DOI:
10.1039/D1SC07271D
(Edge Article)
Chem. Sci., 2022,
13, 4801-4812
Stereodivergent synthesis of enantioenriched azepino[3,4,5-cd]-indoles via cooperative Cu/Ir-catalyzed asymmetric allylic alkylation and intramolecular Friedel–Crafts reaction†
Received
31st December 2021
, Accepted 27th March 2022
First published on 30th March 2022
Abstract
The development of enantioselective annulation reactions using readily available substrates for the construction of structurally and stereochemically diverse heterocycles is a compelling topic in diversity-oriented synthesis. Herein, we report efficient catalytic asymmetric formal 1,3-dipolar (3 + 4) cycloadditions of azomethine ylides with 4-indolyl allylic carbonates for the construction of azepino[3,4,5-cd]-indoles fused with a challenging seven-membered N-heterocycle, a frequently occurring tricyclic indole scaffold in bioactive compounds and pharmaceuticals. Through cooperative Cu/Ir-catalyzed asymmetric allylic alkylation followed by intramolecular Friedel–Crafts reaction, an array of azepino[3,4,5-cd]-indoles were obtained in good yields with excellent diastereo-/enantioselective control. More importantly, the full stereodivergence of this transformation was established via synergistic catalysis followed by acid-promoted epimerization, and up to eight stereoisomers of the cycloadducts bearing three stereogenic centers could be predictably achieved from the same set of starting materials for the first time. Quantum mechanical computations established a plausible mechanism for the synergistic Cu/Ir catalysis to stereodivergently introduce two vicinal stereocenters whose stereochemical information is remotely delivered across the fused azepine ring to control the third chiral center. Epimerization of the last center involves protonation-enabled reversal of the thermodynamically controlled relative configuration.
Introduction
Transforming simple precursors into a library of natural product-like or drug-like scaffolds with skeletal and stereochemical diversity remains an important objective as well as a huge challenge in diversity-oriented synthesis (DOS).1 As a straightforward methodology to serve such endeavors, the so-called reagent-based or branching DOS approach which involves the application of different reaction partners/conditions to access distinct molecular architectures from a common substrate has received considerable attention.2 For example, the diversity-oriented synthesis of enantioenriched N-heterocycles using in situ generated azomethine ylides (AMYs) from readily available aldimine esters as key building blocks has been intensively studied for decades (Scheme 1a).3,4 Specifically, transition-metal catalyzed asymmetric 1,3-dipolar (3 + 2) cycloadditions of AMYs with electron-deficient alkenes are known to be one of the most important tools for the construction of five-membered chiral pyrrolidines.5 Recent developments in enantioselective higher order (3 + 3) cycloaddition further extended the synthetic applicability of AMYs to approach six-membered N-heterocycles including piperidines and 1,4,5-triazines.6 In contrast, assembling important skeletons other than five-/six-membered rings, for instance, seven-membered N-heterocycles, via 1,3-dipolar (3 + 4) cycloaddition of AMYs is extremely difficult owing to the disfavored entropic factors and transannular interactions7 and the challenge in stereocontrol of multiple stereogenic centers.4 In 2014, we reported an Et3N-catalyzed formal (3 + 4) annulation of aldimine esters with methyl coumalate for the preparation of functionalized racemic azepine derivatives.8a However, the catalytic asymmetric 1,3-dipolar (3 + 4) cycloaddition of AMYs, especially for the construction of seven-membered N-heterocycles featuring stereodivergence, remains elusive and a formidable task.
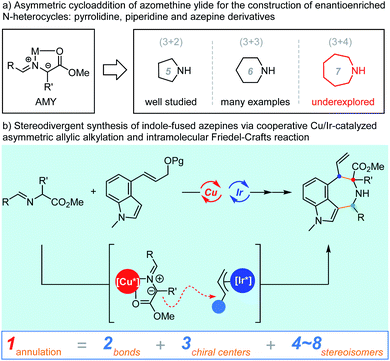 |
| Scheme 1 Synthetic approaches to chiral azepino[3,4,5-cd]indoles. | |
On the other hand, the stereochemical diversity in cycloaddition reactions represents another challenging subject in organic synthesis. For bioactive compounds and pharmaceutical molecules bearing multiple stereogenic centers, both absolute and relative configurations can greatly affect their biological activities.9 Therefore, the development of reliable and facile access to all stereoisomers of a relevant scaffold from the same starting materials would be highly significant. In recent years, the strategy of synergistic catalysis has attracted extensive attention in the area of stereodivergent synthesis,10,11 which offers access to all four stereoisomeric compounds bearing two stereogenic centers through judicious permutation of two individual chiral catalysts, which simultaneously activate two different reaction partners, respectively.12,13 Recently, such a strategy has been successfully applied in Cu/Ir-catalyzed stereodivergent 1,3-dipolar (3 + 3) cycloaddition of AMYs with 2-indolyl allyl carbonates, furnishing enantioenriched tetrahydro-γ-carbolines bearing three stereogenic centers with the stereoselective formation of only four isomers among the total eight stereoisomers.14 Despite these achievements, to the best of our knowledge, catalytic stereodivergent 1,3-dipolar cycloaddition of AMYs for the precise construction of all eight stereoisomers of N-heterocycles incorporating three stereogenic centers is unprecedented. We anticipated that the ambiphilic π-allyl-iridium species in situ generated from 4-indolyl allylic carbonates may serve as a unique 4-atom unit in formal 1,3-dipolar (3 + 4) cycloaddition of azomethine ylides,15 providing a novel route to azepino[3,4,5-cd] indole derivatives through asymmetric allylic alkylation16 followed by intramolecular Friedel–Crafts reaction17 (Scheme 1b). The chiral azepino[3,4,5-cd] indole framework is present in numerous bioactive compounds and pharmaceuticals,18,19 while very few examples have been documented for its enantioselective preparation so far.20 Herein, we report the development of cooperative Cu/Ir-catalyzed formal 1,3-dipolar (3 + 4) cycloaddition of azomethine ylides to afford azepino[3,4,5-cd]-indole derivatives bearing three stereogenic centers with high diastereo- and enantiocontrol. Importantly, combining synergistic catalysis and late-stage epimerization allows the precise construction of all eight stereoisomers of the 3,4-fused tricyclic indoles, thus making the current transformation fully stereodivergent.
Results and discussion
Condition optimization
To examine the feasibility of the designed azepino[3,4,5-cd]-indole synthesis, we first explored the reaction using alanine derived aldimine ester 1a (serving as an AMY precursor) and (E)-4-indolyl allyl carbonate 2a (serving as a π-allyl-Ir precursor) as the model substrates, and K2CO3 as the base in dichloroethane at room temperature. With the dual [Cu(I)/(S,Sp)-L1 + Ir(I)/(R,Ra)-L4] catalyst combination utilized in our previous stereodivergent 1,3-dipolar (3 + 3) cycloaddition of AMYs with (E)-2-indolyl allyl carbonates,14 unfortunately, no desired (3 + 4) cycloadduct 3a was observed, while isolation of the reaction mixture indicated that the reaction was interrupted in the first coupling step. We envisioned that the addition of a stronger Lewis acid might be able to activate the imine moiety of the allylation intermediate and thus promote the subsequent intramolecular Friedel–Crafts reaction.17 To our delight, 0.5 equivalents of Zn(OTf)2 significantly accelerated the desired cyclization, furnishing the cycloadduct azepino[3,4,5-cd] indole 6,9-cis-3a in 52% yield with unsatisfactory diastereoselectivity (3
:
1 dr; only 6,9-cis-isomers were formed due to the subsequent cyclization step being stereospecific) albeit with excellent enantioselectivity (99% ee for both isomers) (Table 1, entry 1). To improve the diastereoselectivity control, chiral phosphoramidite ligands21a (L5 and L6) commonly used in Ir-catalyzed asymmetric allylic alkylation were then evaluated for this reaction. With the combination of [Cu(I)/(S,Sp)-L1 + Ir(I)/(Sa,S,S)-L5] as the dual catalysts, the sequential reaction proceeded smoothly to afford the desired cycloadduct in 55% yield with high diastereoselectivity (18
:
1 dr) and excellent enantioselectivity (99% ee) (Table 1, entry 2). Lower diastereoselectivity was observed when using bulky ortho-methoxy substituted phosphoramidite L6 as the chiral ligand for the iridium complex (entry 3). Further screening of chiral Phosferrox ligands21b for the copper complex ((S,Sp)-L1–L3) and iridium complex ((Sa,S,S)-L5 and (Sa,S,S)-L6) identified the combination of [Cu(I)/(S,Sp)-L1 + Ir(I)/(Sa,S,S)-L5] as optimal in terms of diastereo-/enantioselectivity (entries 3–5). With the catalytic system established, we then examined various additives to improve the yield. Among the tested Lewis/Brønsted acids, zinc oxide22 led to no reaction at all, while metal triflates such as Sc(OTf)3, Yb(OTf)3 and Sn(OTf)2 afforded moderate yields with 9
:
1 to 17
:
1 dr (entries 6–9). Other promoters including Et2O·BF3 and (PhSO2)2NH gave no better results either (entries 10 and 11). Carefully monitoring the reaction by NMR experiments revealed that the unsatisfactory yield was mainly caused by the decomposition of an imine-containing allylation intermediate in the cyclization step, and no other byproduct was observed in this transformation. Accordingly, we examined the feasibility of adding an external aldehyde to minimize the undesired imine-decomposition with yield improvement although it is a limitation of this method. Gratifyingly, the cyclization proceeded smoothly in the presence of 0.5 equivalents of Zn(OTf)2 and two equivalents of p-chlorobenzaldehyde, delivering 3a in 67% yield with maintained diastereoselectivity and enantioselectivity (entry 13). (Z)-4-indolyl allyl carbonate 2a′ was not a viable reactant partner in this transformation due to the less efficiency of the allylation step. Additionally, both of copper and iridium catalysts are critical for the efficiency of the initial coupling reaction. Without either of these two metal complexes, no reaction occurred under otherwise identical reaction conditions (entries 14 and 15).
