DOI:
10.1039/D2SC02827A
(Edge Article)
Chem. Sci., 2022,
13, 11427-11432
A base-catalyzed approach for the anti-Markovnikov hydration of styrene derivatives†
Received
19th May 2022
, Accepted 24th August 2022
First published on 26th August 2022
Abstract
The base-catalyzed addition of 1-cyclopropylethanol to styrene derivatives with an acidic reaction workup enables anti-Markovnikov hydration. The use of either catalytic organic superbase or crown ether-ligated inorganic base permits hydration of a wide variety of styrene derivatives, including electron-deficient, ortho-substituted and heteroaryl variants. This protocol complements alternative routes to terminal alcohols that rely on stoichiometric reduction and oxidation processes. The utility of this method is demonstrated through multigram scale reactions and its use in a two-step hydration/cyclization process of ortho-halogenated styrenes to prepare 2,3-dihydrobenzofuran derivatives.
Introduction
β-Aryl alcohols are an important alcohol subclass that find wide utility in organic chemistry. This substructure is prominently featured in bioactive compounds (Fig. 1a)1 and is a common synthetic intermediate for phenethyl-functionalized compounds, including several pharmaceuticals (Fig. 1b).2 Medicinal chemistry discovery efforts also frequently employ this building block to perform structure activity relationship (SAR) studies on phenethyl units, with three such examples shown in Fig. 1c.3 Two common approaches to β-aryl alcohols are reduction of arylacetic acids4 and hydroboration/oxidation of styrene derivatives.5,6 While reliable for simple substrates, these methods have limitations that can prevent rapid access to alcohols with diverse aryl groups. For instance, non-commercial arylacetic acids require separate multistep syntheses.7 Meanwhile, traditional stoichiometric hydroboration/oxidation protocols8 are often unselective and low yielding for electron-deficient or ortho-substituted styrenes and heteroaryl variants, thus requiring alternative hydroboration methods and reagents.9 A new approach to β-aryl alcohols from styrene derivatives that does not rely on reduction or oxidation events could therefore improve access to this valuable class of alcohol.10
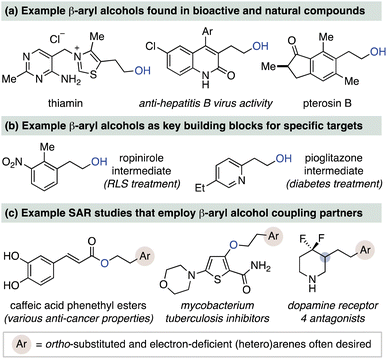 |
| Fig. 1 Examples of the importance of β-aryl substituted alcohols. | |
The catalytic anti-Markovnikov hydration of aryl-substituted alkenes has long been desired as a sustainable and complementary alternative to stoichiometric hydroboration/oxidation processes.11 Several strategies toward this goal have recently been reported for styrenes, including Grubbs' triple relay catalytic process,12 Arnold's and Li's biocatalytic approaches,13 and Lei's photocatalytic alkene oxidation method.14 While these methods are impressive in their strategy for achieving anti-Markovnikov selectivity, they have yet to be applied toward more complex styrene substrates, including vinyl N-heteroarenes. Thus, there remains a current challenge to develop catalytic hydration methods that can access densely functionalized β-aryl alcohols to better reflect the structural diversity represented in Fig. 1.
We proposed to use base catalysis for the anti-Markovnikov hydration of styrene derivatives as a new approach to β-aryl alcohols. We recently disclosed the use of the phosphazene superbase P4-t-Bu or 18-crown-6-ligated KO-t-Bu as basic catalysts for the nucleophilic addition of alcohols to aryl-substituted alkenes.15,16 Water does not participate in this reaction, so we instead sought a nucleophilic “protected water” source that could be used to achieve formal hydration (Fig. 2).17 Thus, following hydroetherification, facile deprotection could provide straightforward access to β-aryl alcohols with complete regiocontrol. We herein report the discovery of an unconventional nucleophilic water surrogate that enables anti-Markovnikov hydration of styrene derivatives.
