DOI:
10.1039/D1SC06026K
(Edge Article)
Chem. Sci., 2022,
13, 454-460
K3V2O3F4(IO3)3: a high-performance SHG crystal containing both five and six-coordinated V5+ cations†
Received
1st November 2021
, Accepted 5th December 2021
First published on 6th December 2021
Abstract
The combination of d0 transition metal oxofluorides with iodate anions helps to synthesize polar crystals. Herein, a novel polar crystal, K3V2O3F4(IO3)3, which is the first metal vanadium iodate with two types of V5+-centered polyhedra (VO4F2 octahedron and VO3F2 trigonal bipyramid), has been prepared hydrothermally. It crystallizes in the polar space group of Cmc21 and its structure displays an unprecedented 0D [V2O3F4(IO3)3]3− anion, which is composed of Λ-shaped cis-[VO2F2(IO3)2]3− and [VO2F2(IO3)]2− anions interconnected via the corner-sharing of one oxo anion. The synergy gained from the VO4F2, VO3F2 and IO3 groups resulted in K3V2O3F4(IO3)3 exhibiting both a strong second-harmonic generation (SHG) response (1.3 × KTiOPO4) under 2050 nm laser irradiation and a large birefringence (0.158 @ 2050 nm). This study provides a facile route for designing SHG materials by assembling various vanadium oxide-fluoride motifs and iodate anions into one compound.
Introduction
Polar crystals are one of the hotspots for scientific investigations, and are urgently needed in the fields of pyroelectrics, ferroelectrics, piezoelectrics and SHG optics. As an input laser passes through an SHG crystal, frequency doubling occurs, which can extend the spectral ranges of the output laser.1–3 Hence, the SHG crystals play a crucial role in modern laser science and technology. On the other hand, the birefringent crystals that can create and control the polarized light, are also highly needed in the laser industry.4–6 Notably, SHG efficiency and birefringence are primarily related to the polarization and anisotropic properties of crystal structures.7–9
The iodate anion is able to exhibit giant microscopic acentricity owing to the second-order Jahn–Teller (SOJT) distortion induced by the lone-pair electrons of the I5+ cation.10–12 Accordingly, the design and synthesis of novel metal iodates with aligned IO3 groups have attracted tremendous commercial and academic interest. Of great importance are metal iodates which can exhibit high SHG response (dij > 10 × KDP) or/and large birefringence (Δn > 0.15 @ 1064 nm).13–29 Recently, two new effective routes to high performance metal iodate SHG crystals have been developed. One is using the 2D [Bi2O2]2+ layer and 3D [BiF2]+ cationic network for the confined iodate anions to be arranged in a favorable additive fashion, which has successfully led to the discovery of BiO(IO3) (12.5 × KDP)20 and Bi(IO3)F2 (11.5 × KDP, 0.22 @ 1064 nm).21 On the other hand, the condensation of iodate anions can also lead to the formation of polyiodates with outstanding SHG or birefringence properties, such as GdI5O14 (15 × KDP)29 and SrI2O5F2 (0.180 @ 1064 nm).26
The V5+-centered polyhedra feature rich structural configurations and large optical anisotropy. The V5+ cation can be four, five and six coordinated with tetrahedral, trigonal-bipyramidal and octahedral geometries, respectively. Many metal vanadates featuring an isolated VO4 tetrahedron are able to display large SHG effects, such as Li3VO4 (6 × KDP), LiRb2VO4 (5 × KDP) and SrTa2V2O11 (0.5 × LiNbO3).30–32 Interestingly, K3V5O14 (100 × α-SiO2) contains a 2D [V5O14]3− layer involving both VO4 and VO5 groups.33 Moreover, introducing the V5+ cation into metal iodate might help in achieving large SHG effects, hence the vanadyl iodates are an ideal class of materials for the exploration of SHG crystals.34–42 The VO4 tetrahedron usually does not link with the IO3 units directly, so as to form mixed anion compounds. Only Zn2(VO4)(IO3) (6 × KDP) is SHG-active, which features isolated (VO4)3− and (IO3)− anions bridged by Zn2+ cations.36 The five-coordinated V5+ cation can be connected to 1D V-IO3 chains via the bridging of iodate units, such as the 1D [VO2(IO3)2]− chain in NaVO2(IO3)2(H2O) (20 × KDP, 0.22 @ 1064 nm).39 Besides, the SOJT effect of the V5+ cation can lead to the octahedron being distorted toward a corner (C4), an edge (C2) or a face (C3), such as the VO5F octahedron (C2 distortion) in CsVO2F(IO3) (1.1 × KTP),34 VO4F2 (C3 distortion) in α- and β-Ba2[VO2F2(IO3)2](IO3) (9 × KDP)35 and VO6 (C4 distortion) in K(VO)2O2(IO3)3 (3.6 × KTP).38 So far, however, all of the metal vanadyl iodates reported involve only a kind of V5+-centered polyhedron. It is of great interest but challenging if two different types of V-centered polyhedra can be combined into one metal iodate. In addition, exploring novel SHG materials with unprecedented crystal structures via the substitution and bridging of V-centered oxyfluoride groups such as the VO4F2 octahedron in 0D Λ-shaped cis-[VO2F2(IO3)2]3− polyanions is a facile route.34,35 We also hope that new SHG-active vanadium iodate-fluorides feature a smaller-dimensional anionic architecture in order to exhibit a larger birefringence.
