DOI:
10.1039/D1SC05974B
(Edge Article)
Chem. Sci., 2022,
13, 7240-7246
Ultrafast and selective labeling of endogenous proteins using affinity-based benzotriazole chemistry†
Received
29th October 2021
, Accepted 27th May 2022
First published on 30th May 2022
Abstract
Chemical modification of proteins is enormously useful for characterizing protein function in complex biological systems and for drug development. Selective labeling of native or endogenous proteins is challenging owing to the existence of distinct functional groups in proteins and in living systems. Chemistry for rapid and selective labeling of proteins remains in high demand. Here we have developed novel affinity labeling probes using benzotriazole (BTA) chemistry. We showed that affinity-based BTA probes selectively and covalently label a lysine residue in the vicinity of the ligand binding site of a target protein with a reaction half-time of 28 s. The reaction rate constant is comparable to the fastest biorthogonal chemistry. This approach was used to selectively label different cytosolic and membrane proteins in vitro and in live cells. BTA chemistry could be widely useful for labeling of native/endogenous proteins, target identification and development of covalent inhibitors.
Introduction
Selective chemical labeling of proteins is highly valuable for studying and controlling protein structures, dynamics and functions in vitro and in living systems,1–9 for development of new materials10 and for drug development.11–13 As proteins carry many copies of various functional groups, it is challenging to selectively label a protein at a defined site.14 Moreover, the labeling conditions have to be mild in aqueous solution and reactions should be orthogonal to other functionalities in living systems when a protein is to be labeled in vivo. Numerous chemical tagging approaches in combination with bioorthogonal chemistry enable incorporation of chemical probes into proteins in cells and organisms.3,15–18 Although these approaches are versatile and robust, most of these approaches rely on exogenous expression of protein of interest (POI) with genetic tags or non-canonical amino acids.2,6,8,19–22 The advance in selective labeling of endogenous proteins facilitates affinity-based protein profiling,23 proteomic analysis of cellular organelles and protein complexes,24 target identification,25 and diagnosis.26
Affinity labeling of proteins based on proximity-driven chemistry has been a powerful approach for selective labeling of native or endogenous proteins,27 and for the development of covalent inhibitors.11 Following the protein–ligand interaction, the electrophilic group of the probe is placed in proximity of the POI, thereby facilitating nucleophilic attack by residues in the vicinity of the ligand-binding site, leading to covalent binding. Depending on the purpose of application, affinity labeling approaches can afford the covalent binding of ligands that eventually disrupts protein function or locks the protein at a defined state. Alternatively, these approaches can label native or endogenous proteins without functional disruption owing to the concomitant release of ligands upon labeling. In the latter, ligand-directed probes that consist of a ligand for the POI, an electrophilic leaving group and a tag have been used for covalent attachment of a desired tag to the POI. The operation is very facile without genetic manipulation.
A range of reactive groups have been developed and used in affinity labeling of proteins, including classical electrophiles such as α-halocarbonyls, epoxides, acrylamides, vinyl sulfonates, etc. (Fig. 1a), which have been used for covalent binding of ligands/inhibitors.28–32 The emerging ligand-directed chemistry has been used to label endogenous proteins in living systems, including tosyl (LDT),33–35 alkyloxyacyl imidazole (LDAI),36–38O-nitrobenzoxadiazole (O-NBD),39 dibromophenylbenzoate (LDBB),40 difluorophenylbenzoate,41N-sulfonyl pyridone,42 and N-acyl-N-alkyl sulfonamide (LDNASA) chemistry (Fig. 1a).43 These reactive groups are relatively less reactive towards nucleophiles under certain conditions, while the reaction is accelerated by proximity effect to achieve selectivity. However, most of the affinity-labeling reagents suffer from sluggish reaction rates, which require hours to a day to yield sufficiently labeled proteins. Recently, the LDNASA strategy achieves the most rapid reaction rate with a second-order rate constant in the range of 104 M−1 s−1.43 Therefore, new reactive groups for affinity labeling with fast reaction rates and good selectivity remain in high demand. Herein, we report a benzotriazole (BTA) chemistry that can be incorporated into affinity labeling probes for rapid and selective labeling of native or endogenous proteins in vitro and in cells.