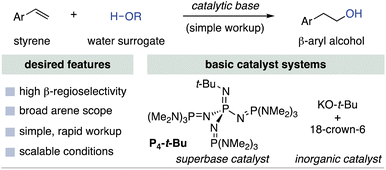 |
| Fig. 2 Overview of a base-catalyzed approach to anti-Markovnikov styrene hydration. | |
Results and discussion
Base-catalyzed alcohol addition reactions to styrene derivatives are reversible such that the substrate identities and reaction conditions contribute to the observed equilibrium yields. These considerations and the identification of effective catalysts are extensively documented in a recent report from our group that served as the basis for this work.15b,d We began by examining P4-t-Bu-catalyzed addition reactions using oxygen pronucleophiles that could potentially serve as water surrogates.18 These reactions were conducted under our previously optimized reaction conditions with 2-chloro-3-vinylpyridine (1) as a model alkene (Scheme 1a).15d Alcohols comprised of common O-protecting groups, such as trimethylsilanol, phenol, p-methoxybenzyl alcohol and allyl alcohol do not undergo addition. These results are consistent with our previous studies that revealed alcohol addition to styrenes is both kinetically and thermodynamically challenging, indicating a more nucleophilic water surrogate is necessary for addition.15,19 However, alcohols comprised of common aliphatic protecting groups, such as tert-butanol and 2-trimethylsilylethanol, provide low addition yields. Examination of cyclopropyl-substituted alcohols led to the discovery that 1-cyclopropylethanol undergoes addition in 82% isolated yield. The resulting 1-methyl 1′-cyclopropylmethyl (MCPM) ether is an uncommon protecting group developed for oligosaccharide synthesis.20 For comparison, cyclopropylmethanol, dicyclopropylmethanol and iso-propanol all provide lower addition yields. The isolated MCPM ether 2 undergoes high-yielding deprotection in 3 min using methanesulfonic acid, presumably through a cyclopropyl-stabilized carbocation hydrolysis mechanism (Scheme 1b).21
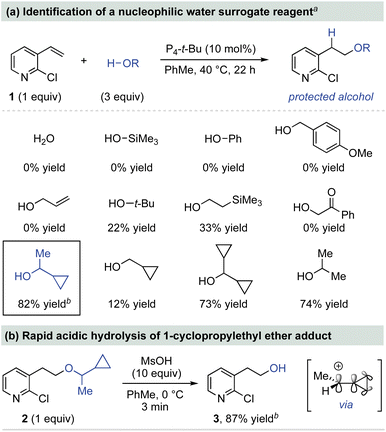 |
| Scheme 1 Optimization studies for (a) superbase-catalyzed alcohol addition and (b) ether hydrolysis. a Yields determined by 1H NMR spectroscopy. b Isolated yield of purified product. | |
We next investigated the substrate scope for anti-Markovnikov hydration using 1-cyclopropylethanol (4) and an in situ acidic workup procedure (Table 1). First, in Table 1a, a comparison of superbase (P4-t-Bu in PhMe) and inorganic (KO-t-Bu/18-crown-6 in DME) catalysts is shown for two styrene derivatives (5 and 6) and two chlorinated vinyl pyridines (3 and 7). The inorganic conditions were selected based on our prior extensive screening of bases for hydroetherification reactions,15b with additional information provided in the ESI.† As seen in Table 1a, the inorganic conditions enable synthetically useful alcohol yields (49–61%) while the use of P4-t-Bu generally provides higher yields (60–83%). The yield differences between the superbase and inorganic conditions are likely a solvent equilibrium effect, as we previously measured alcohol addition to be more favorable in PhMe than in DME.15b While P4-t-Bu is an efficient catalyst in both solvents, the KO-t-Bu/18-crown-6 system is most active in DME and typically does not catalyze the reaction in PhMe to its equilibrium position.15d
Table 1 Product scope for anti-Markovnikov hydrationa
Yields are of purified alcohol product; reactions performed on 0.5 to 1.0 mmol scale.