Table 1 Optimization of reaction conditionsa
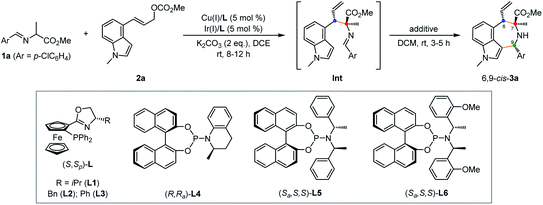
|
Entry |
L for Cu(I) |
L for Ir(I) |
Additive (mol%) |
drb |
Yield (%)c |
ee (%)d |
All reactions were carried out with 0.30 mmol 1a and 0.20 mmol 2a in 2 mL of DCE. Cu(I) = Cu(MeCN)4BF4. Ir(I) = [Ir(cod)Cl]2.
dr was determined by the 1H NMR of the crude reaction mixture.
Isolated yields of the overall two steps.
ee was determined by chiral HPLC analysis.
2 equiv. of 4-chlorobenzaldehyde was added.
|
1 |
(S,Sp)-L1 |
(R,Ra)-L4 |
Zn(OTf)2 (50) |
3 : 1 |
52 |
99 |
2 |
(S,Sp)-L1 |
(Sa,S,S)-L5 |
Zn(OTf)2 (50) |
18 : 1 |
55 |
99 |
3 |
(S,Sp)-L1 |
(Sa,S,S)-L6 |
Zn(OTf)2 (50) |
12 : 1 |
58 |
99 |
4 |
(S,Sp)-L2 |
(Sa,S,S)-L5 |
Zn(OTf)2 (50) |
16 : 1 |
60 |
99 |
5 |
(S,Sp)-L3 |
(Sa,S,S)-L5 |
Zn(OTf)2 (50) |
14 : 1 |
54 |
99 |
6 |
(S,Sp)-L1 |
(Sa,S,S)-L5 |
ZnO (50) |
— |
— |
— |
7 |
(S,Sp)-L1 |
(Sa,S,S)-L5 |
Sc(OTf)3 (50) |
15 : 1 |
45 |
99 |
8 |
(S,Sp)-L1 |
(Sa,S,S)-L5 |
Yb(OTf)3 (50) |
17 : 1 |
55 |
99 |
9 |
(S,Sp)-L1 |
(Sa,S,S)-L5 |
Sn(OTf)2 (50) |
9 : 1 |
35 |
99 |
10 |
(S,Sp)-L1 |
(Sa,S,S)-L5 |
(PhSO2)2NH (50) |
14 : 1 |
57 |
99 |
11 |
(S,Sp)-L1 |
(Sa,S,S)-L5 |
Et2O·BF3 (20) |
4 : 1 |
40 |
99 |
12e |
(S,Sp)-L1 |
(Sa,S,S)-L5 |
Yb(OTf)3 (50) |
16 : 1 |
65 |
99 |
13e |
(S,Sp)-L1 |
(Sa,S,S)-L5 |
Zn(OTf)2 (50) |
18 : 1 |
67 |
99 |
14 |
(S,Sp)-L1 |
— |
Zn(OTf)2 (50) |
— |
— |
— |
15 |
— |
(Sa,S,S)-L5 |
Zn(OTf)2 (50) |
— |
— |
— |
Substrate scope
With the optimal reaction conditions in hand, we set out to explore the substrate scope of azomethine ylide precursors in this cycloaddition. As illustrated in Table 2, an array of aromatic aldehyde derived imine esters 1 underwent efficient asymmetric allylic alkylation/intramolecular Friedel–Crafts reaction with 2a, producing the corresponding azepino[3,4,5-cd]-indoles 3a–m in moderate to good yields with high diastereoselectivities and excellent enantioselectivities (Table 2, entries 1–13). The substituents on the aryl ring of aldimine esters 1 with different electronic properties (electron-withdrawing, -neutral and -donating) in different positions (para-, meta- and ortho-) displayed a negligible influence on the stereochemical outcome. Heterocyclic 2-thienyl and 2-furyl aldimine esters exhibited comparable levels of reactivity, giving the desired cycloadducts 3n and 3o in synthetically acceptable yields with high diastereoselectivities and 99% ee (entries 14 and 15). In addition, the less reactive aliphatic aldehyde derived imine ester was tolerated well and the corresponding cycloadduct 3p was isolated in moderate yield with excellent stereoselective control (entry 16). Moreover, the reaction of 2-amino-γ-butyrolactone derived aldimine ester 1q with 2a proceeded smoothly, affording the desired spiro heterocyclic azepino[3,4,5-cd] indole 3q in 62% yield with good dr and 99% ee (entry 17). Next, azomethine ylides derived from various natural and unnatural amino acid esters were subjected to this reaction. Aldimine esters containing alkyl, allyl, ether or ester functional groups at the α-position all proved to be viable, furnishing the desired cycloadducts 3r–v in acceptable yields with 19
:
1 to 20
:
1 dr and 99% ee (entries 18–22). No reaction occurred when an α-phenyl substituted aldimine ester was employed probably due to the unfavourable steric hindrance. The current protocol could also be readily extended to a glycine derived azomethine ylide, which worked as a suitable partner to deliver the corresponding product 3w with good diastereoselectivity (9
:
1 dr) and excellent enantioselectivity (99% ee, entry 23). Additionally, comparable diastereoselectivity and enantioselectivity were maintained in a 1.0 mmol (2a) scale reaction (entry 24).
Table 2 Substrate scope of aldimine estersa
Then, we turned our attention to examine the scope of 4-indolyl allyl carbonates and the results are summarized in Table 3. We were pleased to observe that indole moieties bearing halogen, phenyl, vinyl or cyclopropyl substituents at the 5-, 6- or 7-position were compatible with this reaction, delivering the highly functionalized azepino[3,4,5-cd] indoles 3x-D in moderate to good yields, good to high diastereoselectivities and excellent enantioselectivities (Table 3, entries 1–7). N-Allyl- or N-benzyl-protected 4-indolyl allyl carbonates were well tolerated in this transformation to afford the corresponding cycloadducts 3E and 3F, respectively, in good yields with high diastereoselectivities and excellent enantioselectivities (entries 8 and 9). N-unprotected 4-indolyl allyl carbonate also underwent the current formal 1,3-dipolar [3 + 4] cycloaddition with 1a, providing the desired product 3G in 66% yield with 11
:
1 dr and 99% ee (entry 10).
Table 3 Substrate scope of 4-indolyl allylic carbonatesa
Next, we examined the feasibility of the stereodivergent access to the stereoisomers of azepino[3,4,5-cd] indole derivatives. Six aldimine esters (1a, 1n and 1t–w) were chosen as the representative substrates to react with 4-indolyl allyl carbonate 2a using different sets of Cu/Ir catalyst combinations. As shown in Table 4, in each case, with the pairwise combination of the dual system from the complete four sets of catalyst permutations Cu(I)/(R,Rp)-L1 or Cu(I)/(S,Sp)-L1 with Ir(I)/(Ra,R,R)-L5 or Ir(I)/(Sa,S,S)-L5, four 6,9-cis-isomers of all eight stereoisomers, that is, (6S,7S,9R)-, (6R,7S,9S)-, (6R,7R,9S)-, and (6S,7R,9R)-3, were precisely constructed in good yields with high diastereoselectivities and excellent enantioselectivities.