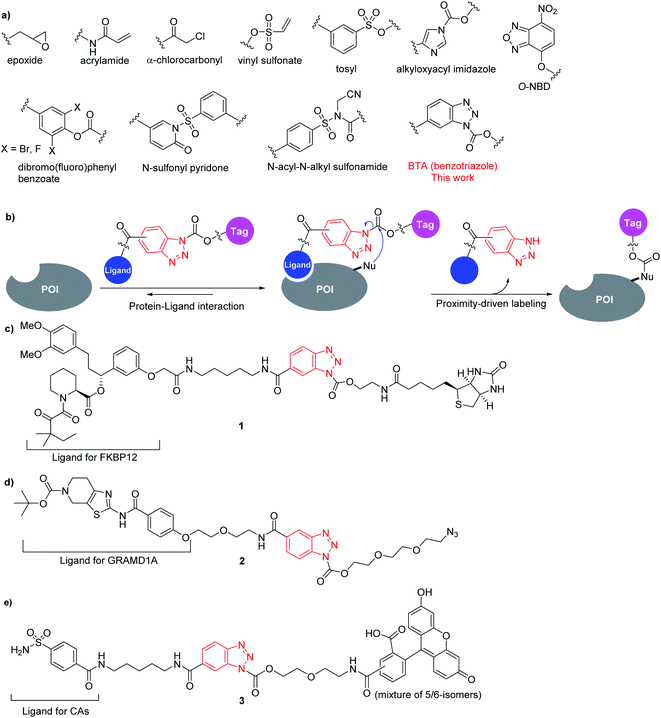 |
| Fig. 1 (a) Reactive groups used in affinity labeling. (b) Schematic illustration of affinity labeling using BTA chemistry for selective protein modification. (c–e) Compounds used for labeling FKBP12 (c), GRAMD1A (d) and carbonic anhydrases (CAs) (e) in this study. | |
Results and discussion
Benzotriazole contains two electron-deficient sp2 nitrogen atoms (N2 and N3) and the pKa of the N1–H is ca. 8. It has been used as an efficient synthetic auxiliary group for the N-substitution of various compounds in organic synthesis, because the benzotriazolate anion is a good leaving group and N-substituted derivatives are easy to prepare.44,45 Moreover, ligands or chemical handles could be placed on the phenyl ring for a design of affinity labeling probes (Fig. 1b).
As proof of principle, we designed compound 1, in which a synthetic ligand for the FKBP12 protein (SLF)46 is linked to a biotin tag via the benzotriazole moiety and linkers (Fig. 1c). The covalent conjugation of 1 with the recombinant FKBP12 protein was evaluated by denaturing SDS-PAGE and monitored by western blot of biotin. Reaction of 5 μM FKBP12 with 10 μM compound 1 proceeded rapidly in aqueous solution with a half-time of 113 s (Fig. 2a and b). According to the equation of observed rate constant (kobs), under the conditions used (the concentration of reagent is much higher than Kd of ligand binding), the observed ultrafast reaction rate reflects the reactivity of the BTA chemistry driven by proximity effect (kchem) (ESI†). Addition of parental ligand SLF led to abolishment of the conjugation, suggesting that the BTA reaction is selectively driven by a proximity effect mediated by ligand binding (Fig. 2a). In order to compare with the reaction rate of LDNASA chemistry, we prepared the same LDNASA probe that was reported to afford the highest rate constant in affinity-labeling reagents and measured the kinetics for labeling of the FKBP12 protein (Fig. S1†).43 Under the same conditions, the observed half-time of the labeling reaction by LDNASA was determined to be 151 s. Therefore, the affinity labeling of FKBP12 by BTA chemistry is ca. 1.3-fold faster than LDNASA chemistry.
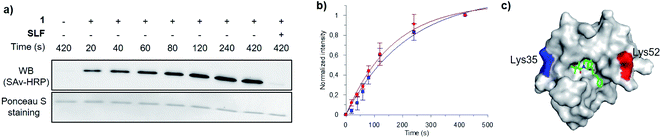 |
| Fig. 2
In vitro labeling of FKBP12 protein. (a) SDS-PAGE and western blotting analysis of labeling reaction of 5 μM FKBP12 with 10 μM 1. (b) Kinetics of labeling reactions of 5 μM FKBP12 using 10 μM 1 (red) vs. LDNASA (blue). The solid line shows the fitting to a single-exponential equation for determining t1/2. Error bars represent standard deviation of three independent experiments. (c) The crystal structure of the FKBP12-SLF complex (PDB ID: 1FKG). The labelling sites of 1 are marked as red (Lys52, the major labeling site) and blue (Lys35, the minor labelling site). SLF is shown in green sticks. | |
The stability of the BTA probe 1 in DMSO and in aqueous buffer was measured by HPLC. The half-life of 1 was determined to be 3.7 h in neutral buffer at 37 °C. 63% of 1 remained intact after 48 h in DMSO at 37 °C, while no detectable degradation of 1 was observed in DMSO at −20 °C for more than 10 months (Fig. S2†). Therefore, the BTA probe is stable enough for labeling reactions. Reaction of 1 with FKBP12 led to conjugation of one or two biotin groups to the protein (Fig. S3†). A peptide mapping analysis was carried out to determine the labeling site(s) on FKBP12. After reaction with 1, FKBP12 was digested with trypsin and subjected to LC-MS/MS analysis (ESI†). The results showed that Lys52 (ca. 11 Å away from SLF) is the major labeling site and Lys35 (ca. 17 Å away from SLF) is the minor labeling site (Fig. 2c and S4†). Both sites are in the vicinity of the SLF-binding site on FKBP12 (Fig. 2c). The results showed that the BTA probe undergoes nucleophilic attack by the ε-amino group of lysine side chains to attach a biotin group to the protein with concomitant release of the ligand.