5 equiv. of 4 used.
1 h reaction time.
4 equiv. of 4 used.
|
|
Table 1b shows hydration products of other vinyl arenes using the superbase conditions, while a full comparison to inorganic conditions is provided in the ESI.† Styrenes (5, 6, 8–15) featuring diverse substitution patterns with nitro, fluoro, chloro, bromo, sulfonyl, trifluoromethyl and benzoyl substituents undergo hydration in good yields. A notable feature of this scope is that ortho-substituents are well tolerated. Vinyl heteroarenes are also effective substrates, including those with halogens prone to nucleophilic aromatic substitution. Thus, halogenated 2-, 3-, and 4-vinyl pyridine derivatives (3, 7, 17 and 18) undergo hydration in high yields. Beyond pyridines, quinoline (16) and thiazole (19) derivatives are also hydrated in good yields. Although 1-cyclopropylethanol (4) will add to electron-neutral styrenes and 1,1-disubstituted variants, only low equilibrium yields are observed under these conditions.15b Representative substrates documenting the electronic limitations of this method are shown in the ESI.†
We next compared the hydration yields of several substrates from Table 1 to traditional hydroboration/oxidation protocols using BH3·THF and 9-BBN (Scheme 2a). Although use of these boranes results in high yields of β-phenethyl alcohols from electron-neutral styrenes, decreased yields and selectivities are common for electron-deficient and heteroaryl variants.8 Hydroboration/oxidation of 2,6-dichlorostyrene (20) leads to mixtures of α- and β-phenethyl alcohols while 2-nitro-4-methylstyrene (21) results in low yields of β-phenethyl alcohol. An even greater limitation is observed for 3-bromo-4-vinylpyridine (22), in which only net hydrogenation occurs with no alcohol formation. In contrast, base-catalyzed hydration gives high yields with exclusive β-regioselectivity for these substrates.
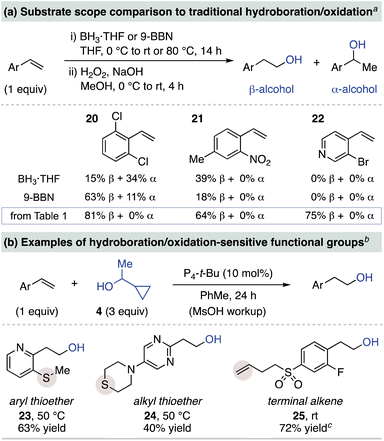 |
| Scheme 2 Comparison of base-catalyzed hydration to hydroboration/oxidation methods. a Yields and regioselectivities determined by 1H NMR spectroscopy of the crude reaction mixture; yields from Table 1 represent isolated yields. b Yields are of purified alcohol product. c 5 equiv. of 4 used. | |
Base-catalyzed hydration is also likely to have complementary functional group tolerance to hydroboration/oxidation protocols. This is illustrated in Scheme 2b, where substrates with an aryl thioether (23) and a thiomorpholine (24) that are sensitive to oxidizing conditions undergo P4-t-Bu-catalyzed hydration in moderate yields.22 Substrate 25, featuring a terminal alkene that is reactive toward traditional hydroboration, illustrates the chemoselectivity of this method for styrene hydration.23
Scheme 3 demonstrates the scalability of this protocol through three hydration reactions conducted on 10 mmol scale or greater. Hydration of 3-bromo-4-vinylpyridine (22) is best accomplished using P4-t-Bu and thus 5 mol% catalyst loading provided access to 1.4 grams of alcohol 17.24 The hydration of 2,6-dichlorostyrene (20, 30 mmol) was achieved in 73% yield using catalytic KO-t-Bu, exemplifying the practicality of the inorganic conditions. Although the highest styrene hydration yields are obtained using 3 equiv. of 1-cyclopropylethanol (4), we also identified conditions that allow for only moderately