Table 4 Representative examples of stereodivergencea
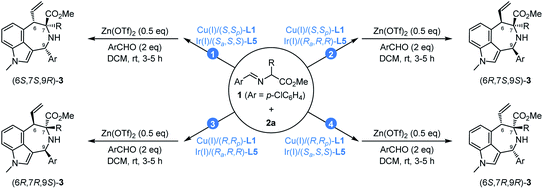
|
entry |
Ar |
R
|
Cooperative copper/iridium catalysts |
Cu(I)/(S,Sp)-L1 |
Cu(I)/(S,Sp)-L1 |
Cu(I)/(R,Rp)-L1 |
Cu(I)/(R,Rp)-L1 |
Ir/(Sa,S,S)-L5 |
Ir/(Ra,R,R)-L5 |
Ir/(Ra,R,R)-L5 |
Ir/(Sa,S,S)-L5 |
All reactions were carried out with 0.30 mmol 1 and 0.20 mmol 2a in 2 mL of DCE within 12–18 h for allylation step. dr was determined by the 1H NMR of the crude reaction mixture. Isolated yields of the overall two steps. ee was determined by chiral HPLC analysis.
Discrepancy in configuration is caused by the sequence rule.
|
1 |
p-ClC6H4 |
Me |
(6S,7S,9R)-3a, 67% yield |
(6R,7S,9S)-3a, 63% yield |
(6R,7R,9S)-3a, 62% yield |
(6S,7R,9R)-3a, 66% yield |
18 : 1 dr, 99% ee |
20 : 1 dr, 99% ee |
17 : 1 dr, 99% ee |
20 : 1 dr, 99% ee |
2b |
2-thienyl |
Me |
(6S,7S,9S)-3n, 58% yield |
(6R,7S,9R)-3n, 45% yield |
(6R,7R,9R)-3n, 60% yield |
(6S,7R,9S)-3n, 52% yield |
14 : 1 dr, 99% ee |
10 : 1 dr, 99% ee |
14 : 1 dr, 99% ee |
10 : 1 dr, 99% ee |
3 |
p-ClC6H4 |
CH2CH CH2 |
(6S,7S,9R)-3t, 66% yield |
(6R,7S,9S)-3t, 60% yield |
(6R,7R,9S)-3t, 60% yield |
(6S,7R,9R)-3t, 62% yield |
20 : 1 dr, 99% ee |
20 : 1 dr, 99% ee |
19 : 1 dr, 99% ee |
20 : 1 dr, 99% ee |
4b |
p-ClC6H4 |
CH2Ot-Bu |
(6S,7R,9R)-3u, 65% yield |
(6R,7R,9S)-3u, 60% yield |
(6R,7S,9S)-3u, 61% yield |
(6S,7S,9R)-3u, 62% yield |
20 : 1 dr, 99% ee |
20 : 1 dr, 99% ee |
20 : 1 dr, 99% ee |
19 : 1 dr, 99% ee |
5 |
p-ClC6H4 |
(CH2)2CO2Me |
(6S,7S,9R)-3v, 58% yield |
(6R,7S,9S)-3v, 54% yield |
(6R,7R,9S)-3v, 52% yield |
(6S,7R,9R)-3v, 55% yield |
20 : 1 dr, 99% ee |
20 : 1 dr, 99% ee |
19 : 1 dr, 99% ee |
18 : 1 dr, 97% ee |
6 |
p-ClC6H4 |
H |
(6S,7S,9R)-3w, 54% yield |
(6R,7S,9S)-3w, 52% yield |
(6R,7R,9S)-3w, 55% yield |
(6S,7R,9R)-3w, 56% yield |
9 : 1 dr, 99% ee |
10 : 1 dr, 99% ee |
10 : 1 dr, 99% ee |
10 : 1 dr, 99% ee |
Scale-up experiments and synthetic application
To demonstrate the practicality of this methodology, a scale-up synthesis of azepino[3,4,5-cd]-indole with catalyst combination [Cu(I)/(S,Sp)-L1 + Ir(I)/(Ra,R,R)-L5] was performed under the optimal reaction conditions. To our delight, (6R,7S,9S)-3a could be obtained in good and comparable yield without loss of diastereoselective and enantioselective control (Scheme 2). Then, azepino[3,4,5-cd]-indole (6R,7S,9S)-3a obtained herein readily underwent different synthetic transformations. Palladium(II)-catalyzed cyclopropanation of (6R,7S,9S)-3a with diazomethane afforded the corresponding product 4 in a good yield with maintained diastereoselectivity and enantioselectivity. Iridium(I)-catalyzed hydroboration of the terminal alkene in (6R,7S,9S)-3a delivered the anti-Markovnikov product 5 in 89% yield without loss of enantiopurity. Ester reduction with NaBH4 provided the corresponding compound 6 in 75% yield with the retention of diastereoselectivity and enantioselectivity.
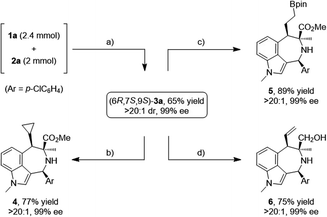 |
| Scheme 2 Scale-up experiments and synthetic transformations. | |
Exploration of stereodivergent synthesis of all eight stereoisomers
Based on the experimental results and X-ray diffraction analysis of the achieved cycloadducts (vide infra),23 we postulated that the stereochemical outcome of this formal 1,3-dioplar (3 + 4) cycloaddition originated from a cooperative Cu/Ir-catalyzed stereodivergent asymmetric allylic alkylation followed by a stereospecific intramolecular Friedel–Crafts reaction, thus only four 6,9-cis-isomers of all eight stereoisomers could be prepared with the distinct permutations of the four sets of chiral Cu and chiral Ir catalysts. C9-epimerization of the 6,9-cis-isomers would be an ideal pathway for expedient access to the other four stereoisomers (6,9-trans-isomers). All C9-epimerization attempts failed under basic conditions.24 However, to our delight, C9-epimerization of the 6,9-cis-cycloadducts could be readily realized through the TFA-promoted ring-opening-cyclization reaction. In the presence of 2 equivalents of trifluoroacetic acid (TFA) in dichloromethane, 6,9-cis-isomers (6S,7S,9R)-3a and (6R,7R,9S)-3a could be efficiently converted into the corresponding 6,9-trans-isomers (6S,7S,9S)-3a and (6R,7R,9R)-3a, respectively, without any erosion of enantioselectivity (Scheme 3A). Additionally, (6S,7S,9S)-3a and (6R,7R,9R)-3a could be directly achieved through a one-pot allylation/cyclization/epimerization process when using TFA as the cyclization promoter (see the ESI† for details). Less efficient C9-epimerization was observed for the other two 6,9-cis-isomers (6R,7S,9S)-3a and (6S,7R,9R)-3a, and moderate yields of the corresponding enantioenriched 6,9-trans-isomers (6R,7S,9R)-3a and (6S,7R,9S)-3a were obtained with the 6,9-cis-isomers being recovered. Nevertheless, the chemical yields of the two 6,9-trans-stereoisomers could be readily improved through iteration of TFA-promoted C9-epimerization (Scheme 3B). The absolute configurations of the cycloadduct (6S,7S,9R)-3a and the two epimerized (6R,7R,9R)-3a and (6R,7S,9R)-3a were unequivocally determined by X-ray diffraction analysis.23
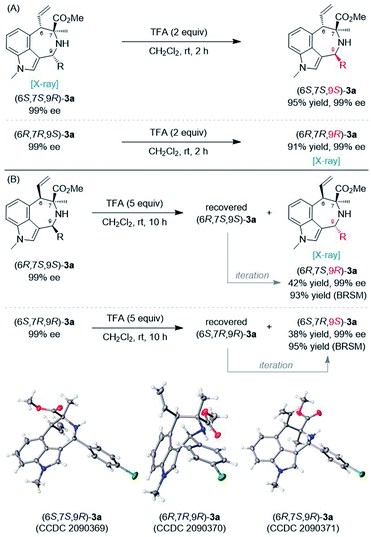 |
| Scheme 3 TFA-promoted C9-epimerization of 6,9-cis-3a to access another four 6,9-trans-3a stereoisomers. | |
Computational mechanistic studies
Using quantum mechanical computations,25 we investigated the mechanism by which the azepino[3,4,5-cd]-indole products are stereoselectively constructed with generation of three chiral centers. The M06-L functional26 was used on account of the balanced performance for main-group and transition-metal chemistry and the ability to describe non-covalent interactions. Notably, the mechanism involves an array of stereocontrolling steps that dictate the absolute configurations at the C6/C7/C9 sites, respectively. Initial stereochemical information is introduced by synergistic Cu/Ir catalysis at the C6/C7 positions in the enantio- and diastereodivergent formation of the C6–C7 bond to rapidly build up molecular complexity. This dual-catalytic process will be studied first.27,28 The ensuing steps feature achiral reagent promoted cyclization and epimerization with effective delivery of chirality, which will be explored later.
a. Synergistic Cu/Ir catalysis.