To examine the feasibility of using BTA chemistry in biological contexts, we labeled FKBP12 with 1 in cell lysates. HeLa cell lysate (total protein concentration of 1.5 mg mL−1) was mixed with recombinant FKBP12 (2 μM) and 1 (up to 10 μM) at 37 °C. The labeling reaction was completely abolished by SLF (Fig. 3a). Non-specific labelling was not observed with up to 10 μM 1 at reaction times of up to 2 h (Fig. S5†). To demonstrate labeling of endogenous FKBP12 in live cells, we incubated HeLa cells with 1 μM 1 and subjected the labeled cell lysates to immunoprecipitation using streptavidin beads. We observed specific pull-down of endogenous FKBP12, which was abolished by SLF (Fig. 3b). These results suggest that BTA chemistry is biorthogonal and can be used for selective labeling of endogenous proteins in biological contexts.
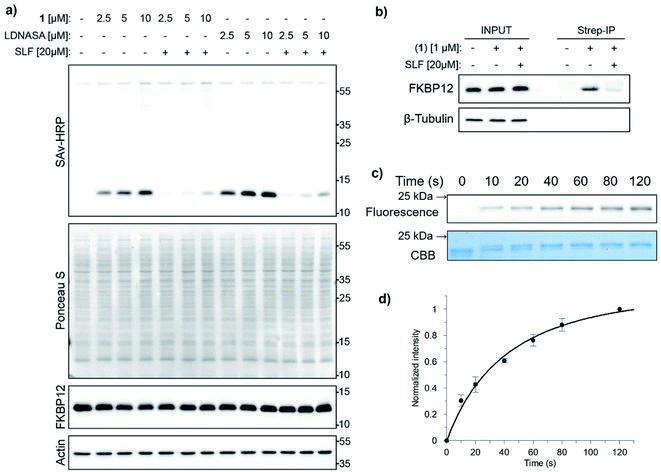 |
| Fig. 3 (a) SDS-PAGE and western blotting analysis of FKBP12 labeling with 1 or LDNASA (2.5–10 μM) for 5 min in HeLa cell lysates supplemented with 2 μM recombinant FKBP12. (b) HeLa cells labeled with 1 were subjected to immunoprecipitation using streptavidin beads. Endogenous FKBP12 was visualized using an antibody against FKBP12. Data are representative of three independent experiments. (c) SDS-PAGE and in-gel fluorescence analysis of the labeling of 5 μM GRAMD1A (StART domain) with 10 μM 2. CBB: Coomassie brilliant blue staining. (d) Kinetics of the labeling reaction shown in (c). The solid line shows the fitting to an equation for determining the rate constant of second-order reaction (ESI†). Error bars represent standard deviation of three independent experiments. | |
Prompted by the results of 1, we used BTA chemistry to label a new protein. Autophagy is an evolutionarily conserved intracellular degradation system through which cytoplasmic constituents are sequestered in double-membrane autophagosomes and delivered to lysosomes for clearance. Recently, we reported novel autophagy inhibitors, autogramins, and identified their cellular target GRAMD1A, a cholesterol-transfer protein localizing at the endoplasmic reticulum (ER) membrane contact sites. Autogramins compete with cholesterol binding to the StART domain of GRAMD1A, thereby inhibiting its cholesterol transfer activity that is required for autophagosome biogenesis.47,48 GRAMD1A also plays an important role in cholesterol homeostasis at ER-plasma membrane contact sites.49,50 We prepared compound 2 containing autogramin-2, the BTA moiety and azide as a biorthogonal handle (Fig. 1d). Recombinant StART domain of GRAMD1A protein was reacted with 2. Reaction of 2 with GRAMD1A led to conjugation of a single azide group to the protein (Fig. S6†). The labeling was detected by incorporation of the DBCO-TAMRA fluorescent dye into the protein via strain-promoted azide–alkyne cycloaddition as well as by the shift of mobility of the labeled protein in SDS-PAGE (Fig. 3c). Reaction of 5 μM GRAMD1A with 10 μM compound 2 proceeded rapidly in aqueous solution with a half-time of 28 s. The second-order reaction rate constant of BTA probe 2 was determined to be (2.7 ± 0.4) × 103 M−1 s−1 (Fig. 3d, ESI†). A peptide mapping analysis using LC-MS/MS showed that Lys158 (ca. 11.6 Å away from autogramin-2) is the major labeling site (Fig. S7†), suggesting that the BTA probe undergoes nucleophilic attack by the ε-amino group of lysine side chain in the vicinity of the ligand-binding site to attach an azide group to the protein with concomitant release of the ligand.
