DOI:
10.1039/D1SC06578E
(Edge Article)
Chem. Sci., 2022,
13, 3140-3146
Porous nanographene formation on γ-alumina nanoparticles via transition-metal-free methane activation†
Received
25th November 2021
, Accepted 22nd February 2022
First published on 22nd February 2022
Abstract
γ-Al2O3 nanoparticles promote pyrolytic carbon deposition of CH4 at temperatures higher than 800 °C to give single-walled nanoporous graphene (NPG) materials without the need for transition metals as reaction centers. To accelerate the development of efficient reactions for NPG synthesis, we have investigated early-stage CH4 activation for NPG formation on γ-Al2O3 nanoparticles via reaction kinetics and surface analysis. The formation of NPG was promoted at oxygen vacancies on (100) surfaces of γ-Al2O3 nanoparticles following surface activation by CH4. The kinetic analysis was well corroborated by a computational study using density functional theory. Surface defects generated as a result of surface activation by CH4 make it kinetically feasible to obtain single-layered NPG, demonstrating the importance of precise control of oxygen vacancies for carbon growth.
Introduction
Graphene is a two-dimensional (2D) allotrope of carbon arranged in a planar hexagonal lattice that displays high elasticity and electronic/thermal conductivity.1 When pentagons are introduced into the hexagonal frameworks, ring closure takes place with a positive curvature to give fullerenes.2 When 2D graphene is wrapped into a cylindrical structure, a 1D carbon nanotube could be obtained.3–5 In contrast, the synthesis of a three-dimensionally and periodically arranged single-walled graphene with a negative curvature has remained a challenge since Mackay and Terrones proposed an ideal 3D minimum-surface graphene structure (Scheme 1) in 1991,6 despite the recent advances in organic synthesis realizing small molecules with a few heptagons7,8 or octagons.9,10
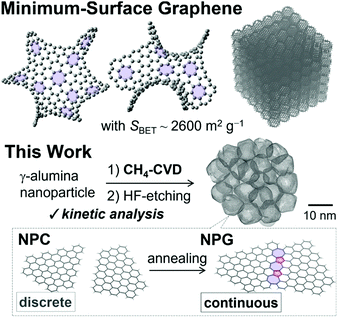 |
| Scheme 1 (top) Schematic of the minimum-surface graphene analogue originally reported by Mackay and Terrones.6 (bottom) Schematic of the synthesis of single-walled nanoporous carbon (NPC) and its conversion to single-walled nanoporous graphene (NPG) by the fusion of edge sites.13–16 | |
In this regard, chemical vapor deposition (CVD) on templated materials11–24 is a prominent strategy for realizing 3D minimum-surface graphene. Especially, CVD on alumina nanoparticles (ANPs) that display high thermal stability25 as the templates gave nanoporous graphene (NPG) materials from CH4.14–17 The NPGs have a 3D continuous and seamless nanostructure with a large surface area, approaching the ideal value of 2D graphene (2627 m2 g−1).26 NPGs also have fascinating features including high electrical conductivity,16 elastic and flexible nature,15,17 and unprecedentedly high electrochemical stability.16,17
The typical synthesis of NPG is performed as follows: a uniform carbon coating via CVD of CH4 at 900 °C on ANPs is followed by the removal of ANPs by chemical washing, and the subsequent high-temperature annealing at temperatures higher than 1600 °C under an inert atmosphere to give a single-walled NPG material (Scheme 1 and Fig. S1†).14–17 It is essential to use stable CH4 with a high bond dissociation energy (439 kJ mol−1)27,28 as a carbon source, rather than more reactive unsaturated hydrocarbons such as acetylene and propylene, for high-quality graphene formation by suppressing carbon stacking.
Understanding the type of reaction steps involved in the CH4-CVD process will represent a breakthrough in the synthesis of sophisticated nanoporous carbon materials, by using tastefully designed templates at lower temperatures via CH4-CVD. We recently reported the radical activation of CH4 on MgO,24 but the reaction mechanism on ANPs may vary owing to the differences in the geometry and electronic structure of the active site. Such a fundamental understanding of CH4 chemistry on the surfaces of metal oxides could also help us to efficiently activate hydrocarbons29–49 by controlling coke deposition at the molecular level.