In light of the stereospecific anti-attack on the allyl-iridium in the C6–C7 bond-forming step, we reasoned that the absolute configuration at C6 is determined by the stereochemical structure of π-allyl-iridium species, which is formed from the oxidative addition of 4-indolyl allyl carbonate 2a with Ir(I) (see Fig. 1A for the schematic description and Fig. 1B for the computations). We considered an activated form of the iridium catalyst IM1, which contains a metallacyclic structure with the ligations of L5 and COD.29 In the presence of (S,S,S)-L5, the competition of TS1avs.TS1b exhibits a large barrier difference of 2.9 kcal mol−1, favoring the formation of π-allyl-iridium IM3a. Meanwhile, IM3a is 2.9 kcal mol−1 lower in free energy than the diastereomeric IM3b. The kinetic and thermodynamic preference for IM3a led us to infer that it represents the predominant allyl-iridium whereby the C6 stereocenter can be furnished through the anti-displacement-type coupling with the nucleophilic Cu-AMY species IM4. The computations allow us to correctly predict a 6S stereocenter when applying (S,S,S)-L5 (and 6R with (R,R,R)-L5).
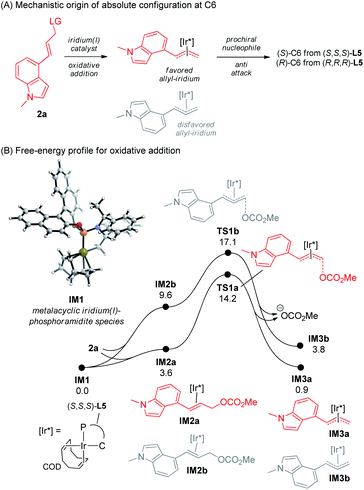 |
| Fig. 1 Mechanistic origin of C6 stereoselectivity. Oxidative addition of 2a with the activated iridium catalyst. Free energies are provided in kcal mol−1.30 | |
In attempts to understand the stereodiscrimination of allyl-iridium governing C6 stereochemistry, we explored how the chiral Ir catalyst creates the observed energetic differences in TS1 and IM3. For the former, we notice, as visualized in Fig. 2A, that the steric bulk of COD (blue, front) is circumvented by the indolyl unit (yellow, back) in TS1a, while the same structures result in a steric impediment to the catalyst–substrate interactions in TS1b. Such features have been carried over to allyl-iridium IM3a/IM3b to cause stronger steric repulsions in the latter, which is described in Fig. 2B. The comparisons reflect the effectiveness of the Ir catalyst in selectively exposing one π-allyl diastereoface for the following transformations.
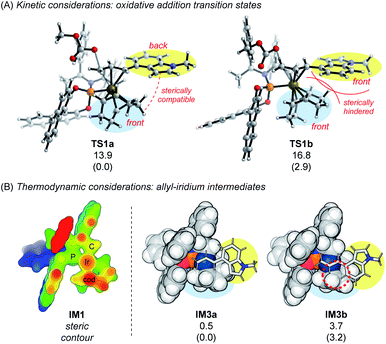 |
| Fig. 2 Steric analysis for C6 stereoselectivity. Free energies are provided in kcal mol−1, with relative values in parentheses.31,32 | |
With an understanding of the generation of allyl-iridium, we investigated the coupling of the electrophilic allyl-iridium IM3a with the nucleophilic Cu-AMY complex IM4 to rationalize the stereoselectivity for the C7 center.27,28 The mechanistic origin of the C7-stereoselection lies in a diastereofacial preference of the AMY unit (Fig. 3A). Despite simultaneous engagement of two chiral catalysts in the coupling step, Table 4 indicates that the stereochemical outcome at C7 depends exclusively on L1. To reveal the underlying mode of stereoinduction, the combinations of (S,S)-L1 with (S,S,S)-L5 (TS2/TS2') and (R,R,R)-L5 (TS3/TS3′) were studied. Regarding the Ir catalyst, it follows from previous analysis that (S,S,S)-L5 and (R,R,R)-L5 will selectively give IM3a and its enantiomer ent-IM3a, respectively. For the Cu catalyst, evaluation of the two possible diastereomers of the Cu-AMY complex discloses smaller steric repulsions when the aryl group is arranged on the unoccupied side of L1, leading to a free-energy difference of 2.6 kcal mol−1 favoring the participation of IM4, the main form of Cu-AMY, in C6–C7 coupling (see Fig. S1 in the ESI† for details). On the basis of these considerations, the transition states for the interception of allyl-iridium IM3a/ent-IM3a by Cu-AMY species IM4 were calculated (Fig. 3B). For both catalyst combinations, stereoregulation by (S,S)-L1 makes the si face of the AMY moiety more favorable than the re face, regardless of the absolute configuration of L5, thus generating a 7S stereocenter. The notable barrier differences (4.7 and 6.4 kcal mol−1) contribute to the excellent ee, and the correspondence between (S,S)-L1 and 7S agrees with experiments.
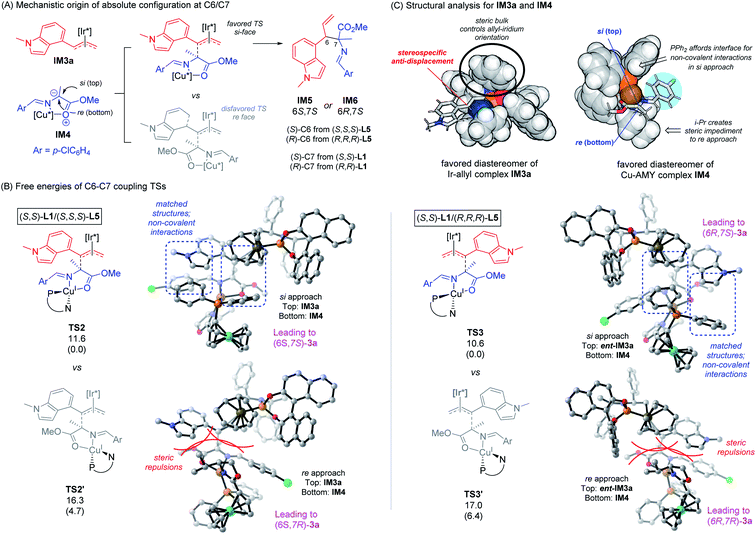 |
| Fig. 3 Mechanistic origin of C7 stereoselectivity. Coupling between in situ-generated Cu-AMY species and π-allyl-iridium species. IM3a/IM4 are used as energy zero. Free energies are provided in kcal mol−1. | |
The three-dimensional transition-state structures on display in Fig. 3B indicate a shared mode of stereoinduction for the two catalyst combinations. The si-TSs TS2/TS3 not only manifest good structural matching between the catalyst-bound activated substrates but also involve C–H⋯π interactions of COD and the indolyl group with the –PPh2 phosphino moiety of L1 (see Fig. S2 in the ESI† for a visual analysis of non-covalent interactions). The re-TSs, in contrast, are disfavored because an i-Pr group on L1 presents a steric obstacle to the approaching allyl-iridium. As supported by a further structural analysis in Fig. 3C, while the relative orientations of the two reactive species are controlled by the steric bulk of L5, it is the stereocontrol elements of L1 that act as the main origin of AMY-diastereofacial selectivity. The L1-dependence of the resultant C7-stereoselectivity can be interpreted accordingly.
b. Zn(II)-promoted intramolecular Friedel–Crafts reaction.