Our explorations of the A+–V5+–(IO3)−–F− system have resulted in the discovery of K3V2O3F4(IO3)3, which features an unprecedented 0D [V2O3F4(IO3)3]3− binucleate polyanion, being composed of both the VO4F2 octahedron and VO3F2 trigonal-bipyramid. Significantly, K3V2O3F4(IO3)3 features a strong SHG effect of 1.3 × KTP under 2050 nm laser irradiation and a large birefringence of 0.158 @ 2050 nm.
Experimental
Materials and synthesis
Caution: Hydrofluoric acid is toxic and corrosive! It must be handled with extreme caution and appropriate protective equipment and training.
K2CO3 (>98%), V2O5 (>98%), HF (48–51%), and H5IO6 (98%), were used as purchased from Adamas-beta. Single crystals of K3V2O3F4(IO3)3 were obtained via hydrothermal reactions. The starting materials are V2O5, (91 mg, 0.5 mmol), K2CO3, (124.4 mg, 0.9 mmol), H5IO6 (250.8 mg, 1.1 mmol), HF (0.35 mL) and H2O (1 mL). A mixture of the starting materials was put into Teflon pouches (23 mL) sealed in an autoclave, which was heated at 230 °C for 72 hours and cooled to 30 °C at 4 °C h−1. Yellow brick-shaped crystals of K3V2O3F4(IO3)3 were obtained in high yields of ∼85% (based on I). The phase purity of K3V2O3F4(IO3)3 was checked by powder-XRD (Fig. S1†). The field-emission scanning electron microscopy (FESEM) analyses of K3V2O3F4(IO3)3 revealed the existence of K, V, I and F elements (Fig. S2†), and the molar ratio of K
:
V
:
I
:
F (1
:
64
:
1.53
:
2.46) is consistent with the compositions determined by single-crystal XRD analysis.
Powder X-ray diffraction
Powder X-ray diffraction (PXRD) patterns were recorded on a Rigaku MiniFlex II diffractometer with graphite-monochromated Cu Kα radiation in the 2θ range of 10–70° with a step size of 0.02°.
Energy-dispersive X-ray spectroscopy
Microprobe elemental analyses were performed on a field-emission scanning electron microscope (FESEM, JSM6700F) equipped with an energy-dispersive X-ray spectroscope (EDS, Oxford INCA).
Thermal analysis
Thermogravimetric analysis (TGA) and differential scanning calorimetry (DSC) were performed with a NETZCH STA 449F3 unit under a N2 atmosphere, at a heating rate of 10 °C min−1 in the range from 30 to 1000 °C.
Optical measurements
Infrared (IR) spectra were recorded on a Magna 750 FT-IR spectrometer in the form of KBr pellets in the range from 4000 to 400 cm−1.
Ultraviolet-visible-infrared (UV-vis-IR) spectra in the range of 200–2500 nm were recorded on a PerkinElmer Lambda 950 UV-vis-NIR spectrophotometer. Reflectance spectra were converted into absorption spectra by using the Kubelka–Munk function.43
Second harmonic generation measurements
Powder SHG measurements were taken with a Q switch Nd: YAG laser generating radiation at 2.05 μm according to the method of Kurtz and Perry.44 Crystalline K3V2O3F4(IO3)3 samples were sieved into distinct particle-size ranges (45–53, 53–75, 75–105, 105–150, 150–210, and 210–300 μm). Sieved KTiOPO4 (KTP) samples in the same particle-size range were used as references. The SHG response of α-Ba2[VO2F2(IO3)2](IO3),35 α-Ba2[GaF4(IO3)2](IO3)45 and γ-KMoO3(IO3)46 was remeasured under the same experimental conditions.