In this work, we investigated the early-stage CH4 activation toward the formation of NPGs on γ-Al2O3 nanoparticles via reaction kinetics using thermogravimetry-mass spectrometry (TG-MS) and density functional theory (DFT), and surface analysis with high-resolution annular dark-field scanning transmission electron microscopy (ADF-STEM), temperature-programmed desorption (TPD) of H2O, and in situ infrared (IR) spectroscopy. We found that the formation of NPG is promoted at oxygen vacancies on (100) surfaces of γ-Al2O3, which are generated at temperatures higher than 800 °C in the presence of CH4. No transition metal reaction center was involved in CH4-CVD. This process is completely different from conventional methane activation catalysis for graphene50–57 and carbon nanotube58–61 growth at 1000 °C, oxidative39–41 and non-oxidative42 coupling to ethylene, and partial oxidation to methanol,44–49 which use transition metal elements as reaction centers. We first discuss the rate-limiting step of NPG formation on ANPs based on reaction kinetics using TG-MS and a reaction pathway search using DFT. We constructed a model (100) γ-Al2O3 surface based on high-resolution ADF-STEM, TPD of H2O, and in situ IR spectroscopy. Next, we discuss the strategy to further enhance the formation of NPG based on the surface activation process monitored by TG-MS and in situ IR spectroscopy. Details of the experimental and computational methods are provided in the ESI.†
Results and discussion
Kinetic analysis
Fig. 1a–d shows the dependence of the rate of CH4-CVD on the partial pressure of CH4 and reaction temperature using TG. The vertical axis shows the nominal number of layers of deposited carbon on ANPs calculated by the specific surface area of ANPs.17 The rate of carbon growth was pseudo-first order with respect to the partial pressure of CH4 for both first- and second-layer depositions (Fig. 1c). The formation of single-layered nanographene at a specific time of reaction was confirmed by the red-shifted strong G′-band17 of Raman spectra (Fig. S1†). This pseudo-linear dependence of the rate of carbon growth on CH4 partial pressure suggests that the rate-limiting step of NPG growth may be the initial CH4 activation52,62 on the surface. The rate of carbon growth for the first layer was faster than those for the second and third layers, as shown in Fig. 1a and b. We evaluated the effective activation energies ΔE≠ for the first- and second-layer carbon growth from the Arrhenius plots as shown in Fig. 1d, and obtained ΔE≠ = 124 kJ mol−1 and 308 kJ mol−1, respectively. The latter value is in a good agreement with the activation energy for CH4 decomposition onto the surface of carbon films (303 kJ mol−1).63 Thus, the initial carbon deposition on the γ-ANP surface was kinetically more favorable than the subsequent carbon deposition on the deposited carbon surface, and this makes it feasible to selectively form single-walled porous nanographene. The carbon deposition proceeded after an induction time of 5–10 min. Transient evolutions of CO, H2, and H2O were also observed in the effluent gas of CH4-CVD reaction during the induction period as shown in Fig. 1e and f. To check the effect of H2 on the activation of oxide surfaces, we examined H2 treatment of ANPs at 900 °C for 30 min before CH4-CVD. However, there was no significant difference observed in the rate of reactions by TG (Fig. S2†). These indicate that CH4 chemically activated γ-ANPs and resulted in reactive surfaces for CH4-CVD.
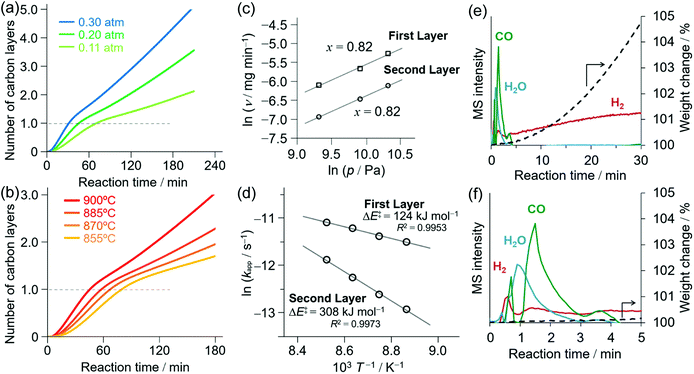 |
| Fig. 1 Kinetic analysis of CH4-CVD for porous nanographene. (a) Weight changes during CH4-CVD for various partial pressures of CH4 at 900 °C as monitored by TG. CH4 was introduced to the reactor at 0 min. (b) Weight changes during CH4-CVD on γ-ANPs at various temperatures as monitored by TG. (c) CH4 partial pressure dependence on the rate of carbon growth v at 900 °C. (d) Arrhenius plots for the first- and second-layer deposition. P/P0 = 0.2 for CH4 supply; the total rate of flow was fixed at 100 mL min−1. (e) TG-MS analysis of CH4-CVD under a steady flow of He (80 mL min−1) and CH4 (20 mL min−1) at 900 °C showing gas evolution for H2, H2O, and CO as well as the TG curve (dashed line). (f) Enlarged view of Fig. 1e showing the transient evolution of H2O and CO. | |
Surface analysis
To clarify the structure of the reaction center of the NPG growth on γ-ANP (first layer), we next investigated the structure of the reaction sites on γ-ANP by ADF-STEM, TPD of H2O, and in situ IR spectroscopy, and found that the oxygen vacancy sites of the partially hydrated (100) surface of γ-ANP are potential reaction centers.
The high-resolution ADF-STEM images (Fig. 2a, b and S3†) indicate that γ-ANPs had {100} as one of the main facets. The corresponding fast Fourier transformed (FFT) image was consistent with the neutron diffraction pattern for the (100) surface of γ-Al2O3.64 The γ-ANPs were prepared by hydrothermal synthesis, and the amount of transition metal impurities in the γ-ANPs was negligible according to our elemental analysis (for details, see the ESI†). We did not observe dispersion of any transition metal impurities on the surfaces in the ADF-STEM images.