Having rationalized the generation of C6/C7 stereocenters by Cu/Ir synergistic catalysis, we sought to establish the mechanism of chirality transfer from existing stereocenters to C9 when treated by an achiral Lewis acid Zn(OTf)2 (Fig. 4A). Notably, a strong stereochemical correlation between the C6 and C9 centers can be inferred from Table 4, i.e., the formation of 9R/9S is apparently dictated by the initially introduced 6S/6R respectively to yield the 6,9-cis-isomers, while the configuration of C7 appears to be irrelevant. To gain a mechanistic understanding, we hypothesize that the coordination of the Zn(II) Lewis acid on the allylation intermediate IM5/IM6 can activate the electrophilic C
N bond and thus promote the cyclization to construct the desired azepino[3,4,5-cd]-indole skeleton.33 Possible cyclization TSs were calculated and are presented in Fig. 4B. Interestingly, the assumption that the selectivity is kinetically controlled would lead to an incorrect stereochemical prediction for the cyclization of IM5, since TS4′ (yielding (6S,7S,9S)-3a) is lower in energy than TS4 (yielding the observed (6S,7S,9R)-3a). Given the low barriers of TS4/TS4′, we speculated for IM5 that a thermodynamic control of C9-stereoselectivity might be operative. The postulate is supported by the lower energy of (6S,7S,9R)-3a than the C9-epimer, the difference being 1.3 kcal mol−1. With regard to IM6, (6R,7S,9S)-3a is calculated to be both the kinetic (TS5vs.TS5′) and the thermodynamic product, thus its formation can be unambiguously predicted from the computations.
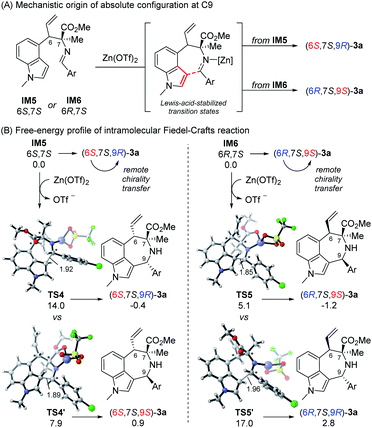 |
| Fig. 4 Mechanistic origin of C9 stereoselectivity in Lewis-acid-promoted intramolecular Friedel–Crafts reaction. Free energies are provided in kcal mol−1 and distances in Å. | |
c. TFA-promoted C9-Epimerization.
Lastly, we turn to elucidate the mechanism of TFA-promoted C9-epimerization. We have found through studying Lewis-acid-enabled intramolecular Friedel–Crafts reaction that the combinations of 6S,9R and 6R,9S configurations result in higher thermodynamic stabilities than 6S,9S and 6R,9R, respectively, in the ring system. We thus investigated the driving force behind the C9-epimerization. For a possible explanation, the strong Brønsted acid TFA might have protonated 3a to reverse the epimerization thermodynamics and thus permit the transformation. Indeed, it can be seen from Fig. 5 that the C9-epimerization of the protonated (6S,7S,9R)-3a-H+ is exergonic by −2.6 kcal mol−1, suggesting adequate spontaneity. This is in contrast to the stability of (6S,7S,9R)-3a (see left in Fig. 4B) and can be ascribed to an intramolecular N–H⋯O hydrogen bond in the epimerized (6S,7S,9S)-3a-H+ (see the 3D structures in Fig. 5). On the other hand, the free-energy change for the C9-epimerization of (6R,7S,9S)-3a-H+ is only −0.1 kcal mol−1, and equilibration with its C9-epimer is expected given sufficient time. The lack of driving force is partly because of the higher energy of the parent (6R,7S,9R)-3a which is 4.0 kcal mol−1 less stable than the epimeric (6R,7S,9S)-3a (see right in Fig. 4B). Upon comparing the predicted behaviors of 3a-H+ with the experimental results in Scheme 3, the obvious difference in yields and the amount of TFA can be very well explained, which in turn validates the computational models.
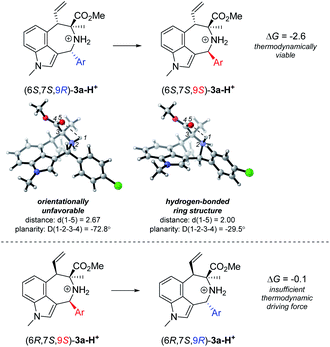 |
| Fig. 5 Thermodynamics of TFA-promoted C9-epimerization. Free energies are in kcal mol−1 and distances in Å. | |
With the thermodynamics established, we calculated the free-energy profile in more detail to further our understanding of the mechanistic role of TFA. We envisioned that the acidic conditions might render 3a prone to C9-stereoablation through a ring-opening C–N bond cleavage, followed by an aza-Michael reaction that re-constructs the ring with an opposite absolute configuration at the C9 center to yield the epimer. The proposed isomerization mechanism of (6S,7S,9R)-3a is shown in Fig. 6 (a similar profile is established for (6R,7S,9S)-3a and is provided in Fig. S3†). With a relatively low total barrier of 11.4 kcal mol−1 and intermediate energy of 7.4 kcal mol−1, the proposed pathway can equilibrate the mixture in a reasonable amount of time to complete the C9-epimerization.
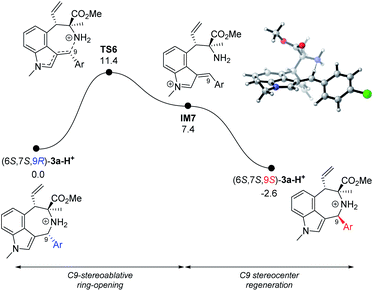 |
| Fig. 6 Mechanism of TFA-promoted C9-epimerization for (6S,6S,9R)-3a. Free energies are provided in kcal mol−1. | |
In summary, we have disclosed by quantum mechanical calculations that the stereodivergent construction of azepino[3,4,5]-indoles involves initial generation of vicinal C6/C7 stereocenters via cooperative Cu/Ir catalysis, which is followed by intramolecular Friedel–Crafts reaction with remote site-to-site chirality transfer from C6 to C9 assisted by an achiral Lewis acid. Subsequent treatment by a strong Brønsted acid can steer a late-stage C9-epimerization with a protonation-tuned thermodynamic tendency, stereoablative ring-opening and stereocenter regeneration via a second cyclization.
Conclusions
In conclusion, we have developed a cooperative Cu/Ir-catalyzed asymmetric allylic alkylation/intramolecular Friedel–Crafts reaction of azomethine ylides with 4-indolyl allyl carbonates, affording a facile access to a variety of biologically important chiral azepino[3,4,5-cd] indoles in a stereodivergent manner. The Cu-stabilized azomethine ylide in situ generated from aldimine esters serving as an ambiphilic 3-atom component and the unique π-allyl-iridium species in situ generated from 4-indolyl allylic carbonates serving as an ambiphilic 4-atom component were essential for the success of this stereodivergent formal 1,3-dipolar (3 + 4) cycloaddition of azomethine ylides. From the same set of starting materials, four 6,9-cis-stereoisomers of the cycloadduct could be accessed at will with excellent diastereoselectivity and enantioselectivity by employing an appropriate permutation of chiral copper and chiral iridium catalysts. Of particular note, merging the synergistic catalysis with TFA-promoted C9-epimerization enables the predictable preparation of all eight stereoisomers of the azepino[3,4,5-cd] indole derivatives bearing three stereogenic centers, which provides the first example of fully stereodivergent formal 1,3-dipolar (3 + 4) cycloaddition for the construction of seven-membered N-heterocycles. By quantum mechanical computations, we unravelled that the stereochemical information at the three stereocenters is sequentially established as the reaction proceeds, the key stereocontrolling steps being the generation of allyl-iridium, the diastereoface-selective coupling with Cu-AMY species, and the Lewis-acid-promoted intramolecular Friedel–Crafts reaction of the resultant allylation intermediate. The presence of a strong Brønsted acid can reverse the intrinsic thermodynamic favorability of 6,9-cis-stereoisomers, reinstitute the allylated intermediate, and reconstruct the ring system with a 6,9-trans-stereoselectivity to complete the C9-epimerization, thus granting access to up to eight stereoisomers.
Data availability
All experimental and characterization data in this manuscript are available in the ESI.† Crystallographic data for compounds (6S,7S,9R)-3a, (6R,7R,9R)-3a, and (6R,7S,9R)-3a have been deposited at the CCDC and assigned numbers 2090369–2090371, respectively.
Author contributions
C. J. W. conceived and designed the research. C. J. W. and X. Q. D. directed the project. L. X., F. X. and C. F. performed the research. B. L. and Y. D. performed the DFT calculations. L. W., Y. D., and C. J. W. co-wrote the paper with assistance from L. X. and X. Q. D. All authors analyzed the data, discussed the results, and commented on the manuscript.