Single crystal structure determination
Single-crystal X-ray diffraction data for the title compound were collected on an Agilent Technologies SuperNova dual-wavelength CCD diffractometer with Mo Kα radiation (λ = 0.71073 Å) at 293 K. Data reduction was performed with CrysAlisPro, and the absorption correction based on the multi-scan method was applied.47 The structure of K3V2O3F4(IO3)3 was determined by the direct methods and refined by full-matrix least-squares fitting on F2 using SHELXL-2014.48 All of the non-hydrogen atoms were refined with anisotropic thermal parameters. The structure was checked for missing symmetry elements using PLATON, and none were found.49 The flack factor was refined to be −0.04(3), indicating the correctness of its absolute structure.50 Crystallographic data and structural refinements of the compound are listed in Table 1, and selected bond distances, as well as bond angles, are listed in Tables S1 and S2.†
Table 1 Crystallographic data for K3V2O3F4(IO3)3
R
1 = ∑‖Fo| − |Fc‖/∑|Fo| and wR2 = {∑w[(Fo)2 − (Fc)2]2/∑w[(Fo)2]2}1/2.
|
Formula |
K3V2O3F4(IO3)3 |
Formula weight |
867.88 |
Crystal system |
Orthorhombic |
Space group |
Cmc21 |
T (K) |
293(2) |
a (Å) |
11.6073(9) |
b (Å) |
8.5216(7) |
c (Å) |
15.0983(12) |
V (Å3) |
1493.41(20) |
Z
|
4 |
D
c (g cm3) |
3.860 |
μ (mm−1) |
8.393 |
Goodness of fit on F2 |
1.022 |
Flack factor |
−0.04(3) |
R
1, wR2 [I > 2σ(I)]a |
0.0272, 0.0655 |
R
1, wR2 (all data)a |
0.0286, 0.0664 |
Computational method
The electronic structure and optical properties were determined using a plane-wave pseudopotential method within density functional theory (DFT) implemented in the total energy code CASTEP.51,52 For the exchange and correlation functional, we chose Perdew–Burke–Ernzerhof (PBE) in the generalized gradient approximation (GGA).53 The interactions between the ionic cores and the electrons were described using the norm-conserving pseudopotential.54 The following orbital electrons were treated as valence electrons: I-5s25p5, V-3s23p63d34s2, K-3s23p64s1, O-2s22p4 and F-2s22p5. The numbers of plane waves included in the basis sets were determined using the cutoff energy of 850 eV. A Monkhorst–Pack k-point sampling of 2 × 2 × 3 was used to perform numerical integration of the Brillouin zone. During the optical property calculations, more than 900 empty bands were involved to ensure the convergence of linear optical properties and SHG coefficients. The other parameters and convergent criteria are the default values of the CASTEP code.51,52
The calculations of second-order NLO properties were based on length-gauge formalism within the independent-particle approximation.55 We adopted Chen's static formula, which was derived by Rashkeev et al.56 and later improved by Chen's group. The static second-order NLO susceptibility can be expressed as
χαβγ = χαβγ (VE) + χαβγ (VH) + χαβγ (two bands) |
where
χαβγ (VE) and
χαβγ (VH) contribute to
χαβγ from virtual-electron processes and virtual-hole processes, respectively, and
χαβγ (two bands) contributes to
χαβγ from the two-band processes. The formulae for calculating
χαβγ (VE), and
χαβγ (VH), are given in ref.
57.
Results and discussion
Crystal structure
K3V2O3F4(IO3)3 crystallizes in the polar space group Cmc21 (no. 36) (Table 1). Compared to previously discovered vanadium iodates, K3V2O3F4(IO3)3 exhibits a novel 0D [V2O3F4(IO3)3]3− binuclear polyanion, with K+ ions acting as the counter cations to achieve the charge balance (Fig. 1).
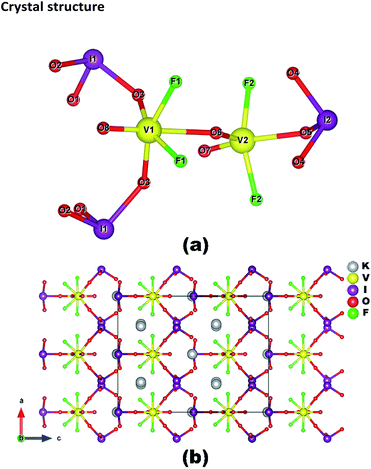 |
| Fig. 1 View of the structures 0D [V2O3F4(IO3)3]3− polyanion (a) and K3V2O3F4(IO3)3 along the b-axis (b). | |
The asymmetric unit of K3V2O3F4(IO3)3 contains 2 K, 2 V, 2 I, 2 F, and 8 O atoms, among which the K(1), V(1), V(2), I(2), and O(5)–O(8) atoms lie on the mirror plane whereas the remaining atoms are located at the general sites. All of the I5+ cations link with three O atoms with the bond lengths ranging from 1.782(6) to 1.882(6) Å and the lone-pair electrons occupy the open side of the IO3 trigonal pyramid.