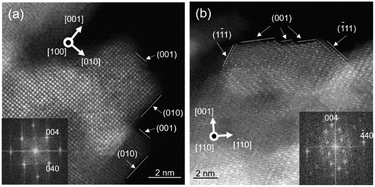 |
| Fig. 2 High resolution ADF-STEM image of γ-ANPs. (a) [100] and (b) [110] orientations. The insets of (a) and (b) are the corresponding FFT images. | |
TG analysis of CH4-CVD suggests that reaction temperatures above 800 °C are essential for carbon growth from CH4. We analyzed the effects of temperature on the surface structure of γ-ANPs by IR and TPD (Fig. 3a and b) up to 900 °C. The IR spectra showed desorption of H2O and depletion of surface-bound hydroxyl groups (3800–3000 cm−1)65,66 as the operating temperature increased under a steady flow of inert gases. Water desorption became almost negligible after 30 min at 900 °C as shown in the TPD (Fig. 3b), but a sharp absorption band in the IR spectrum centered at 3701 cm−1 originating from “isolated” hydroxyl groups25,67 still remained (Fig. 3a). This indicates that terminal μ1-hydroxyl groups existed even at such high temperature. Although the isolated hydroxyl group was labile toward proton exchange upon exposure to CD4 at temperatures higher than 650 °C (Fig. S4 and S5†), no deposition of carbon occurred at lower temperatures. This supports that further activation of surfaces by the formation of oxygen vacancies,68,69 as observed by TG-MS (Fig. 1e and f) at higher temperatures in the presence of CH4, is crucial for carbon growth to take place.
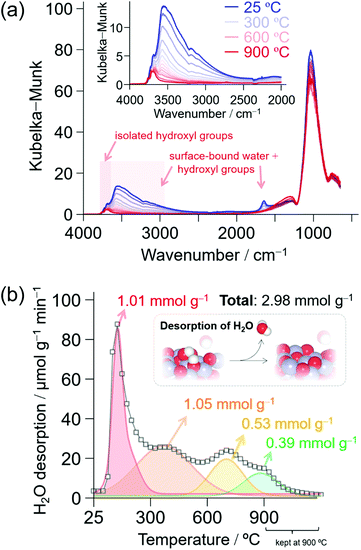 |
| Fig. 3 H2O desorption profile of γ-ANP: (a) Temperature dependence of IR spectra under a steady flow of Ar at 5 mL min−1. (b) TPD profile of H2O desorption from the surfaces of γ-ANP at 10 K min−1 quantified by GC (TCD). Gas: He flow at 200 mL min−1. The inset shows a schematic of water desorption from two protons and an oxide to give a surface defect. The thermal treatment under inert gas atmosphere was immediately followed by CH4-CVD (Fig. 1) by introducing CH4. | |
DFT calculations
To further examine the reaction mechanism, we constructed a partially dehydrated γ-Al2O3 (100) surface model with an oxygen vacancy. We evaluated the reaction pathway of the initial CH4 activation on the vacancy site using the plane-wave DFT and climbing-image nudged elastic band methods under periodic boundary conditions. We used the Perdew–Burke–Ernzerhof (PBE) exchange-correlation functional70 combined with Grimme's DFT-D3 empirical dispersion correction71 to account for the van der Waals interactions (PBE-D3). For details of the DFT calculations including the computational methods and structures of the intermediates and transition states involved in the CH4 activation on γ-Al2O3 (100), see Sections S1.4 and S3 of the ESI.†
We found that the dissociative adsorption of CH4 on the oxygen vacancy is the rate-limiting step, which is in agreement with our experiments, and that the dissociative addition undergoes in terms of the Lewis acid-base mechanism.25,72–74
We first examined CH4 activation on a 5-coordinated Al site (Fig. S6†).66 Methanol formation is expected on the oxide surface from CH4, and this is followed by desorption of the molecule to give an oxygen vacancy (4-coordinated Al site), as shown in Fig. S7.† The released methanol will decompose in the gas phase to afford CO and H2 at the operating temperatures of 800–900 °C,75 and this was confirmed by TG-MS analysis (Fig. 1f). With this in mind, we then investigated CH4 activation on the 4-coordinated Al site.
Fig. 4a shows the calculated potential energy profile of CH4 activation on the partially dehydrated γ-Al2O3 (100) model surface (Fig. S7†), from the physisorption of CH4 to the formation of surface-bound methylene
. The physisorption energy ΔEad is –20 kJ mol−1. The subsequent dissociative addition of adsorbed
gives a surface-bound methyl group
and hydrogen atom (H*) with the activation energy ΔEa,TS1 of 140 kJ mol−1. For surface reactions with small ΔEad, as in the present case, the effective activation energy ΔE≠ of the overall reaction evaluated as the sum of ΔEa,TS1 and ΔEad (ref. 76) is 120 kJ mol−1. This theoretical value is in excellent agreement with the experimental value of 124 kJ mol−1 (Fig. 1d).