To demonstrate the application of BTA chemistry to labeling and imaging of endogenous proteins in live cells, we prepared compound 3 that consists of arylsulfonamide as a ligand for carbonic anhydrases (CAs), BTA and fluorescein as a fluorescent reporter. Carbonic anhydrases are a family of enzymes that catalyze the interconversion between carbon dioxide and carbonic acid. CA and its inhibitor arylsulfonamides have been serving as an important model for studies of protein–ligand interaction.51 The cell-surface transmembrane CAs (CA9 and CA12) are overexpressed in many tumors and appear to be regulated by microenvironmental hypoxia. They contribute to the tumor microenvironmental acidity and tumor malignant phenotype, making them a valuable diagnostic and prognostic biomarker for cancer.52–54 CA12 is overexpressed in breast cancer cells and is transcriptionally regulated by estrogen receptor α (ERα).55 To label the cell surface membrane protein, probe 3 was prepared with a fluorescein dye containing carboxylic acid to make it less cell-permeable. Confocal microscopy showed that probe 3 quickly labels the plasma membrane of the breast cancer cell line MCF-7. The labeling was abolished by the inhibitor of CAs, ethoxzolamide (EZA) (Fig. 4a and b). To confirm the specificity of the labeling, MCF-7 cells labeled with 3 were lysed and subjected to denaturing SDS-PAGE. Selective covalent labeling of CA12 was confirmed by western blot and immunoprecipitation (Fig. 4c and d). The observed multiple bands of CA12 correlate with its glycosylation in cells.56 Immunoprecipitation using the anti-fluorescein isothiocyanate (FITC) antibody led to specific pull-down of CA12, which was abolished by EZA (Fig. 4d). These results demonstrated that BTA chemistry can be used to selectively label endogenous proteins in live cells.
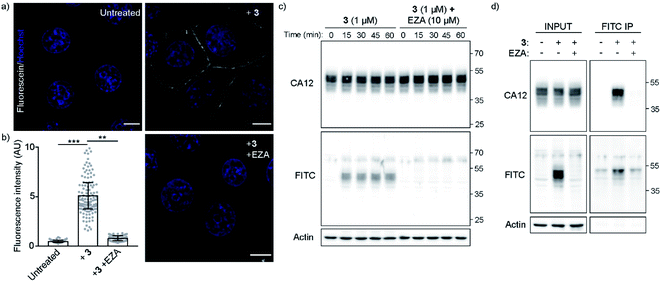 |
| Fig. 4 Labeling of endogenous CA12 using 3. (a) Representative confocal fluorescent images of MCF7 cells treated with 3 in the absence and the presence of EZA. Nuclei were counterstained with Hoechst 33342. Scale bars, 10 μm. (b) Quantification of fluorescein fluorescence intensity from (a). Data were shown as mean ± sd from three biologically independent experiments. Data points represent individual cells pooled from the three independent experiments (n > 100 cells). Significance was determined from biological replicates using a one-way ANOVA with Tukey's multiple comparisons tests. **p = 0.0014, ***p = 0.0009. (c) Western blot analysis of MCF7 cells treated with 3 in the absence and the presence of EZA. (d) MCF7 cells labeled with 3 were subjected to immunoprecipitation using the anti-FITC antibody. CA12 and the fluorescein-labeled protein were visualized using antibodies against CA12 and FITC, respectively. Data are representative of three independent experiments. | |
In summary, we have developed BTA chemistry, which shows high selectivity, bioorthogonality and rapid reaction kinetics for labeling of endogenous proteins. The reaction rate of BTA chemistry was faster than LDNASA chemistry, and was comparable to the fastest biorthogonal reactions. The modular design of BTA probes makes it facile to label various cytosolic and membrane proteins with different tags. We envision that BTA chemistry could be an invaluable tool for labeling of native/endogenous proteins, target identification and development of covalent inhibitors.