The V(1) atom links with two oxo, two fluoride and two iodate anions to form a Λ-shaped cis-[VO2F2(IO3)2]3− polyanion (Fig. 1a). Within the V(1)O4F2 polyhedron, there are one short (1.576(10) Å) and one long (2.311(9) Å) V–O bonds as well as four normal (1.872(6) Å for V–O; 1.872(5) Å for V–F) bonds (Fig. S3a†). The cis- and trans-O/F–V(1)–O/F bond angles are in the range of 79.0(2)–101.7(3)° and 158.2(3)–178.9(4)°, respectively. Hence, the coordination geometry of the V(1)5+ cation is a distorted octahedron (C4 direction). The distortion magnitude, Δd is defined as the difference in the diagonal bond lengths divided by the cosine of the related angle. The Δd of the VO4F2 octahedron in K3V2O3F4(IO3)3 was calculated to be 0.74, which is larger than that of VO6 (0.62, C4 direction) in K(VO)2O2(IO3)3,38 but smaller than those of the VO4F2 octahedron (1.20, C3 direction) in α-Ba2[VO2F2(IO3)2](IO3)35 and VO5F (1.23, C2 direction) in CsVO2F(IO3).34
The V(2) atom is five-coordinated by two oxo, two fluoride anions, and one oxygen atom from an iodate anion (Fig. 1a). Within the V(2)O3F2 group, there are two short (1.633(9) and 1.700(9) Å) and one long (2.032(8) Å) V–O bonds as well as two normal (1.856(6) Å) V–F bonds (Fig. S3b†). The O–V(1)–O bond angles are in the range of 102.9(4)–150.3(4)° and the F–V(1)–F bond angle is 155.7(3)°. Hence, the V(2)O3F2 group can be described as a distorted tetragonal pyramid, which is similar to VO4F in β-Ba[VFO2(IO3)2].35
The Λ-shaped cis-[VO2F2(IO3)2]3− anion and the [VO2F2(IO3)]2− anion are bridged via the corner-sharing (O(6)) into a unique 0D [V2O3F4(IO3)3]3− polyanion (Fig. 1a). The 0D [V2O3F4(IO3)3]3− anion can also be regarded as a binuclear [V2O3F4] corner-sharing with three iodate anions, two on the six-coordinated V(1) center and one on the five-coordinated V(2) center. All of the K+ ions act as spacers between [V2O3F4(IO3)3]3− polyanions and maintain charge balance. K(1) is eight coordinated by one VO4F2 octahedron in a bidentate fashion (2F) and three IO3− units also in a bidentate fashion, whereas the K(2) atom is seven-coordinated by two oxygen atoms from two IO3− anions, one F and one O from a VO4F2 octahedron, and three F from one VO4F2 and two VO3F2 units (Fig. S4†). The K–F and K–O bond lengths are in the ranges of 2.621(6)–2.736(6) Å and 2.720(6)–3.067 (8) Å, respectively. The values of bond valence sum (BVS) are calculated to be 1.048, 0.962, 5.051, 4.794, 4.973, and 4.801 for K(1), K(2), V(1), V(2), I(1) and I(2), respectively, showing that their oxidation states are +1, +5 and +5.
Structural comparison
It is interesting to note that both the [V2O3F4(IO3)3]3− anion in K3V2O3F4(IO3)3 and [VO2F(IO3)]− anion in CsVO2F(IO3) can be related to the 0D Λ-shaped cis-[VO2F2(IO3)2]3− unit in α-Ba2[VO2F2(IO3)2](IO3), the first example of a metal vanadium iodate-fluoride.35 For the structural transformation from α-Ba2[VO2F2(IO3)2](IO3) to CsVO2F(IO3), the 0D [VO2F2(IO3)2]3− units are changed into 0D [VO3F4(IO3)2]4− units via the replacement of one F with one O atom, and then the neighboring 0D Λ-shaped units are bridged via the connections of axial oxide and iodate anions.34 However for K3V2O3F4(IO3)3, one (IO3)− group in one half 0D [VO2F2(IO3)2]3− unit was omitted, while the other half 0D [VO2F2(IO3)2]3− unit sustains the Λ-shaped configuration, and then such different neighboring 0D units are connected via the corner-sharing of one oxide anion only. Hence, the dimensionality of the [V2O3F4(IO3)3]3− anion in K3V2O3F4(IO3)3 is smaller than that of the [VO2F(IO3)]− anion in CsVO2F(IO3) (0D vs. 3D).
Thermal and optical properties
The thermogravimetric (TG) analyses revealed that K3V2O3F4(IO3)3 is thermally stable up to 220 °C (Fig. 2a). The IR spectrum of K3V2O3F4(IO3)3 indicates that the sharp absorption peaks mostly appear in the range of 400–1025 cm−1, which can be assigned to the V–O vibrations (824–994 cm−1) and I–O vibrations (411–783 cm−1) (Fig. S5†).34,35 The UV-vis-IR spectrum shows that K3V2O3F4(IO3)3 has a bandgap of 2.40 eV related to the absorption edge of 485 nm (Fig. 2b).