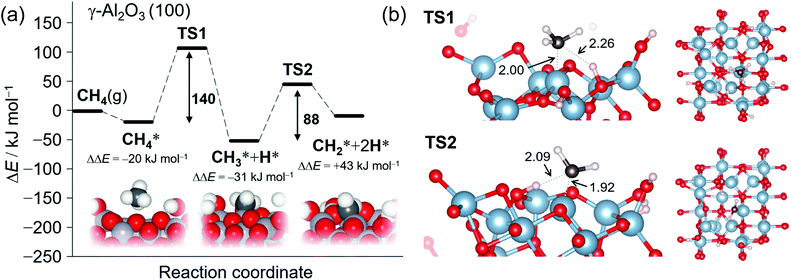 |
| Fig. 4 (a) Potential energy profile for the sequential C–H bond cleavage from a CH4 σ complex on a γ-Al2O3 (100) surface with a surface defect. The Al atom on the reaction site is set to be 4-coordinate. Activation energies were computed with the PBE-D3 functional and the corresponding structures of the initial, intermediate, and final states. The asterisk indicates the surface-bound species. Geometry for the oxygen defect is also shown in Fig. S7.† (b) Obtained structures of TS1 and TS2. Red: oxygen, steel blue; aluminum, black: carbon, and white: hydrogen atoms, with distances given in Ångströms. | |
Bader charges77 {q} of the transition state (TS) structure for the dissociative addition (TS1) in Fig. 5 clearly show that this reaction proceeds heterolytically following an acid-base mechanism, as suggested by the surface characterizations. Hδ+ (q = +0.66) interacts with the oxygen Lewis base site (q = −1.49), while CH3δ– (q = −0.65) is bound to the aluminum Lewis acid site (q = +2.35) at TS1. Pyramidal CH3δ– at TS1 also supports the Lewis acid–base mechanism.72–74 The expected value for the square of the total spin angular momentum, <S2> = 0.023 at TS1, indicates significant electron pairing during the dissociative adsorption. The counter plot of the difference charge density in TS1 (Fig. 5) suggests that the large overlap between the doubly occupied 2p orbital at CH4 and the vacant 3p orbital at the bare Al atom promotes the reaction.
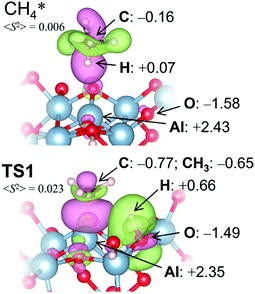 |
| Fig. 5 Charge difference profiles and Bader charges of selected atoms/groups on a γ-Al2O3 (100) surface with a defect. Green contour: positive charges, pink contour: negative charges. | |
After dissociative adsorption, the surface
is converted to
with a considerably lower activation energy (ΔEa = 88 kJ mol−1) via a proton transfer transition state TS2. The formed reactive
species could then undergo a coupling reaction with another
, which would result in the formation of
and longer hydrocarbons on the surface, as reported in the conversion of surface bound-methylene to a class of graphene materials on Al2O3 surfaces.78 Although we cannot exclude the possibility that
will desorb to the gas phase,42,79 selective formation of single-layered NPG (Fig. S1†) indicates that this surface reaction rather than a gas-phase reaction enhances the first-layer deposition of carbon.
The activation energy for the first step of CH4 activation
on a non-defective hydrated γ-Al2O3 (100) surface (ΔE≠) was 244 kJ mol−1 at an octahedrally coordinated (six-coordinated) Al site (Fig. S8†). This value is much higher than 120 kJ mol−1 at a tetrahedrally coordinated Al center on a defective surface (Fig. 4a and S7†), corroborating that the CH4 activation on non-defective hydrated γ-Al2O3 (100) surfaces is kinetically less favorable than that on oxygen vacancy surfaces.
Thermal stability and surface activation of templates for ideal 3D minimum-surface graphenes
Thus, TG, IR, and DFT calculations demonstrate that the initial desorption of H2O from γ-ANPs without CH4 (Fig. 3) cannot trigger off the reaction, while the subsequent elimination of surface oxygens with CH4 (Fig. 1e and f) is essential for generating the active surfaces in CH4-CVD. The resultant Lewis acid-base pair at oxygen vacancies can make the formation of single-walled NPG kinetically feasible with a significantly lower activation energy as compared to a radical mechanism.24 Interestingly, further desorption of H2O from the γ-ANP surfaces by heating from 900 to 1000 °C resulted in slower reaction rates (Table S1†). This could be explained by the structural changes of γ-ANP: The specific surface area of γ-ANPs decreased from 158 m2 g−1 for pristine to 139 m2 g−1 upon treatment at 900 °C for 2 h, and eventually decreased to 124 m2 g−1 upon annealing at 1000 °C for 2 h. The structural reorganization during CH4-CVD was also supported by 27Al nuclear magnetic resonance (NMR), which showed an increase in octahedrally coordinated stable Al centers ([6]Al) induced by annealing during CH4-CVD (Fig. S9†), and XRD (Fig. S10†).