Conflicts of interest
There are no conflicts to declare.
Acknowledgements
This work was supported by NSFC (220711186, 220711187, and 22073067) and the Hubei Province Natural Science Foundation (2020CFA036 and 2021CFA069). The Program of Introducing Talents of Discipline to Universities of China (111 Project) is also appreciated.
Notes and references
- S. L. Schreiber, Science, 2000, 287, 1964–1969 CrossRef CAS PubMed.
- M. D. Burke and S. L. Schreiber, Angew. Chem., Int. Ed., 2004, 43, 46–58 CrossRef PubMed.
-
(a) C. Chen, X. Li and S. L. Schreiber, J. Am. Chem. Soc., 2003, 125, 10174–10175 CrossRef CAS PubMed;
(b) R. Huisgen, Angew. Chem., Int. Ed., 1963, 2, 565–598 CrossRef;
(c)
A. Padwa and W. H. Pearson, Synthetic Applications of 1,3-Dipolar Cycloaddition Chemistry Toward Heterocycles and Natural Products, Wiley-VCH, New York, 2002 CrossRef.
-
(a) L. Wei, X. Chang and C.-J. Wang, Acc. Chem. Res., 2020, 53, 1084–1100 CrossRef CAS PubMed;
(b) X. Fang and C. J. Wang, Org. Biomol. Chem., 2018, 16, 2591–2601 RSC;
(c) J. Adrio and J. C. Carretero, Chem. Commun., 2019, 55, 11979–11991 RSC;
(d) T. Hashimoto and K. Maruoka, Chem. Rev., 2015, 115, 5366–5412 CrossRef CAS PubMed.
- For the most recent examples of transition-metal catalyzed asymmetric 1,3-dipolar (3 + 2) cycloadditions, see:
(a) X. Chang, Y. Yang, C. Shen, K.-S. Xue, Z.-F. Wang, H. Cong, H.-Y. Tao, L. W. Chung and C.-J. Wang, J. Am. Chem. Soc., 2021, 143, 3519–3535 CrossRef CAS PubMed;
(b) Z. Wang, D.-C. Wang, M.-S. Xie, G.-R. Qu and H.-M. Guo, Org. Lett., 2020, 22, 164–167 CrossRef CAS PubMed;
(c) H. Cui, K. Li, Y. Wang, M. Song, C. Wang, D. Wei, E.-Q. Li, Z. Duan and F. Mathey, Org. Biomol. Chem., 2020, 18, 3740–3746 RSC;
(d) X. Cheng, D. Yan, X.-Q. Dong and C.-J. Wang, Asian J. Org. Chem., 2020, 9, 1567–1570 CrossRef CAS;
(e) X.-J. Zou, W.-L. Yang, J.-Y. Zhu and W.-P. Deng, Chin. J. Chem., 2020, 38, 435–438 CrossRef CAS;
(f) B. Liu, W. Li, H.-H. Wu and J. Zhang, Org. Chem. Front., 2019, 6, 694–698 RSC;
(g) L. Liang, H.-Y. Niu, D.-C. Wang, X.-H. Yang, G.-R. Qu and H.-M. Guo, Chem. Commun., 2019, 55, 553–556 RSC;
(h) Z. Gan, M. Zhi, R. Han, E.-Q. Li, Z. Duan and F. Mathey, Org. Lett., 2019, 21, 2782–2785 CrossRef CAS PubMed;
(i) X. Chang, X.-S. Sun, C. Che, Y.-Z. Hu, H.-Y. Tao and C.-J. Wang, Org. Lett., 2019, 21, 1191–1196 CrossRef CAS PubMed;
(j) G. S. Caleffi, O. Larranaga, M. Ferrandiz-Saperas, P. R. R. Costa, C. Najera, A. de Cozar, F. P. Cossio and J. M. Sansano, J. Org. Chem., 2019, 84, 10593–10605 CrossRef CAS PubMed;
(k) Y.-P. Zhang, Y. You, J.-Q. Zhao, X.-J. Zhou, X.-M. Zhang, X.-Y. Xu and W.-C. Yuan, Org. Chem. Front., 2019, 6, 1879–1884 RSC;
(l) C. Shen, Y. Yang, L. Wei, W.-W. Dong, L. W. Chung and C.-J. Wang, iScience, 2019, 11, 146–159 CrossRef CAS PubMed;
(m) F. Cheng, S. J. Kalita, Z.-N. Zhao, X. Yang, Y. Zhao, U. Schneider, N. Shibata and Y.-Y. Huang, Angew. Chem., Int. Ed., 2019, 58, 16637–16643 CrossRef CAS PubMed;
(n) Y. Xiong, Z. Du, H. Chen, Z. Yang, Q. Tan, C. Zhang, L. Zhu, Y. Lan and M. Zhang, J. Am. Chem. Soc., 2019, 141, 961–971 CrossRef CAS PubMed.
- For examples of transition-metal catalyzed asymmetric 1,3-dipolar (3 + 3) cycloadditions, see:
(a) M.-C. Tong, X. Chen, H.-Y. Tao and C.-J. Wang, Angew. Chem., Int. Ed., 2013, 52, 12377–12380 CrossRef CAS PubMed;
(b) H. Guo, H. Liu, F.-L. Zhu, R. Na, H. Jiang, Y. Wu, L. Zhang, Z. Li, H. Yu, B. Wang, Y. Xiao, X.-P. Hu and M. Wang, Angew. Chem., Int. Ed., 2013, 52, 12641–12645 CrossRef CAS PubMed;
(c) C. Yuan, H. Liu, Z. Gao, L. Zhou, Y. Feng, Y. Xiao and H. Guo, Org. Lett., 2015, 17, 26–29 CrossRef CAS PubMed;
(d) W.-L. Yang, C.-Y. Li, W.-J. Qin, F.-F. Tang, X. Yu and W.-P. Deng, ACS Catal., 2016, 6, 5685–5690 CrossRef CAS;
(e) F. Xiao, S.-M. Xu, X.-Q. Dong and C.-J. Wang, Org. Lett., 2021, 23, 706–710 CrossRef CAS PubMed;
(f) M. Potowski, J. O. Bauer, C. Strohmann, A. P. Antonchick and H. Waldmann, Angew. Chem., Int. Ed., 2012, 51, 9512–9516 CrossRef CAS PubMed;
(g) Z.-L. He, H.-L. Teng and C.-J. Wang, Angew. Chem., Int. Ed., 2013, 52, 2934–2938 CrossRef CAS PubMed;
(h) Q.-H. Li, L. Wei and C.-J. Wang, J. Am. Chem. Soc., 2014, 136, 8685–8692 CrossRef CAS PubMed;
(i) H. Liu, Y. Wu, Y. Zhao, Z. Li, L. Zhang, W. Yang, H. Jiang, C. Jing, H. Yu, B. Wang, Y. Xiao and H. Guo, J. Am. Chem. Soc., 2014, 136, 2625–2629 CrossRef CAS PubMed;
(j) H.-L. Teng, L. Yao and C.-J. Wang, J. Am. Chem. Soc., 2014, 136, 4075–4080 CrossRef CAS PubMed;
(k) Z.-L. He, F. K. Sheong, Q.-H. Li, Z. Lin and C.-J. Wang, Org. Lett., 2015, 17, 1365–1368 CrossRef CAS PubMed.
-
(a) G. A. Molander, Acc. Chem. Res., 1998, 31, 603–609 CrossRef CAS;
(b) C. Galli and L. Mandolini, Eur. J. Org. Chem., 2000, 3117–3125 CrossRef CAS.
-
(a) K. Liu, H.-L. Teng and C.-J. Wang, Org. Lett., 2014, 16, 4508–4511 CrossRef CAS PubMed;
(b) Z. Dai, J. Zhu, J. Wang, W. Su, F. Yang and Q. Zhou, Adv. Synth. Catal., 2020, 362, 545–551 CrossRef CAS;
(c) N. De and E. J. Yoo, Recent Advances in the Catalytic Cycloaddition of 1,n-Dipoles, ACS Catal., 2018, 8, 48–58 CrossRef CAS.
-
(a) B. Waldeck, Chirality, 1993, 5, 350–355 CrossRef CAS PubMed;
(b) F. Lovering, J. Bikker and C. Humblet, J. Med. Chem., 2009, 52, 6752–6756 CrossRef CAS PubMed;
(c) K. M. Rentsch, J. Biochem. Biophys. Methods, 2002, 54, 1–9 CrossRef CAS PubMed.