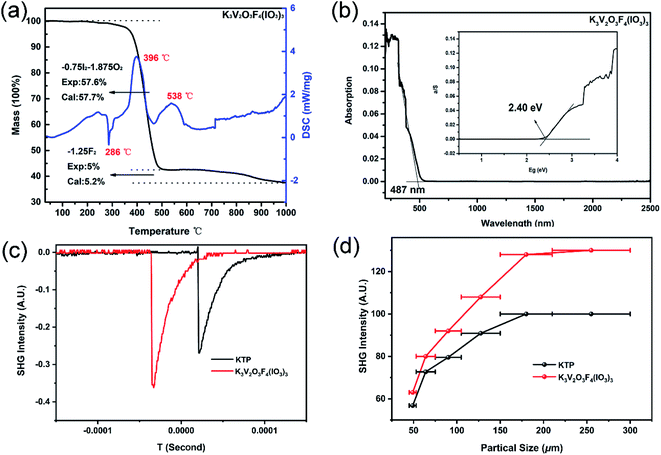 |
| Fig. 2 The TG-DSC curves (a), the UV-vis-IR spectrum (b), the measured oscilloscope traces of the SHG signals (150–210 μm) (c) and SHG intensity vs. particle size of compounds under 2050 nm laser irradiation (d). KTP served as the reference. | |
SHG properties
Under 2050 nm laser irradiation, K3V2O3F4(IO3)3 displays a very strong SHG signal, which is 1.3 times as large as that of KTP with a particle size of 150–210 μm. Furthermore, it is type-I phase-matchable (Fig. 2c and d). Compared to the metal iodates with Λ-shaped motifs such as K5(W3O9F4)(IO3) (1.0 × KTP),16 α-Ba2[GaF4(IO3)2](IO3) (0.4 × KTP)45 and γ-KMoO3(IO3) (1.1 × KTP)46 as well as the crystals containing different V-centered polyhedra such as K3V5O14 (100 × α-SiO2)33 and Pb2(V2O4F)(VO2)(SeO3)3 (0.3 × KDP),58 K3V2O3F4(IO3)3 features a higher SHG performance.
It is interesting to compare the SHG effect of K3V2O3F4(IO3)3 with previously reported vanadium iodates (Table S3†). The SHG response of K3V2O3F4(IO3)3 is stronger than that of other metal vanadium iodate-fluorides such as α-Ba2[VO2F2(IO3)2](IO3) (0.7 × KTP) and CsVO2F(IO3) (1.1 × KTP).34,35 However, compared with K(VO)2O2(IO3)3, K3V2O3F4(IO3)3 features a smaller SHG effect (1.3 vs. 3.6 × KTP), which can be attributed to the well-arranged VO6 octahedra and IO3 groups in the 1D [(VO)2O2(IO3)3]− chain in K(VO)2O2(IO3)3. Hence, we consider that the ideal alignment of vanadium oxide-fluoride and iodate anions may lead to an outstanding SHG effect, which is an effective route for the exploration of high-performance SHG crystals.
Structure–property relationship analysis
The calculation of the band structure showed that K3V2O3F4(IO3)3 is an indirect bandgap compound with a bandgap of 2.212 eV, which is smaller than the 2.40 eV from our UV-vis-IR measurements (Fig. 3a). Therefore, a small scissor of 0.188 eV was used for the more accurate description of optical properties.
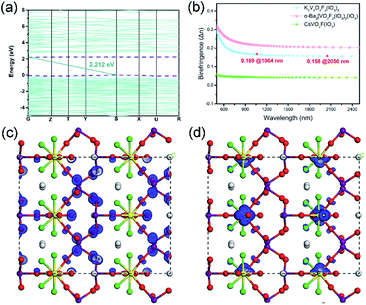 |
| Fig. 3 The calculated band structure (a), the birefringence (b), and the SHG density plots: (c) VB and (d) CB for K3V2O3F4(IO3)3. | |
The partial density of states (PDOS) graph of K3V2O3F4(IO3)3 is shown in Fig. S6.† The highest valence band (VB) is occupied by the O-2p states whereas the lowest conduction band (CB) is occupied by the large, medium and small amounts of V-4d, O-2p and I-5p states, respectively. Hence, V, I and O atoms dominated the bandgap of K3V2O3F4(IO3)3.
Calculations of the birefringent properties of K3V2O3F4(IO3)3 were performed (Fig. 3b). The birefringence of K3V2O3F4(IO3)3 is calculated to be 0.158 @ 2050 nm, which is large enough to achieve phase-matching. Compared to previously reported metal vanadium iodate-fluorides including α-Ba2[VO2F2(IO3)2](IO3) and CsVO2F(IO3),34,35 reduction of the structural dimension from the 3D [VO2F(IO3)]− framework to the 0D unit produces a large enhancement of optical anisotropy. Hence, the birefringence of K3V2O3F4(IO3)3 (0.158 @ 2050 nm) and α-Ba2[VO2F2(IO3)2](IO3) (0.200 @ 2050 nm) is far larger than that of CsVO2F(IO3) (0.04 @ 2050 nm).34,45 Additionally, the birefringence of K3V2O3F4(IO3)3 at 1064 nm (0.168) is also larger than that of K5(W3O9F4)(IO3) (0.083 @ 1064 nm),16 α-Ba2[GaF4(IO3)2](IO3) (0.126 @ 1064 nm)45 and γ-KMoO3(IO3) (0.087 @ 1064 nm)46 with Λ-shaped motifs.