Author contributions
Y. W. W. supervised the project. X. X., Y. Z. and D. P. C. carried out the research. M. G., S. L. L., and R. A. Z. carried out and analyzed the peptide mapping experiments of GRAMD1A. Y. Zhou analyzed the data of peptide mapping of FKBP12. X. X., Y. Z., D. P. C. and Y. W. W. wrote the paper.
Conflicts of interest
There are no conflicts to declare.
Acknowledgements
This work was supported by the European Research Council (ChemBioAP), Vetenskapsrådet (No. 2018-04585), the Knut and Alice Wallenberg Foundation and Göran Gustafsson Foundation for Research in Natural Sciences to Y. W. W. D. P. C. was supported by a fellowship from the Canadian Institute of Health Research (MFE-152550). We acknowledge the Biochemical Imaging Center (BICU) at Umeå University and the National Microscopy Infrastructure, NMI (VR-RFI 2016-00968) for providing assistance in microscopy. We thank Alexander Manoilov and Jonas Bergquist at the Mass Spectrometry Based Proteomics Facility, Uppsala University for performing the LC-MS/MS experiment. We acknowledge the Chemical Proteomics facility at Biomedicum, Karolinska Institutet, part of both SciLifeLab and the Swedish National Infrastructure for Biological Mass Spectrometry (BioMS).
References
- P. E. Dawson and S. B. Kent, Synthesis of native proteins by chemical ligation, Annu. Rev. Biochem., 2000, 69, 923–960 CrossRef CAS PubMed.
- B. N. Giepmans, S. R. Adams, M. H. Ellisman and R. Y. Tsien, The fluorescent toolbox for assessing protein location and function, Science, 2006, 312(5771), 217–224 CrossRef CAS PubMed.
- E. M. Sletten and C. R. Bertozzi, Bioorthogonal chemistry: fishing for selectivity in a sea of functionality, Angew. Chem., Int. Ed., 2009, 48(38), 6974–6998 CrossRef CAS PubMed.
- C. P. R. Hackenberger and D. Schwarzer, Chemoselective Ligation and Modification Strategies for Peptides and Proteins, Angew. Chem., Int. Ed., 2008, 47(52), 10030–10074 CrossRef CAS PubMed.
- M. Vila-Perello and T. W. Muir, Biological Applications of Protein Splicing, Cell, 2010, 143(2), 191–200 CrossRef CAS PubMed.
- K. Lang and J. W. Chin, Cellular incorporation of unnatural amino acids and bioorthogonal labeling of proteins, Chem. Rev., 2014, 114(9), 4764–4806 CrossRef CAS PubMed.
- Y. Takaoka, A. Ojida and I. Hamachi, Protein organic chemistry and applications for labeling and engineering in live-cell systems, Angew. Chem., Int. Ed., 2013, 52(15), 4088–4106 CrossRef CAS PubMed.
- X. Chen and Y. W. Wu, Selective chemical labeling of proteins, Org. Biomol. Chem., 2016, 14(24), 5417–5439 RSC.
- L. Xu, S. L. Kuan and T. Weil, Contemporary Approaches for Site-Selective Dual Functionalization of Proteins, Angew. Chem., Int. Ed. Engl., 2021, 60, 13757–13777 CrossRef CAS PubMed.
- L. S. Witus and M. B. Francis, Using synthetically modified proteins to make new materials, Acc. Chem. Res., 2011, 44(9), 774–783 CrossRef CAS PubMed.
- J. Singh, R. C. Petter, T. A. Baillie and A. Whitty, The resurgence of covalent drugs, Nat. Rev. Drug Discovery, 2011, 10(4), 307–317 CrossRef CAS PubMed.
- N. Krall, F. P. da Cruz, O. Boutureira and G. J. Bernardes, Site-selective protein-modification chemistry for basic biology and drug development, Nat. Chem., 2016, 8(2), 103–113 CrossRef CAS PubMed.
- Q. Y. Hu, F. Berti and R. Adamo, Towards the next generation of biomedicines by site-selective conjugation, Chem. Soc. Rev., 2016, 45(6), 1691–1719 RSC.