- S. Krautwald, D. Sarlah, M. A. Schafroth and E. M. Carreira, Science, 2013, 340, 1065–1068 CrossRef CAS PubMed.
- For selected reviews and perspectives on dual-metal catalysis, see:
(a) S. Krautwald and E. M. Carreira, J. Am. Chem. Soc., 2017, 139, 5627–5639 CrossRef CAS PubMed;
(b) L. Lin and X. Feng, Chem. – Eur. J., 2017, 23, 6464–6482 CrossRef CAS PubMed;
(c) I. P. Beletskaya, C. Nájera and M. Yus, Chem. Rev., 2018, 118, 5080–5200 CrossRef CAS PubMed;
(d) Y. Wu, X. Huo and W. Zhang, Chem. – Eur. J., 2020, 26, 4895–4916 CrossRef CAS PubMed;
(e) U. B. Kim, D. J. Jung, H. J. Jeon, K. Rathwell and S.-g. Lee, Chem. Rev., 2020, 120, 13382–13433 CrossRef CAS PubMed;
(f) L. Wei and C.-J. Wang, Chin. J. Chem., 2021, 39, 15–24 CrossRef CAS.
- For selected representative stereodivergent synthesis using dual metal catalysts, metal/organocatalysts or dual organocatalysts, see:
(a) S. Krautwald, M. A. Schafroth, D. Sarlah and E. M. Carreira, J. Am. Chem. Soc., 2014, 136, 3020–3023 CrossRef CAS PubMed;
(b) T. Sandmeier, S. Krautwald, H. F. Zipfel and E. M. Carreira, Angew. Chem., Int. Ed., 2015, 54, 14363–14367 CrossRef CAS PubMed;
(c) F. A. Cruz and V. M. Dong, J. Am. Chem. Soc., 2017, 139, 1029–1032 CrossRef CAS PubMed;
(d) X. Jiang, J. J. Beiger and J. F. Hartwig, J. Am. Chem. Soc., 2017, 139, 87–90 CrossRef CAS PubMed;
(e) X. Huo, R. He, X. Zhang and W. Zhang, J. Am. Chem. Soc., 2016, 138, 11093–11096 CrossRef CAS PubMed;
(f) X. Huo, R. He, J. Fu, J. Zhang, G. Yang and W. Zhang, J. Am. Chem. Soc., 2017, 139, 9819–9822 CrossRef CAS PubMed;
(g) L. Wei, S.-M. Xu, Q. Zhu, C. Che and C.-J. Wang, Angew. Chem., Int. Ed., 2017, 56, 12312–12316 CrossRef CAS PubMed;
(h) L. Wei, Q. Zhu, S.-M. Xu, X. Chang and C.-J. Wang, J. Am. Chem. Soc., 2018, 140, 1508–1513 CrossRef CAS PubMed;
(i) X. Huo, J. Zhang, J. Fu, R. He and W. Zhang, J. Am. Chem. Soc., 2018, 140, 2080–2084 CrossRef CAS PubMed;
(j) X. Jiang, P. Boehm and J. H. Hartwig, J. Am. Chem. Soc., 2018, 140, 1239–1242 CrossRef CAS PubMed;
(k) Z.-T. He, X. Jiang and J. H. Hartwig, J. Am. Chem. Soc., 2019, 141, 13066–13073 CrossRef CAS PubMed;
(l) L. Wei, Q. Zhu, L. Xiao, H.-Y. Tao and C.-J. Wang, Nat. Commun., 2019, 10, 1594 CrossRef PubMed;
(m) Q. Zhang, H. Yu, L. Shen, T. Tang, D. Dong, W. Chai and W. Zi, J. Am. Chem. Soc., 2019, 141, 14554–14559 CrossRef CAS PubMed;
(n) R. He, X. Huo, L. Zhao, F. Wang, L. Jiang, J. Liao and W. Zhang, J. Am. Chem. Soc., 2020, 142, 8097–8103 CrossRef CAS PubMed;
(o) L. Peng, Z. He, X. Xu and C. Guo, Angew. Chem., Int. Ed., 2020, 59, 14270–14274 CrossRef CAS PubMed;
(p) S. Singha, E. Serrano, S. Mondal, C. G. Daniliuc and F. Glorius, Nat. Catal., 2020, 3, 48–54 CrossRef CAS;
(q) M. Zhu, Q. Zhang and W. Zi, Angew. Chem., Int. Ed., 2021, 60, 6545–6552 CrossRef CAS PubMed;
(r) S.-Q. Yang, Y.-F. Wang, W.-C. Zhao, G.-Q. Lin and Z.-T. He, J. Am. Chem. Soc., 2021, 143, 7285–7291 CrossRef CAS PubMed;
(s) B. Kim, Y. Kim and S. Y. Lee, J. Am. Chem. Soc., 2021, 143, 73–79 CrossRef CAS PubMed;
(t) R. Jiang, L. Ding, C. Zheng and S.-L. You, Science, 2021, 371, 380–386 CrossRef CAS PubMed;
(u) J. Zhang, X. Huo, J. Xiao, L. Zhao, S. Ma and W. Zhang, J. Am. Chem. Soc., 2021, 143, 12622–12632 CrossRef CAS PubMed;
(v) L. Xiao, L. Wei and C.-J. Wang, Angew. Chem., Int. Ed., 2021, 60, 24930–24940 CrossRef CAS PubMed;
(w) Y. Peng, X. Hong, Y. Luo, L. Wu and W. Zhang, Angew. Chem., Int. Ed., 2021, 60, 24941–24949 CrossRef CAS PubMed.
- For other selected strategies for stereodivergent synthesis, see:
(a) S.-L. Shi, Z. L. Wong and S. L. Buchwald, Nature, 2016, 532, 353–356 CrossRef CAS PubMed;
(b) D. Kaldre, I. Klose and N. Maulide, Science, 2018, 361, 664–667 CrossRef CAS PubMed;
(c) H. Zheng, Y. Wang, C. Xu, X. Xu, L. Lin, X. Liu and X. Feng, Nat. Commun., 2018, 9, 1968 CrossRef PubMed;
(d) T.-T. Gao, W.-W. Zhang, X. Sun, H.-X. Lu and B.-J. Li, J. Am. Chem. Soc., 2019, 141, 4670–4677 CrossRef CAS PubMed;
(e) X. Liu, S. Jin, W.-Y. Zhang, Q.-Q. Liu, C. Zheng and S.-L. You, Angew. Chem., Int. Ed., 2020, 59, 2039–2043 CrossRef CAS PubMed;
(f) D.-X. Zhu, J.-G. Liu and M.-H. Xu, J. Am. Chem. Soc., 2021, 143, 8583–8589 CrossRef CAS PubMed;
(g) X. Hu, X. Tang, X. Zhang, L. Lin and X. Feng, Nat. Commun., 2021, 12, 3012 CrossRef PubMed;
(h) S. Ge, W. Cao, T. Kang, B. Hu, H. Zhang, Z. Su, X. Liu and X. Feng, Angew. Chem., Int. Ed., 2019, 58, 4017–4021 CrossRef CAS PubMed;
(i) C. Xu, K. Wang, D. Li, L. Lin and X. Feng, Angew. Chem., Int. Ed., 2019, 58, 18438–18442 CrossRef CAS PubMed;
(j) C. Xu, J. Qiao, S. Dong, Y. Ahou, X. Liu and X. Feng, Chem. Sci., 2021, 12, 5458–5463 RSC.
- S.-M. Xu, L. Wei, C. Shen, L. Xiao, H.-Y. Tao and C.-J. Wang, Nat. Commun., 2019, 10, 5553 CrossRef PubMed.
- During the preparation of this manuscript, with arylidene/alkylidene aminomalonates as the precursors of non-prochiral AMYs, Deng documented an Ir-catalyzed asymmetric (3 + 4) cycloaddition of 4-indolyl allylic alcohols to generate only cis-isomeric azepino[3,4,5-cd] indoles bearing two stereogenic centers, see: W.-L. Yang, T. Ni and W.-P. Deng, Org. Lett., 2021, 23, 588–594 CrossRef CAS PubMed . However, the reported catalytic system was unable to realize the stereodivergent preparation of azepino[3,4,5-cd] indoles bearing three stereogenic centers, and prochiral aldimine esters are not compatible with such a single-catalyst protocol due to the unsatisfactory diastereo-selectivity control..