The specific SHG contributions from the respective groups have also been studied by DFT calculations. The calculated non-vanishing independent SHG tensors are d15 = 15.23 pm V−1, d24 = 1.98 pm V−1, and d33 = 11.46 pm V−1. Notably, the largest SHG d15 tensor is 15.23 pm V−1, which is larger than that of CsVO2F(IO3) (13.9 pm V−1), α-Ba2[VO2F2(IO3)2](IO3) (d31 = 4.68 pm V−1), γ-KMoO3(IO3) (d11 = 5.76 pm V−1) and α-Ba2[GaF4(IO3)2](IO3) (d33 = 1.93 pm V−1).34,35,45,46 The calculated results are roughly consistent with the SHG effect magnitude: K3V2O3F4(IO3)3 (1.3 × KTP) > CsVO2F(IO3) (1.1 × KTP) = γ-KMoO3(IO3) (1.1 × KTP) > α-Ba2[VO2F2(IO3)2](IO3) (0.7 × KTP) > α-Ba2[GaF4(IO3)2](IO3) (0.4 × KTP).34,35,45,46
Furthermore, the SHG origins of K3V2O3F4(IO3)3 were investigated and the SHG density maps are shown in Fig. 3c and d. The dominating SHG source is the non-bonding O-2p states in the VB, whereas, in the CB, the empty V-3d states, O-2p states from V–O–I bridges, and few F-2p states define the SHG response of K3V2O3F4(IO3)3. The SHG contribution percentages of VO4F2, VO3F2, and IO3 groups are 32.5%, 15.6%, and 50.2%, respectively. Accordingly, we suggest that assembling different vanadium oxide fluoride motifs and iodate anions produces a synergistic effect, and led to a strong SHG response for K3V2O3F4(IO3)3.
Conclusions
In conclusion, explorations of metal vanadium iodate-fluorides afforded K3V2O3F4(IO3)3 with a 0D [V2O3F4(IO3)3]3− polyanion, which is composed of three types of SHG functional groups (VO4F2 octahedron, VO3F2 square pyramid, and IO3 triangular pyramid). Interestingly, K3V2O3F4(IO3)3 exhibits a very strong SHG effect (1.3 × KTP) and a large birefringence (0.158 @ 2050 nm). Therefore, K3V2O3F4(IO3)3 is a promising SHG crystal. Further studies to explore new metal iodate SHG materials containing two different d0-TM centered polyhedra are in progress.
Data availability
All data included in this study are available upon request by contact with the corresponding author.
Author contributions
Chen Jin: conceptualization, methodology, writing – original draft, data curation, visualization, writing – review & editing; Li Yi-Lin, Chen Yan and Chen Qian-Qian: data curation; Hu Chun-Li: formal analysis, software; Mao Jiang-Gao: supervision.
Conflicts of interest
There are no conflicts to declare.
Acknowledgements
Our work has been supported by the National Natural Science Foundation of China (No. 22031009, 21875248, 21921001, and 21975256).
Notes and references
- M. Mutailipu, K. R. Poeppelmeier and S. Pan, Chem. Rev., 2021, 121, 1130–1202 CrossRef CAS PubMed.
- K. M. Ok, E. O. Chi and P. S. Halasyamani, Chem. Soc. Rev., 2006, 35, 710–717 RSC.
- G. Zou and K. M. Ok, Chem. Sci., 2020, 11, 5404–5409 RSC.
- X. Meng, W. Yin and M. Xia, Coord. Chem. Rev., 2021, 439, 213916 CrossRef CAS.
- M. Zhang, D. An, C. Hu, X. Chen, Z. Yang and S. Pan, J. Am. Chem. Soc., 2019, 141, 3258–3264 CrossRef CAS PubMed.
- C. Wu, T. Wu, X. Jiang, Z. Wang, H. Sha, L. Lin, Z. Lin, Z. Huang, X. Long, M. G. Humphrey and C. Zhang, J. Am. Chem. Soc., 2021, 143, 4138–4142 CrossRef CAS PubMed.
- C. Jin, X. Shi, H. Zeng, S. Han, Z. Chen, Z. Yang, M. Mutailipu and S. Pan, Angew. Chem., Int. Ed., 2021, 60, 20469–20475 CrossRef CAS PubMed.
- X. Zhang, L. Kang, P. Gong, Z. Lin and Y. Wu, Angew. Chem., Int. Ed., 2021, 60, 6386–6390 CrossRef CAS PubMed.