- C. Zhang, E. V. Vinogradova, A. M. Spokoyny, S. L. Buchwald and B. L. Pentelute, Arylation Chemistry for Bioconjugation, Angew. Chem., Int. Ed. Engl., 2019, 58(15), 4810–4839 CrossRef CAS PubMed.
- C. P. Ramil and Q. Lin, Bioorthogonal chemistry: strategies and recent developments, Chem. Commun., 2013, 49(94), 11007–11022 RSC.
- C. D. Spicer and B. G. Davis, Selective chemical protein modification, Nat. Commun., 2014, 5, 4740 CrossRef CAS PubMed.
- D. M. Patterson, L. A. Nazarova and J. A. Prescher, Finding the right (bioorthogonal) chemistry, ACS Chem. Biol., 2014, 9(3), 592–605 CrossRef CAS PubMed.
- M. Yang, J. Li and P. R. Chen, Transition metal-mediated bioorthogonal protein chemistry in living cells, Chem. Soc. Rev., 2014, 43(18), 6511–6526 RSC.
- H. M. O'Hare, K. Johnsson and A. Gautier, Chemical probes shed light on protein function, Curr. Opin. Struct. Biol., 2007, 17(4), 488–494 CrossRef PubMed.
- C. R. Jing and V. W. Cornish, Chemical Tags for Labeling Proteins Inside Living Cells, Acc. Chem. Res., 2011, 44(9), 784–792 CrossRef CAS PubMed.
- Y. Zhang, K. Y. Park, K. F. Suazo and M. D. Distefano, Recent progress in enzymatic protein labelling techniques and their applications, Chem. Soc. Rev., 2018, 47(24), 9106–9136 RSC.
- Y. W. Wu and R. S. Goody, Probing protein function by chemical modification, J. Pept. Sci., 2010, 16(10), 514–523 CrossRef CAS PubMed.
- M. J. Evans and B. F. Cravatt, Mechanism-based profiling of enzyme families, Chem. Rev., 2006, 106(8), 3279–3301 CrossRef CAS PubMed.
- W. Qin, K. F. Cho, P. E. Cavanagh and A. Y. Ting, Deciphering molecular interactions by proximity labeling, Nature Methods, 2021, 18(2), 133–143 CrossRef CAS PubMed.
- S. Ziegler, V. Pries, C. Hedberg and H. Waldmann, Target identification for small bioactive molecules: finding the needle in the haystack, Angew. Chem., Int. Ed. Engl., 2013, 52(10), 2744–2792 CrossRef CAS PubMed.
- N. K. Devaraj and R. Weissleder, Biomedical applications of tetrazine cycloadditions, Acc. Chem. Res., 2011, 44(9), 816–827 CrossRef CAS PubMed.
- T. Tamura and I. Hamachi, Chemistry for Covalent Modification of Endogenous/Native Proteins: From Test Tubes to Complex Biological Systems, J. Am. Chem. Soc., 2019, 141(7), 2782–2799 CrossRef CAS PubMed.
- J. M. Ostrem, U. Peters, M. L. Sos, J. A. Wells and K. M. Shokat, K-Ras(G12C) inhibitors allosterically control GTP affinity and effector interactions, Nature, 2013, 503(7477), 548–551 CrossRef CAS PubMed.
- W. Liu, F. Li, X. Chen, J. Hou, L. Yi and Y. W. Wu, A rapid and fluorogenic TMP-AcBOPDIPY probe for covalent labeling of proteins in live cells, J. Am. Chem. Soc., 2014, 136(12), 4468–4471 CrossRef CAS PubMed.
- D. Wiegandt, S. Vieweg, F. Hofmann, D. Koch, F. Li, Y. W. Wu, A. Itzen, M. P. Muller and R. S. Goody, Locking GTPases covalently in their functional states, Nat. Commun., 2015, 6, 7773 CrossRef CAS PubMed.
- S. R. Adusumalli, D. G. Rawale, U. Singh, P. Tripathi, R. Paul, N. Kalra, R. K. Mishra, S. Shukla and V. Rai, Single-Site Labeling of Native Proteins Enabled by a Chemoselective and Site-Selective Chemical Technology, J. Am. Chem. Soc., 2018, 140(44), 15114–15123 CrossRef CAS PubMed.
- B. Gulen, M. Rosselin, J. Fauser, M. F. Albers, C. Pett, C. Krisp, V. Pogenberg, H. Schluter, C. Hedberg and A. Itzen, Identification of targets of AMPylating Fic enzymes by co-substrate-mediated covalent capture, Nat. Chem., 2020, 12(8), 732–739 CrossRef CAS PubMed.