Such structural reconstruction hinders the synthesis of three-dimensionally and periodically arranged single-walled graphene materials, even with the use of structurally ordered templates such as mesoporous silica80 and zeolites.11 Therefore, precise control of oxygen vacancies as well as high thermal stability of templates will be essential for CH4-CVD reactions on various ANPs (Fig. S11†) and other oxides.20,23,72 Further surface engineering, including activation by gaseous reductants, may be helpful in lowering the operation temperature for more efficient CH4 activation and NPG synthesis in the future.
Conclusions
In summary, we have investigated the early-stage CH4 activation toward porous nanocarbon formation on γ-Al2O3 nanoparticles via reaction kinetics and surface analysis. We found that oxygen vacancies were formed on the surfaces of γ-Al2O3 nanoparticles upon their reaction with CH4 at temperatures higher than 800 °C. Carbon growth was promoted at the oxygen vacancies without the introduction of transition metal reaction centers. The initial dissociative adsorption of CH4 is the rate-limiting step because the overall rate of carbon growth is pseudo-first order for the CH4 partial pressure, and this is supported by DFT calculations. Surface Al at vacancy sites acts as a Lewis acid, whereas the adjacent surface oxygen acts as a Lewis base for dissociative adsorption to give surface-bound methyl and hydroxyl groups. This is followed by subsequent proton transfer to produce reactive surface-bound methylene species, leading to carbon growth. Carbon deposition from stable CH4 was faster on the surfaces of γ-ANPs with oxygen defects than on the deposited carbon films, and this makes it kinetically feasible to selectively form single-walled porous nanographene. Our work shows that precise surface engineering for introducing defects while enforcing the thermal stability of templates is crucial for accelerating CH4-CVD for better-quality NPG with fascinating features.15,16
Data availability
All data associated with this study are available in the main text or the ESI.† Further data will be available upon request to the authors.
Author contributions
M. Y., D. D. T., T. K. and K. Y. conceived and designed the project, and summarized all the data provided by co-authors. T. K. supervised the experiment part. S. G. contributed to thermogravimetry, mass spectrometry, and gas chromatography experiments. S. G. and H. N. contributed to the characterization of nanoporous carbon materials. M. Y., Y. G., M. T., and K. T. contributed to the in situ infrared spectroscopy in the presence of various gases. T. T. and T. Y. contributed to TEM and STEM of γ-ANPs. Q. Z. and A. A. conducted the quantum chemistry calculations. R. C. O. and D. D. T. supervised the quantum chemistry calculations and provided computational resources. M. Y., Q. Z., D. D. T., and K. Y. wrote the original draft, and contributed to visualization of the presented data. All authors contributed to review and editing.
Conflicts of interest
There are no conflicts to declare.
Acknowledgements
This work was supported by Grants-in-Aid (19K15281 and 19H00913) from JSPS, the Ebara-Hatakeyama Memorial Foundation, and Ensemble Grant for Early Career Researchers at Tohoku University. Q. Z. thanks China Scholarship Council for financial support. We thank Tohoku University Molecule and Material Synthesis Platform in the Nanotechnology Platform Project for NMR analysis operated by Mr S. Yoshida at Tohoku University, Japan. The authors acknowledge the kind support of Prof. Dr M. Kakihana and Prof. Dr H. Kato in performing Raman spectroscopy. The authors thank Sumitomo Chemical Co. Ltd for kindly supplying alumina nanoparticles. We thank Prof. Dr A. Muramatsu (Tohoku University), Dr T. Irisawa (Nagoya University), Dr R. Osuga (Tohoku University), and Prof. Dr J. N. Kondo (Tokyo Institute of Technology) for helpful discussions. K. Y. is grateful for the financial support from Building of Consortia for the Development of Human Resources in Science and Technology funded by MEXT and Core Research for Evolutional Science and Technology of the Japan Science and Technology Agency (JST CREST, Grant No. JPMJCR16P3). This research utilized Queen Mary's Apocrita HPC facility, supported by QMUL ITS Research. We are grateful to the UK Materials and Molecular Modeling Hub for computational resources, which were partially funded by EPSRC (EP/P020194/1 and EP/T022213/1).
References
- K. S. Novoselov, A. K. Geim, S. V. Morozov, D. Jiang, Y. Zhang, S. V. Dubonos, I. V. Grigorieva and A. A. Firsov, Science, 2004, 306, 666–669 CrossRef CAS PubMed.
- H. W. Kroto, J. R. Heath, S. C. O'Brien, R. F. Curl and R. E. Smalley, Nature, 1985, 318, 162–163 CrossRef CAS.
- S. Iijima and T. Ichihashi, Nature, 1993, 363, 603–605 CrossRef CAS.
- S. lijima, T. Ichihashi and Y. Ando, Nature, 1992, 356, 776–778 CrossRef.
- P. M. Ajayan and S. Iijima, Nature, 1992, 358, 23 CrossRef.
- A. L. Mackay and H. Terrones, Nature, 1991, 352, 762 CrossRef.
- X. Yang, F. Rominger and M. Mastalerz, Angew. Chem., Int. Ed., 2019, 58, 17577–17582 CrossRef CAS PubMed.