decreased addition yields using 1.2 equiv. of 4. This finding, shown in Table S3† of the ESI,† exploits our previous discovery of a negative alcohol rate order for base-catalyzed hydroetherification reactions.15b Thus, reactions can be run at lower temperatures when less alcohol is used, thereby decreasing the entropic penalty of addition to counteract Le Chatelier's principle. This effect was extended to a challenging oxa-Michael addition process for acrylamide 26, where just 1.5 equiv. of 4 and 30 min are required for a high-yielding KO-t-Bu-catalyzed hydration process at room temperature.25
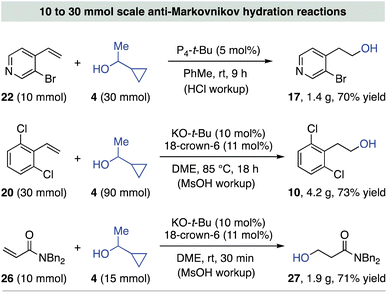 |
| Scheme 3 Examples of preparative scale anti-Markovnikov hydration reactions; yields are of isolated alcohol product. | |
Given that this method is suitable for ortho-halogenated vinyl (hetero)arenes, we reasoned hydration could be sequenced with a cyclization reaction to prepare 2,3-dihydrobenzofuran derivatives.26 Thus, employing substrates from Table 1, base-promoted or metal-catalyzed cyclization reactions produce 28–32 in high yields (Scheme 4). This approach should improve access to this important heterocycle class,27 especially functionalized and aza variants where there is limited availability of the corresponding benzofuran compounds.28
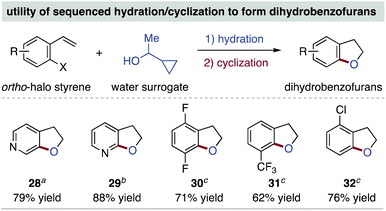 |
| Scheme 4 Utility of Table 1 alcohol products for dihydrobenzofuran derivative synthesis; yields are of isolated product from the cyclization step using given conditions. a CuI (10 mol%), 8-quinolinol (15 mol%), Cs2CO3 (1.5 equiv.), PhMe, 110 °C, 16 h. b NaH (1.5 equiv.), THF, 70 °C, 16 h. c Pd(OAc)2 (3 mol%), JohnPhos (4 mol%), Cs2CO3 (1.5 equiv.), PhMe, 80 °C, 22 h. | |
Conclusions
In conclusion, base-catalyzed addition of 1-cyclopropylethanol to styrene derivatives provides a convenient method for preparing β-aryl substituted alcohols. The use of catalytic P4-t-Bu superbase allows for superior yields to inorganic bases, although KO-t-Bu/18-crown-6 may be a more practical catalyst system for hydration on larger scale. In the broader context, analogous application of the nucleophilic yet easily deprotected 1-cyclopropylethanol water surrogate can likely enable other challenging hydration processes.
Data availability
Compound characterization data and experimental procedures are openly available in the ESI.†
Author contributions
S. P. P., Z. Q. and S. J. S. performed the experimental work. The mansucript was written by J. S. B. with input from all authors.
Conflicts of interest
There are no conflicts to declare.
Acknowledgements
This material is based upon work supported by the National Science Foundation under Grant No. 1944478. We also thank Colorado State University (CSU) for funding and the Analytical Resources Core (RRID: SCR_021758) at CSU for instrument access, training and assistance with sample analysis.
Notes and references
- For references that discuss compounds shown in Fig. 1a, see:
(a) S. Manzetti, J. Zhang and D. van der Spoel, Biochem., 2014, 53, 821–835 CrossRef CAS PubMed;
(b) R.-H. Guo, Q. Zhang, Y.-B. Ma, J. Luo, C.-A. Geng, L.-J. Wang, X.-M. Zhang, J. Zhou, Z.-Y. Jiang and J.-J. Chen, Eur. J. Med. Chem., 2011, 46, 307–319 CrossRef CAS PubMed;
(c) H. R. Dexter, E. Allen and D. M. Williams, Tetrahedron Lett., 2018, 59, 4323–4325 CrossRef CAS.