- T. Tamura, Y. Kioi, T. Miki, S. Tsukiji and I. Hamachi, Fluorophore labeling of native FKBP12 by ligand-directed tosyl chemistry allows detection of its molecular interactions in vitro and in living cells, J. Am. Chem. Soc., 2013, 135(18), 6782–6785 CrossRef CAS PubMed.
- T. Tamura, S. Tsukiji and I. Hamachi, Native FKBP12 engineering by ligand-directed tosyl chemistry: labeling properties and application to photo-cross-linking of protein complexes in vitro and in living cells, J. Am. Chem. Soc., 2012, 134(4), 2216–2226 CrossRef CAS PubMed.
- S. Tsukiji, M. Miyagawa, Y. Takaoka, T. Tamura and I. Hamachi, Ligand-directed tosyl chemistry for protein labeling in vivo, Nat. Chem. Biol., 2009, 5(5), 341–343 CrossRef CAS PubMed.
- K. Yamaura, S. Kiyonaka, T. Numata, R. Inoue and I. Hamachi, Discovery of allosteric modulators for GABAA receptors by ligand-directed chemistry, Nat. Chem. Biol., 2016, 12(10), 822–830 CrossRef CAS PubMed.
- S. Wakayama, S. Kiyonaka, I. Arai, W. Kakegawa, S. Matsuda, K. Ibata, Y. L. Nemoto, A. Kusumi, M. Yuzaki and I. Hamachi, Chemical labelling for visualizing native AMPA receptors in live neurons, Nat. Commun., 2017, 8, 14850 CrossRef CAS PubMed.
- S. H. Fujishima, R. Yasui, T. Miki, A. Ojida and I. Hamachi, Ligand-directed acyl imidazole chemistry for labeling of membrane-bound
proteins on live cells, J. Am. Chem. Soc., 2012, 134(9), 3961–3964 CrossRef CAS PubMed.
- T. Yamaguchi, M. Asanuma, S. Nakanishi, Y. Saito, M. Okazaki, K. Dodo and M. Sodeoka, Turn-ON fluorescent affinity labeling using a small bifunctional O-nitrobenzoxadiazole unit, Chem. Sci., 2014, 5(3), 1021–1029 RSC.
- Y. Takaoka, Y. Nishikawa, Y. Hashimoto, K. Sasaki and I. Hamachi, Ligand-directed dibromophenyl benzoate chemistry for rapid and selective acylation of intracellular natural proteins, Chem. Sci., 2015, 6(5), 3217–3224 RSC.
- H. J. Chan, X. H. Lin, S. Y. Fan, J. Ru Hwu and K. T. Tan, Rapid and Selective Labeling of Endogenous Transmembrane Proteins in Living Cells with a Difluorophenyl Ester Affinity-Based Probe, Chem.–Asian J., 2020, 15(21), 3416–3420 CrossRef CAS PubMed.
- K. Matsuo, Y. Nishikawa, M. Masuda and I. Hamachi, Live-Cell Protein Sulfonylation Based on Proximity-driven N-Sulfonyl Pyridone Chemistry, Angew. Chem., Int. Ed. Engl., 2018, 57(3), 659–662 CrossRef CAS PubMed.
- T. Tamura, T. Ueda, T. Goto, T. Tsukidate, Y. Shapira, Y. Nishikawa, A. Fujisawa and I. Hamachi, Rapid labelling and covalent inhibition of intracellular native proteins using ligand-directed N-acyl-N-alkyl sulfonamide, Nat. Commun., 2018, 9(1), 1870 CrossRef PubMed.
- A. R. Katritzky, S. Rachwal and G. J. Hitchings, Benzotriazole - a Novel Synthetic Auxiliary, Tetrahedron, 1991, 47(16–17), 2683–2732 CrossRef CAS.
- J. X. Wang, G. M. Fang, Y. He, D. L. Qu, M. Yu, Z. Y. Hong and L. Liu, Peptide o-aminoanilides as crypto-thioesters for protein chemical synthesis, Angew. Chem., Int. Ed. Engl., 2015, 54(7), 2194–2198 CrossRef CAS.
- D. A. Holt, J. I. Luengo, D. S. Yamashita, H. J. Oh, A. L. Konialian, H. K. Yen, L. W. Rozamus, M. Brandt, M. J. Bossard, M. A. Levy, D. S. Eggleston, J. Liang, L. W. Schultz, T. J. Stout and J. Clardy, Design, Synthesis, and Kinetic Evaluation of High-Affinity Fkbp Ligands and the X-Ray Crystal-Structures of Their Complexes with Fkbp12, J. Am. Chem. Soc., 1993, 115(22), 9925–9938 CrossRef CAS.