- K. Kato, K. Takaba, S. Maki-Yonekura, N. Mitoma, Y. Nakanishi, T. Nishihara, T. Hatakeyama, T. Kawada, Y. Hijikata, J. Pirillo, L. T. Scott, K. Yonekura, Y. Segawa and K. Itami, J. Am. Chem. Soc., 2021, 143, 5465–5469 CrossRef CAS PubMed.
- M. A. Medel, R. Tapia, V. Blanco, D. Miguel, S. P. Morcillo and A. G. Campaña, Angew. Chem., Int. Ed., 2021, 60, 6094–6100 CrossRef CAS PubMed.
- Y. Byun, L. S. Xie, P. Fritz, T. Ashirov, M. Dincă and A. Coskun, Angew. Chem., Int. Ed., 2020, 59, 15166–15170 CrossRef CAS PubMed.
- Z. Ma, T. Kyotani and A. Tomita, Chem. Commun., 2000, 2365–2366 RSC.
- Z. Ma, T. Kyotani, Z. Liu, O. Terasaki and A. Tomita, Chem. Mater., 2001, 13, 4413–4415 CrossRef CAS.
- T. Kyotani, T. Nagai, S. Inoue and A. Tomita, Chem. Mater., 1997, 9, 609–615 CrossRef CAS.
- H. Nishihara, T. Simura, S. Kobayashi, K. Nomura, R. Berenguer, M. Ito, M. Uchimura, H. Iden, K. Arihara, A. Ohma, Y. Hayasaka and T. Kyotani, Adv. Funct. Mater., 2016, 26, 6418–6427 CrossRef CAS.
- K. Nomura, H. Nishihara, M. Yamamoto, A. Gabe, M. Ito, M. Uchimura, Y. Nishina, H. Tanaka, M. T. Miyahara and T. Kyotani, Nat. Commun., 2019, 10, 2559 CrossRef PubMed.
- K. Nomura, H. Nishihara, N. Kobayashi, T. Asada and T. Kyotani, Energy Environ. Sci., 2019, 12, 1542–1549 RSC.
- M. Yamamoto, S. Goto, R. Tang, K. Nomura, Y. Hayasaka, Y. Yoshioka, M. Ito, M. Morooka, H. Nishihara and T. Kyotani, ACS Appl. Mater. Interfaces, 2021, 13, 38613–38622 CrossRef CAS PubMed.
- R. Tang, M. Yamamoto, K. Nomura, E. Morallón, D. Cazorla-Amorós, H. Nishihara and T. Kyotani, J. Power Sources, 2020, 457, 228042 CrossRef CAS.
- K. Kim, T. Lee, Y. Kwon, Y. Seo, J. Song, J. K. Park, H. Lee, J. Y. Park, H. Ihee, S. J. Cho and R. Ryoo, Nature, 2016, 535, 131–135 CrossRef CAS PubMed.
- D. S. Baek, K. A. Lee, J. Park, J. H. Kim, J. Lee, J. S. Lim, S. Y. Lee, T. J. Shin, H. Y. Jeong, J. S. Son, S. J. Kang, J. Y. Kim and S. H. Joo, Angew. Chem., Int. Ed., 2021, 60, 1441–1449 CrossRef CAS PubMed.
- M. Inagaki, S. Kobayashi, F. Kojin, N. Tanaka, T. Morishita and B. Tryba, Carbon, 2004, 42, 3153–3158 CrossRef CAS.
- T. Morishita, T. Tsumura, M. Toyoda, J. Przepiórski, A. W. Morawski, H. Konno and M. Inagaki, Carbon, 2010, 48, 2690–2707 CrossRef CAS.
- M. Inagaki, M. Toyoda, Y. Soneda, S. Tsujimura and T. Morishita, Carbon, 2016, 107, 448–473 CrossRef CAS.
- S. Sunahiro, K. Nomura, S. Goto, K. Kanamaru, R. Tang, M. Yamamoto, T. Yoshii, J. N. Kondo, Q. Zhao, A. G. Nabi, R. Crespo-Otero, D. Di Tommaso, T. Kyotani and H. Nishihara, J. Mater. Chem. A, 2021, 9, 14296–14308 RSC.
-
P. Euzen, P. Raybaud, X. Krokidis, H. Toulhoat, J.-L. Le Loarer, J.-P. Jolivet and C. Froidefond, Alumina, Wiley-VCH Verlag GmbH, Weinheim, 2002 Search PubMed.
- This is obviously determined by the atomic weight of carbon and the C
C bond length (1.42 Å) in the hexagons..
-
Y.-R. Luo, Handbook of Bond Dissociation Energies in Organic Compounds, CRC Press, London, 2003 Search PubMed.
- S. J. Blanksby and G. B. Ellison, Acc. Chem. Res., 2003, 36, 255–263 CrossRef CAS PubMed.
- J. Weitkamp, ChemCatChem, 2012, 4, 292–306 CrossRef CAS.
- Z. Liang, T. Li, M. Kim, A. Asthagiri and J. F. Weaver, Science, 2017, 356, 299–303 CrossRef CAS PubMed.