- Q. Cheng, H.-F. Tu, C. Zheng, J.-P. Qu, G. Helmchen and S.-L. You, Chem. Rev., 2019, 119, 1855–1969 CrossRef CAS PubMed.
- M. Saifuddin, P. K. Agarwal, S. K. Sharma, A. K. Mandadapu, S. Gupta, V. K. Harit and B. Kundu, Eur. J. Org. Chem., 2010, 5108–5117 CrossRef CAS.
-
(a) Y. Cai, N. Shao, H. Xie, Y. Futamura, S. Panjikar, H. Liu, H. Zhu, H. Osada and H. Zou, ACS Catal., 2019, 9, 7443–7448 CrossRef CAS;
(b) D. Robaa, C. Enzensperger, S. A. Abul, E. E. Khawass, O. E. Sayed and J. Lehmann, J. Med. Chem., 2010, 53, 2646–2650 CrossRef CAS PubMed;
(c) J. S. Scott, A. Bailey, D. Buttar, R. J. Carbajo, J. Curwen, P. R. J. Davey, R. D. M. Davies, S. L. Degorce, C. Donald, E. Gangl, R. Greenwood, S. D. Groombridge, T. Johnson, S. Lamont, M. Lawson, A. Lister, C. J. Morrow, T. A. Moss, J. H. Pink and R. Polanski, J. Med. Chem., 2019, 62, 1593–1608 CrossRef CAS PubMed;
(d) X. Xiong, H. Yuan, Y. Zhang, J. Xu, T. Ran, H. Liu, S. Lu, A. Xu, H. Li, Y. Jiang, T. Lu and Y. Chen, J. Mol. Struct., 2015, 1097, 136–144 CrossRef CAS;
(e) J. Kraxner, H. Hubner and P. Gmeiner, Arch. Pharm., 2000, 333, 287–292 CrossRef CAS.
-
(a) R. Connon and P. J. Guiry, Tetrahedron Lett., 2020, 61, 151696–151705 CrossRef CAS;
(b) T. Nemoto, S. Harada and M. Nakajima, Asian J. Org. Chem., 2018, 7, 1730–1742 CrossRef CAS.
- D.-J. Cheng, H.-B. Wu and S.-K. Tian, Org. Lett., 2011, 13, 5636–5639 CrossRef CAS PubMed.
-
(a) J. F. Teichert and B. L. Feringa, Angew. Chem., Int. Ed., 2010, 49, 2486–2528 CrossRef CAS PubMed;
(b) C. J. Richards and A. W. Mulvaney, Tetrahedron: Asymmetry, 1996, 7, 1419–1430 CrossRef CAS.
- R. Wang, X. Hong and Z. Shan, Tetrahedron Lett., 2008, 49, 636–639 CrossRef CAS.
- CCDC 2090369 ((6S,7S,9R)-3a), 2090370 ((6R,7R,9R)-3a), and 2090371 ((6R,7S,9R)-3a) contain the supplementary crystallographic data for this paper.
- Y. Zhao, L. Liu, W. Sun, J. Lu, D. McEachern, X. Li, S. Yu, D. Bernard, P. Ochsenein, V. Ferey, J.-C. Carry, J. R. Deschamps, D. Sun and S. Wang, J. Am. Chem. Soc., 2013, 135, 7223–7234 CrossRef CAS PubMed.
- All calculations were performed using the Gaussian 09 software package: M. J. Frisch, G. W. Trucks, H. B. Schlegel, G. E. Scuseria, M. A. Robb, J. R. Cheeseman, G. Scalmani, V. Barone, B. Mennucci, G. A. Petersson, H. Nakatsuji, M. Caricato, X. Li, H. P. Hratchian, A. F. Izmaylov, J. Bloino, G. Zheng, J. L. Sonnenberg, M. Hada, M. Ehara, K. Toyota, R. Fukuda, J. Hasegawa, M. Ishida, T. Nakajima, Y. Honda, O. Kitao, H. Nakai, T. Vreven, J. A. Montgomery Jr, J. E. Peralta, F. Ogliaro, M. Bearpark, J. J. Heyd, E. Brothers, K. N. Kudin, V. N. Staroverov, T. Keith, R. Kobayashi, J. Normand, K. Raghavachari, A. Rendell, J. C. Burant, S. S. Iyengar, J. Tomasi, M. Cossi, N. Rega, J. M. Millam, M. Klene, J. E. Knox, J. B. Bakken, V. Cross, C. Adamo, J. Jaramillo, R. Gomperts, R. E. Stratmann, O. Yazyev, A. J. Austin, R. Cammi, C. Pomelli, J. W. Ochterski, R. L. Martin, K. Morokuma, V. G. Zakrzewski, G. A. Voth, P. Salvador, J. J. Dannenberg, S. Dapprich, A. D. Daniels, O. Farkas, J. B. Foresman, J. V. Ortiz, J. Cioslowski and D. J. Fox, Gaussian 09, Gaussian, Inc., Wallingford, CT, 2013 Search PubMed.
- Y. Zhao and D. G. Truhlar, J. Chem. Phys., 2006, 125, 194101–194118 CrossRef PubMed.
- Selected mechanistic studies on stereodivergent dual transition metal catalysis:
(a) A. Changotra, B. Bhaskararao, C. M. Hadad and R. B. Sunoj, J. Am. Chem. Soc., 2020, 142, 9612–9624 CAS;
(b) H. Xu, B. Li, Z. Liu and Y. Dang, ACS Catal., 2021, 11, 9008–9021 CrossRef CAS.
- Other selected theoretical mechanistic studies on stereodivergent synergistic catalysis:
(a) B. Bhaskararao and R. B. Sunoj, J. Am. Chem. Soc., 2015, 137, 15712–15722 CrossRef CAS PubMed;
(b) B. Bhaskararao and R. B. Sunoj, ACS Catal., 2017, 7, 6675–6685 CrossRef CAS;
(c) S. Tribedi, C. M. Hadad and R. B. Sunoj, Chem. Sci., 2018, 9, 6126–6133 RSC;
(d) B. Bhaskararao and R. B. Sunoj, Chem. Sci., 2018, 9, 8738–8747 RSC.
-
(a) C. A. Kiener, C. Shu, C. Incarvito and J. F. Hartwig, J. Am. Chem. Soc., 2003, 125, 14272–14273 CrossRef CAS PubMed;
(b) J. F. Hartwig and L. M. Stanley, Acc. Chem. Res., 2010, 43, 1461–1475 CrossRef CAS PubMed;
(c) D. Marković and J. F. Hartwig, J. Am. Chem. Soc., 2007, 129, 11680–11681 CrossRef PubMed;
(d) S. T. Madrahimov, D. Markovic and J. F. Hartwig, J. Am. Chem. Soc., 2009, 131, 7228–7229 CrossRef CAS PubMed;
(e) A. Leitner, S. Shekhar, M. J. Pouy and J. F. Hartwig, J. Am. Chem. Soc., 2005, 127, 15506–15514 CrossRef CAS PubMed.
- Molecular visualization was carried out in CYLview and PyMOL:
(a)
C. Y. Legault, CYLview, 1.0b, Université de Sherbrooke: Quebec, Montreal, Canada, 2009 Search PubMed;
(b)
W. L. Delano, The PyMOL molecular graphics system, Delano Scientific, 2002 Search PubMed.
- P. Liu, J. Montgomery and K. N. Houk, J. Am. Chem. Soc., 2011, 133, 6956–6959 CrossRef CAS PubMed.
- Steric contours were produced in the Multiwfn code: T. Lu and F. Chen, J. Comput. Chem., 2012, 33, 580–592 CrossRef CAS PubMed.
-
(a) S. Goncalves, S. Santoro, M. Nicolas, A. Wagner, P. Maillos, F. Himo and R. Baati, J. Org. Chem., 2011, 76, 3274–3285 CrossRef CAS PubMed;
(b) G. Alachouzos, C. Holt and A. J. Frontier, Org. Lett., 2020, 22, 4010–4015 CrossRef CAS PubMed;
(c) G.-P. Wang, M.-Q. Chen, S.-F. Zhu and Q.-L. Zhou, Chem. Sci., 2017, 8, 7197–7202 RSC.
Footnotes |
† Electronic supplementary information (ESI) available. CCDC 2090369–2090371. For ESI and crystallographic data in CIF or other electronic format see DOI: 10.1039/d1sc07271d |
‡ These two authors contributed equally. |
|
This journal is © The Royal Society of Chemistry 2022 |
Click here to see how this site uses Cookies. View our privacy policy here.