5, 1–7 Search PubMed.
-
(a) S. Elangovan, J. Neumann, J.-B. Sortais, K. Junge, C. Darcel and M. Beller, Nat. Commun., 2016, 7, 1–8 Search PubMed;
(b) N. Deibl and R. Kempe, Angew. Chem., Int. Ed., 2017, 56, 1663–1666 CrossRef CAS PubMed.
- M. Vayer, S. P. Morcillo, J. Dupont, V. Gandon and C. Bour, Angew. Chem., Int. Ed., 2018, 57, 3228–3232 CrossRef CAS.
- A. Quintard, T. Constantieux and J. Rodriguez, Angew.
Chem., Int. Ed., 2013, 52, 12883–12887 CrossRef CAS.
- N. Deibl and R. Kempe, J. Am. Chem. Soc., 2016, 138, 10786–10789 CrossRef CAS.
-
(a) M. Vellakkaran, K. Singh and D. Banerjee, ACS Catal., 2017, 7, 8152–8158 CrossRef CAS;
(b) M. Subaramanian, S. P. Midya, P. M. Ramar and E. Balaraman, Org. Lett., 2019, 21, 8899–8903 CrossRef CAS.
-
(a) A. S. Alshammari, K. Natte, N. V. Kalevaru, A. Bagabas and R. V. Jagadeesh, J. Catal., 2020, 382, 141–149 CrossRef CAS;
(b) A. Corma, T. Ródenas and M. J. Sabater, Chem.–Eur. J., 2010, 16, 254–260 CrossRef CAS;
(c) S. Rojas-Buzo, P. Concepción, A. Corma, M. Moliner and M. Boronat, ACS Catal., 2021, 11, 8049–8061 CrossRef CAS.
-
(a) K.-i. Shimizu, S. Kanno, K. Kon, S. H. Siddiki, H. Tanaka and Y. Sakata, Catal. Today, 2014, 232, 134–138 CrossRef CAS;
(b) K.-i. Shimizu, N. Imaiida, K. Kon, S. Hakim Siddiki and A. Satsuma, ACS Catal., 2013, 3, 998–1005 CrossRef CAS.
- K.-i. Shimizu, K. Kon, W. Onodera, H. Yamazaki and J. N. Kondo, ACS Catal., 2013, 3, 112–117 CrossRef CAS.
- A. Tomer, F. Wyrwalski, C. Przybylski, J.-F. Paul, E. Monflier, M. Pera-Titus and A. Ponchel, J. Catal., 2017, 356, 111–124 CrossRef CAS.
- A. Afanasenko, S. Elangovan, M. C. Stuart, G. Bonura, F. Frusteri and K. Barta, Catal. Sci. Technol., 2018, 8, 5498–5505 RSC.
- X. Cui, X. Dai, Y. Deng and F. Shi, Chem.–Eur. J., 2013, 19, 3665–3675 CrossRef CAS.
- C. Gonzalez-Arellano, K. Yoshida, R. Luque and P. L. Gai, Green Chem., 2010, 12, 1281–1287 RSC.
- F. Santoro, R. Psaro, N. Ravasio and F. Zaccheria, ChemCatChem, 2012, 4, 1249–1254 CrossRef CAS.
- M. Dixit, M. Mishra, P. A. Joshi and D. O. Shah, Catal. Commun., 2013, 33, 80–83 CrossRef CAS.
- H. Liu, G.-K. Chuah and S. Jaenicke, J. Catal., 2015, 329, 262–268 CrossRef CAS.
-
(a) Y. Wu, Y. Huang, X. Dai and F. Shi, ChemSusChem, 2019, 12, 3185–3191 CrossRef CAS PubMed;
(b) S. Pang, Y. Deng and F. Shi, ChemComm, 2015, 51, 9471–9474 RSC.
- R. V. Jagadeesh, A.-E. Surkus, H. Junge, M.-M. Pohl, J. Radnik, J. Rabeah, H. Huan, V. Schünemann, A. Brückner and M. Beller, Science, 2013, 342, 1073–1076 CrossRef CAS PubMed.
- R. V. Jagadeesh, T. Stemmler, A.-E. Surkus, M. Bauer, M.-M. Pohl, J. Radnik, K. Junge, H. Junge, A. Brückner and M. Beller, Nat. Protoc., 2015, 10, 916–926 CrossRef PubMed.
- R. V. Jagadeesh, K. Murugesan, A. S. Alshammari, H. Neumann, M.-M. Pohl, J. Radnik and M. Beller, Science, 2017, 358, 326–332 CrossRef CAS PubMed.
- G. Hahn, P. Kunnas, N. de Jonge and R. Kempe, Nat. Catal., 2019, 2, 71–77 CrossRef CAS.
- K. Shen, X. Chen, J. Chen and Y. Li, ACS Catal., 2016, 6, 5887–5903 CrossRef CAS.
- N. Yan, Y. Yuan and P. J. Dyson, Dalton Trans., 2013, 42, 13294–13304 RSC.
- F. Kallmeier, R. Fertig, T. Irrgang and R. Kempe, Angew. Chem., Int. Ed., 2020, 59, 11789–11793 CrossRef CAS PubMed.
- S. Rösler, M. Ertl, T. Irrgang and R. Kempe, Angew. Chem., Int. Ed., 2015, 54, 15046–15050 CrossRef PubMed.
- R. Liu, Y. Zhao, R. Huang, Y. Zhao and H. Zhou, CrystEngComm, 2010, 12, 4091–4094 RSC.
- H. Wu, J. Geng, H. Ge, Z. Guo, Y. Wang and G. Zheng, Adv. Energy Mater., 2016, 6, 1600794 CrossRef.
- Z. Du, X. Chen, W. Hu, C. Chuang, S. Xie, A. Hu, W. Yan, X. Kong, X. Wu and H. Ji, J. Am. Chem. Soc., 2019, 141, 3977–3985 CrossRef CAS PubMed.
- T. Choudhury, S. Saied, J. Sullivan and A. Abbot, J. Phys. D: Appl. Phys., 1989, 22, 1185–1195 CrossRef CAS.
- J. Li, S. Xiong, X. Li and Y. Qian, J. Mater. Chem., 2012, 22, 23254–23259 RSC.
- Z. Wang, S. Peng, Y. Hu, L. Li, T. Yan, G. Yang, D. Ji, M. Srinivasan, Z. Pan and S. Ramakrishna, J. Mater. Chem. A., 2017, 5, 4949–4961 RSC.
Footnote |
† Electronic supplementary information (ESI) available. See DOI: 10.1039/d1sc05913k |
|
This journal is © The Royal Society of Chemistry 2022 |
Click here to see how this site uses Cookies. View our privacy policy here.