- J. Shi, Y. He, F. Liang, X. Zhang, D. Xu, J. Yao, G. Zhang, Z. Hu, J. Yao and Y. Wu, J. Mater. Chem. C, 2020, 8, 4226–4233 RSC.
- J. Chen, C. L. Hu, F. Kong and J. G. Mao, Acc. Chem. Res., 2021, 54, 2775–2783 CrossRef CAS PubMed.
- C. L. Hu and J. G. Mao, Coord. Chem. Rev., 2015, 288, 1–17 CrossRef CAS.
- C. Sun, B. Yang and J. Mao, Sci. China: Chem., 2011, 54, 911 CrossRef CAS.
- H.-Y. Chang, S.-H. Kim, P. S. Halasyamani and K. M. Ok, J. Am. Chem. Soc., 2009, 131, 2426–2427 CrossRef CAS PubMed.
- C. F. Sun, C. L. Hu, X. Xu, J. B. Ling, T. Hu, F. Kong, X. F. Long and J. G. Mao, J. Am. Chem. Soc., 2009, 131, 9486–9487 CrossRef CAS PubMed.
- R. E. Sykora, K. M. Ok, P. S. Halasyamani and T. E. Albrecht-Schmitt, J. Am. Chem. Soc., 2002, 124, 1951–1957 CrossRef CAS.
- C. Wu, L. Lin, X. Jiang, Z. Lin, Z. Huang, M. G. Humphrey, P. S. Halasyamani and C. Zhang, Chem. Mater., 2019, 31, 10100–10108 CrossRef CAS.
- C. Huang, C. L. Hu, X. Xu, B. P. Yang and J. G. Mao, Inorg. Chem., 2013, 52, 11551–11562 CrossRef CAS PubMed.
- J. Chen, C.-L. Hu, F.-F. Mao, X.-H. Zhang, B.-P. Yang and J.-G. Mao, Chem. Sci., 2019, 10, 10870–10875 RSC.
- H. Liu, X. Jiang, X. Wang, L. Yang, Z. Lin, Z. Hu, X.-G. Meng, X. Chen and J. Qin, J. Mater. Chem. C, 2018, 6, 4698–4705 RSC.
- S. D. Nguyen, J. Yeon, S. H. Kim and P. S. Halasyamani, J. Am. Chem. Soc., 2011, 133, 12422–12425 CrossRef CAS PubMed.
- F. F. Mao, C. L. Hu, X. Xu, D. Yan, B. P. Yang and J. G. Mao, Angew. Chem., Int. Ed., 2017, 56, 2151–2155 CrossRef CAS PubMed.
- H. Liu, Q. Wu, X. Jiang, Z. Lin, X. Meng, X. Chen and J. Qin, Angew. Chem., Int. Ed., 2017, 56, 9492–9496 CrossRef CAS.
- M. Zhang, X. Su, M. Mutailipu, Z. Yang and S. Pan, Chem. Mater., 2017, 29, 945–949 CrossRef CAS.
- J. Chen, C.-L. Hu and J.-G. Mao, Sci. China Mater., 2021, 2, 400–407 CrossRef.
- M. Gai, Y. Wang, T. Tong, Z. Yang and S. Pan, Inorg. Chem., 2020, 59, 4172–4175 CrossRef CAS PubMed.
- M. Gai, T. Tong, Y. Wang, Z. Yang and S. Pan, Chem. Mater., 2020, 32, 5723–5728 CrossRef CAS.
- H. Fan, C. Lin, K. Chen, G. Peng, B. Li, G. Zhang, X. Long and N. Ye, Angew. Chem., Int. Ed., 2020, 59, 5268–5272 CrossRef CAS PubMed.
- T. Abudouwufu, M. Zhang, S. Cheng, H. Zeng, Z. Yang and S. Pan, Chem. Mater., 2020, 32, 3608–3614 CrossRef CAS.
- J. Chen, C.-L. Hu, F.-F. Mao, B.-P. Yang, X.-H. Zhang and J.-G. Mao, Angew. Chem., Int. Ed., 2019, 58, 11666–11669 CrossRef CAS PubMed.
- X. Su, Y. Chu, Z. Yang, B.-H. Lei, C. Cao, Y. Wang, Q. Liu and S. Pan, J. Phys. Chem. C, 2020, 124, 24949–24956 CrossRef CAS.
- X. Su, Z. Yang, G. Han, Y. Wang, M. Wen and S. Pan, Dalton Trans., 2016, 45, 14394–14402 RSC.
- A. K. Paidi, P. W. Jaschin, K. B. R. Varma and K. Vidyasagar, Inorg. Chem., 2017, 56, 12631–12640 CrossRef CAS PubMed.
- J. Yeon, S.-H. Kim and P. S. Halasyamani, Inorg. Chem., 2010, 49, 6986–6993 CrossRef CAS PubMed.