- G. Zichittella and J. Pérez-Ramírez, Chem. Soc. Rev., 2021, 50, 2984–3012 RSC.
- Y. Wang, P. Hu, J. Yang, Y.-A. Zhu and D. Chen, Chem. Soc. Rev., 2021, 50, 4299–4358 RSC.
- F. Schüth, Science, 2019, 363, 1282–1283 CrossRef PubMed.
- X. Zhang, C. Pei, X. Chang, S. Chen, R. Liu, Z.-J. Zhao, R. Mu and J. Gong, J. Am. Chem. Soc., 2020, 142, 11540–11549 CrossRef CAS PubMed.
- P. Schwach, X. Pan and X. Bao, Chem. Rev., 2017, 117, 8497–8520 CrossRef CAS PubMed.
- Z.-J. Zhao, C.-C. Chiu and J. Gong, Chem. Sci., 2015, 6, 4403–4425 RSC.
- D. Li, Y. Nakagawa and K. Tomishige, Appl. Catal., A, 2011, 408, 1–24 CrossRef CAS.
- J. H. Lunsford, Catal. Today, 2000, 63, 165–174 CrossRef CAS.
- A. M. Arinaga, M. C. Ziegelski and T. J. Marks, Angew. Chem., Int. Ed., 2021, 60, 10502–10515 CrossRef CAS PubMed.
- A. Sato, S. Ogo, K. Kamata, Y. Takeno, T. Yabe, T. Yamamoto, S. Matsumura, M. Hara and Y. Sekine, Chem. Commun., 2019, 55, 4019–4022 RSC.
- S. Arndt, G. Laugel, S. Levchenko, R. Horn, M. Baerns, M. Scheffler, R. Schlögl and R. Schomäcker, Catal. Rev., 2011, 53, 424–514 CrossRef CAS.
- X. Guo, G. Fang, G. Li, H. Ma, H. Fan, L. Yu, C. Ma, X. Wu, D. Deng, M. Wei, D. Tan, R. Si, S. Zhang, J. Li, L. Sun, Z. Tang, X. Pan and X. Bao, Science, 2014, 344, 616–619 CrossRef CAS PubMed.
- C. S. Cooper, R. J. Oldman and C. R. A. Catlow, Chem. Commun., 2015, 51, 5856–5859 RSC.
- A. J. Knorpp, A. B. Pinar, C. Baerlocher, L. B. McCusker, N. Casati, M. A. Newton, S. Checchia, J. Meyet, D. Palagin and J. A. van Bokhoven, Angew. Chem., Int. Ed., 2021, 60, 5854–5858 CrossRef CAS.
- J. Meyet, A. Ashuiev, G. Noh, M. A. Newton, D. Klose, K. Searles, A. P. van Bavel, A. D. Horton, G. Jeschke, J. A. van Bokhoven and C. Copéret, Angew. Chem., Int. Ed., 2021, 60, 16200–16207 CrossRef CAS PubMed.
- Z. Jin, L. Wang, E. Zuidema, K. Mondal, M. Zhang, J. Zhang, C. Wang, X. Meng, H. Yang, C. Mesters and F.-S. Xiao, Science, 2020, 367, 193–197 CrossRef CAS PubMed.
- M. A. Newton, A. J. Knorpp, V. L. Sushkevich, D. Palagin and J. A. van Bokhoven, Chem. Soc. Rev., 2020, 49, 1449–1486 RSC.
- M. H. Mahyuddin, T. Tanaka, Y. Shiota, A. Staykov and K. Yoshizawa, ACS Catal., 2018, 8, 1500–1509 CrossRef CAS.
- B. Ipek, M. J. Wulfers, H. Kim, F. Göltl, I. Hermans, J. P. Smith, K. S. Booksh, C. M. Brown and R. F. Lobo, ACS Catal., 2017, 7, 4291–4303 CrossRef CAS.
- X. Li, W. Cai, J. An, S. Kim, J. Nah, D. Yang, R. Piner, A. Velamakanni, I. Jung, E. Tutuc, S. K. Banerjee, L. Colombo and R. S. Ruoff, Science, 2009, 324, 1312–1314 CrossRef CAS PubMed.
- K. S. Kim, Y. Zhao, H. Jang, S. Y. Lee, J. M. Kim, K. S. Kim, J.-H. Ahn, P. Kim, J.-Y. Choi and B. H. Hong, Nature, 2009, 457, 706–710 CrossRef CAS PubMed.
- H. Chen, W. Zhu and Z. Zhang, Phys. Rev. Lett., 2010, 104, 186101 CrossRef PubMed.
- W. Zhang, P. Wu, Z. Li and J. Yang, J. Phys. Chem. C, 2011, 115, 17782–17787 CrossRef CAS.
- X. S. Li, C. W. Magnuson, A. Venugopal, J. H. An, J. W. Suk, B. Y. Han, M. Borysiak, W. W. Cai, A. Velamakanni, Y. W. Zhu, L. F. Fu, E. M. Vogel, E. Voelkl, L. Colombo and R. S. Ruoff, Nano Lett., 2010, 10, 4328–4334 CrossRef CAS PubMed.