- L. Laraia, A. Friese, D. P. Corkery, G. Konstantinidis, N. Erwin, W. Hofer, H. Karatas, L. Klewer, A. Brockmeyer, M. Metz, B. Scholermann, M. Dwivedi, L. Li, P. Rios-Munoz, M. Kohn, R. Winter, I. R. Vetter, S. Ziegler, P. Janning, Y. W. Wu and H. Waldmann, The cholesterol transfer protein GRAMD1A regulates autophagosome biogenesis, Nat. Chem. Biol., 2019, 15(7), 710–720 CrossRef CAS PubMed.
- Y. W. Wu and H. Waldmann, Toward the role of cholesterol and cholesterol transfer protein in autophagosome biogenesis, Autophagy, 2019, 15(12), 2167–2168 CrossRef CAS PubMed.
- M. Besprozvannaya, E. Dickson, H. Li, K. S. Ginburg, D. M. Bers, J. Auwerx and J. Nunnari, GRAM domain proteins specialize functionally distinct ER-PM contact sites in human cells, Elife, 2018, 7, e31019 CrossRef PubMed.
- J. Sandhu, S. Li, L. Fairall, S. G. Pfisterer, J. E. Gurnett, X. Xiao, T. A. Weston, D. Vashi, A. Ferrari, J. L. Orozco, C. L. Hartman, D. Strugatsky, S. D. Lee, C. He, C. Hong, H. Jiang, L. A. Bentolila, A. T. Gatta, T. P. Levine, A. Ferng, R. Lee, D. A. Ford, S. G. Young, E. Ikonen, J. W. R. Schwabe and P. Tontonoz, Aster Proteins Facilitate Nonvesicular Plasma Membrane to ER Cholesterol Transport in Mammalian Cells, Cell, 2018, 175(2), 514–529 CrossRef CAS PubMed.
- V. M. Krishnamurthy, G. K. Kaufman, A. R. Urbach, I. Gitlin, K. L. Gudiksen, D. B. Weibel and G. M. Whitesides, Carbonic anhydrase as a model for biophysical and physical-organic studies of proteins and protein-ligand binding, Chem. Rev., 2008, 108(3), 946–1051 CrossRef CAS PubMed.
- C. C. Wykoff, N. J. Beasley, P. H. Watson, K. J. Turner, J. Pastorek, A. Sibtain, G. D. Wilson, H. Turley, K. L. Talks, P. H. Maxwell, C. W. Pugh, P. J. Ratcliffe and A. L. Harris, Hypoxia-inducible expression of tumor-associated carbonic anhydrases, Cancer Res., 2000, 60(24), 7075–7083 CAS.
- S. Ivanov, S. Y. Liao, A. Ivanova, A. Danilkovitch-Miagkova, N. Tarasova, G. Weirich, M. J. Merrill, M. A. Proescholdt, E. H. Oldfield, J. Lee, J. Zavada, A. Waheed, W. Sly, M. I. Lerman and E. J. Stanbridge, Expression of hypoxia-inducible cell-surface transmembrane carbonic anhydrases in human cancer, Am. J. Pathol., 2001, 158(3), 905–919 CrossRef CAS PubMed.
- C. P. Potter and A. L. Harris, Diagnostic, prognostic and therapeutic implications of carbonic anhydrases in cancer, Br. J. Cancer, 2003, 89(1), 2–7 CrossRef CAS PubMed.
- D. H. Barnett, S. Sheng, T. H. Charn, A. Waheed, W. S. Sly, C. Y. Lin, E. T. Liu and B. S. Katzenellenbogen, Estrogen receptor regulation of carbonic anhydrase XII through a distal enhancer in breast cancer, Cancer Res., 2008, 68(9), 3505–3515 CrossRef CAS PubMed.
- J. H. Hong, E. Muhammad, C. Zheng, E. Hershkovitz, S. Alkrinawi, N. Loewenthal, R. Parvari and S. Muallem, Essential role of carbonic anhydrase XII in secretory gland fluid and HCO3 (-) secretion revealed by disease causing human mutation, J. Physiol., 2015, 593(24), 5299–5312 CrossRef CAS PubMed.
|
This journal is © The Royal Society of Chemistry 2022 |
Click here to see how this site uses Cookies. View our privacy policy here.