- J. Chen, C.-L. Hu, X.-H. Zhang, B.-X. Li, B.-P. Yang and J.-G. Mao, Angew. Chem., Int. Ed., 2020, 59, 5381–5384 CrossRef CAS PubMed.
- H. Yu, M. L. Nisbet and K. R. Poeppelmeier, J. Am. Chem. Soc., 2018, 140, 8868–8876 CrossRef CAS PubMed.
- B. P. Yang, C. L. Hu, X. Xu, C. Huang and J. G. Mao, Inorg. Chem., 2013, 52, 5378–5384 CrossRef CAS PubMed.
- C. Huang, C.-L. Hu, X. Xu, B.-P. Yang and J.-G. Mao, Dalton Trans., 2013, 42, 7051–7058 RSC.
- C. F. Sun, C. L. Hu, X. Xu, B. P. Yang and J. G. Mao, J. Am. Chem. Soc., 2011, 133, 5561–5572 CrossRef CAS PubMed.
- B. P. Yang, C. L. Hu, X. Xu, C. F. Sun, J. H. Zhang and J. G. Mao, Chem. Mater., 2010, 22, 1545–1550 CrossRef CAS.
- C. F. Sun, T. Hu, X. Xu and J. G. Mao, Dalton Trans., 2010, 39, 7960–7967 RSC.
- X. A. Chen, L. Zhang, X. A. Chang, H. G. Zang and W. Q. Xiao, Acta Crystallogr., Sect. C: Cryst. Struct. Commun., 2006, 62, i76–i78 CrossRef PubMed.
- R. E. Sykora, K. M. Ok, P. S. Halasyamani, D. M. Wells and T. E. Albrecht-Schmitt, Chem. Mater., 2002, 14, 2741–2749 CrossRef CAS.
- P. Kubelka and F. Munk, Z. Tech. Phys., 1931, 12, 259–274 Search PubMed.
- S. K. Kurtz and T. T. Perry, J. Appl. Phys., 1968, 39, 3798–3813 CrossRef CAS.
- J. Chen, C.-L. Hu, F.-F. Mao, J.-H. Feng and J.-G. Mao, Angew. Chem., Int. Ed., 2019, 58, 2098–2102 CrossRef CAS PubMed.
- J. Chen, C.-L. Hu, Y.-L. Li, Q.-Q. Chen, B.-X. Li and J.-G. Mao, J. Alloys Compd., 2021, 162547, DOI:10.1016/j.jallcom.2021.162547.
- R. H. Blessing, Acta Crystallogr., Sect. A: Found. Crystallogr., 1995, 51, 33–38 CrossRef PubMed.
- G. M. Sheldrick, Acta Crystallogr., Sect. C: Struct. Chem., 2015, 71, 3–8 Search PubMed.
- A. Spek, J. Appl. Crystallogr., 2003, 36, 7–13 CrossRef CAS.
-
H. D. Flack and G. Bernardinelli, The use of X-ray crystallography to determine absolute configuration, 2008, vol. 20, pp. 681–690 Search PubMed.
- M. Segall, P. J. Lindan, M. a. Probert, C. J. Pickard, P. J. Hasnip, S. Clark and M. Payne, J. Phys.: Condens. Matter, 2002, 14, 2717 CrossRef CAS.
- V. Milman, B. Winkler, J. White, C. Pickard, M. Payne, E. Akhmatskaya and R. Nobes, Int. J. Quantum Chem., 2000, 77, 895–910 CrossRef CAS.
- J. P. Perdew, K. Burke and M. Ernzerhof, Phys. Rev. Lett., 1996, 77, 3865 CrossRef CAS PubMed.
- J. Lin, A. Qteish, M. Payne and V. Heine, Phys. Rev. B: Condens. Matter Mater. Phys., 1993, 47, 4174 CrossRef PubMed.
- C. Aversa and J. Sipe, Phys. Rev. B: Condens. Matter Mater. Phys., 1995, 52, 14636 CrossRef CAS PubMed.
- S. N. Rashkeev, W. R. Lambrecht and B. Segall, Phys. Rev. B: Condens. Matter Mater. Phys., 1998, 57, 3905 CrossRef CAS.
- J. Lin, M.-H. Lee, Z.-P. Liu, C. Chen and C. J. Pickard, Phys. Rev. B: Condens. Matter Mater. Phys., 1999, 60, 13380 CrossRef CAS.
- L. Lin, X. Jiang, C. Wu, Z. Lin, Z. Huang, M. G. Humphrey and C. Zhang, Dalton Trans., 2021, 50, 7238–7245 RSC.
Footnote |
† Electronic supplementary information (ESI) available. CCDC 2093711. For ESI and crystallographic data in CIF or other electronic format see DOI: 10.1039/d1sc06026k |
|
This journal is © The Royal Society of Chemistry 2022 |
Click here to see how this site uses Cookies. View our privacy policy here.