- M. Losurdo, M. M. Giangregorio, P. Capezzuto and G. Bruno, Phys. Chem. Chem. Phys., 2011, 13, 20836–20843 RSC.
- R. Munoz and C. Gomez-Aleixandre, Chem. Vap. Deposition, 2013, 19, 297–322 CrossRef CAS.
- C. Arya, K. K. H. De Silva and M. Yoshimura, J. Mater. Sci.: Mater. Electron., 2020, 31, 21821–21831 CrossRef CAS.
- J. Kong, A. M. Cassell and H. Dai, Chem. Phys. Lett., 1998, 292, 567–574 CrossRef CAS.
- F. Yang, M. Wang, D. Zhang, J. Yang, M. Zheng and Y. Li, Chem. Rev., 2020, 120, 2693–2758 CrossRef CAS PubMed.
- A. M. Cassell, J. A. Raymakers, J. Kong and H. J. Dai, J. Phys. Chem. B, 1999, 103, 6484–6492 CrossRef CAS.
- V. M. Sivakumar, A. R. Mohamed, A. Z. Abdullah and S. P. Chai, J. Nanomater., 2010, 1–11 Search PubMed.
- A. A. Latimer, A. R. Kulkarni, H. Aljama, J. H. Montoya, J. S. Yoo, C. Tsai, F. Abild-Pedersen, F. Studt and J. K. Nørskov, Nat. Mater., 2017, 16, 225–229 CrossRef CAS PubMed.
- R. Venkateswaran, M. H. Back and G. Scacchi, Carbon, 1994, 32, 911–919 CrossRef.
- J.-P. Beaufils and Y. Barbaux, J. Chim. Phys., 1981, 78, 347–352 CrossRef CAS.
-
G. Socrates, Infrared and Raman Characteristic Group Frequencies: Tables and Charts, John Wiley & Sons, Ltd, New York, 3rd edn, 2004 Search PubMed.
- K. Khivantsev, N. R. Jaegers, J.-H. Kwak, J. Szanyi and L. Kovarik, Angew. Chem., Int. Ed., 2021, 60, 17522–17530 CrossRef CAS PubMed.
- J. G. Larson and W. K. Hall, J. Phys. Chem., 1965, 69, 3080–3089 CrossRef CAS.
- Z. Xie, Z. Li, P. Tang, Y. Song, Z. Zhao, L. Kong, X. Fan and X. Xiao, J. Catal., 2021, 397, 172–182 CrossRef CAS.
- U. Rodemerck, E. V. Kondratenko, T. Otroshchenko and D. Linke, Chem. Commun., 2016, 52, 12222–12225 RSC.
- J. P. Perdew, K. Burke and M. Ernzerhof, Phys. Rev. Lett., 1996, 77, 3865–3868 CrossRef CAS PubMed.
- S. Grimme, J. Antony, S. Ehrlich and H. Krieg, J. Chem. Phys., 2010, 132, 154104 CrossRef PubMed.
- J. Li, S. Zhou, J. Zhang, M. Schlangen, T. Weiske, D. Usharani, S. Shaik and H. Schwarz, J. Am. Chem. Soc., 2016, 138, 7973–7981 CrossRef CAS PubMed.
- R. Wischert, P. Laurent, C. Copéret, F. Delbecq and P. Sautet, J. Am. Chem. Soc., 2012, 134, 14430–14449 CrossRef CAS PubMed.
- R. Wischert, C. Copéret, F. Delbecq and P. Sautet, Angew. Chem., Int. Ed., 2011, 50, 3202–3205 CrossRef CAS PubMed.
- T. Matsushima and J. M. White, J. Catal., 1976, 44, 183–196 CrossRef CAS.
-
J. R. H. Ross, Heterogeneous Catalysis: Fundamentals
and Applications, Elsevier, Amsterdam, 2012 Search PubMed.
- M. Yu and D. R. Trinkle, J. Chem. Phys., 2011, 134, 064111 CrossRef PubMed.
- A. J. Page, S. Saha, H.-B. Li, S. Irle and K. Morokuma, J. Am. Chem. Soc., 2015, 137, 9281–9288 CrossRef CAS PubMed.
- T. Kreuger, W. P. M. van Swaaij, A. N. R. Bos and S. R. A. Kersten, Chem. Eng. J., 2022, 427, 130412 CrossRef CAS.
- C. T. Kresge, M. E. Leonowicz, W. J. Roth, J. C. Vartuli and J. S. Beck, Nature, 1992, 359, 710–712 CrossRef CAS.
Footnotes |
† Electronic supplementary information (ESI) available: Synthetic details and characterisation of materials. See DOI: 10.1039/d1sc06578e |
‡ Present address: RIKEN Center for Advanced Photonics, RIKEN, 2-1 Hirosawa, Wako, Saitama 351-0198, Japan, E-mail: E-mail: kaoru.yamazaki@riken.jp |
|
This journal is © The Royal Society of Chemistry 2022 |
Click here to see how this site uses Cookies. View our privacy policy here.