- For references that discuss compounds shown in Fig. 1b, see:
(a) H. Chen, Y. Chen, Q. Zou and L. Yuan, Org. Process Res. Dev., 2013, 17, 714–717 CrossRef CAS;
(b) L. R. Madivada, R. R. Anumala, G. Gilla, S. Alla, K. Charagondla, M. Kagga, A. Bhattacharya and R. Bandichhor, Org. Process Res. Dev., 2009, 13, 1190–1194 CrossRef CAS.
- For references that discuss compounds shown in Fig. 1c, see:
(a) J. Xie, F. Yang, M. Zhang, C. Lam, Y. Qiao, J. Xiao, D. Zhang, Y. Ge, L. Fu and D. Xie, Bioorg. Med. Chem. Lett., 2017, 27, 131–134 CrossRef CAS PubMed;
(b) J. T. Sanderson, H. Clabault, C. Patton, G. Lassalle-Claux, J. Jean-François, A. F. Paré, M. J. G. Hébert, M. E. Surette and M. Touaibia, Bioorg. Med. Chem., 2013, 21, 7182–7193 CrossRef CAS PubMed;
(c) L. A. T. Cleghorn, P. C. Ray, J. Odingo, A. Kumar, H. Wescott, A. Korkegian, T. Masquelin, A. Lopez Moure, C. Wilson, S. Davis, M. Huggett, P. Turner, A. Smith, O. Epemolu, F. Zuccotto, J. Riley, P. Scullion, Y. Shishikura, L. Ferguson, J. Rullas, L. Guijarro, K. D. Read, S. R. Green, P. Hipskind, T. Parish and P. G. Wyatt, J. Med. Chem., 2018, 61, 6592–6608 CrossRef CAS PubMed;
(d) D. E. Jeffries, J. O. Witt, A. L. McCollum, K. J. Temple, M. A. Hurtado, J. M. Harp, A. L. Blobaum, C. W. Lindsley and C. R. Hopkins, Bioorg. Med. Chem. Lett., 2016, 26, 5757–5764 CrossRef CAS PubMed.
-
(a)
M. B. Smith, March's Advanced Organic Chemistry, 7th edn, Wiley, New York, 2013, pp. 1497–1519 Search PubMed;
(b) S. Werkmeister, K. Junge and M. Beller, Org. Process Res. Dev., 2014, 18, 289–302 CrossRef CAS;
(c) J. Pritchard, G. A. Filonenko, R. van Putten, E. J. M. Hensen and E. A. Pidko, Chem. Soc. Rev., 2015, 44, 3808–3833 RSC.
- For reviews on uncatalyzed hydroboration, see:
(a)
H. C. Brown, Hydroboration, W. A. Benjamin, Inc., New York, 1962 Search PubMed;
(b)
R. S. Dhilon, Hydroboration and Organic Synthesis, Springer, Berlin, Germany, 2007 Search PubMed;
(c) H. C. Brown, Tetrahedron, 1961, 12, 117–138 CrossRef CAS.
- A variety of alternative routes have been employed toward β-aryl alcohols; for representative examples, see:
(a) S. Mohanty, S. Talasila, A. K. Roy and A. C. Karmakar, Org. Process Res. Dev., 2014, 18, 168–173 CrossRef CAS;
(b) C. Huynh, F. Derguini-Boumechal and G. Lindstrumelle, Tetrahedron Lett., 1979, 20, 1503–1506 CrossRef;
(c) J. V. Obligacion and P. J. Chirik, Nat. Rev. Chem., 2018, 2, 15–34 CrossRef CAS PubMed;
(d) M. D. Greenhalgh, D. J. Frank and S. P. Thomas, Adv. Synth. Catal., 2014, 356, 584–590 CrossRef CAS;
(e) M. Zeng, L. Li and S. B. Herzon, J. Am. Chem. Soc., 2014, 136, 7058–7067 CrossRef CAS PubMed;
(f) T. Zhang, Z. Zhang, Y. Nishiyama and H. Maekawa, Tetrahedron, 2016, 72, 2293–2299 CrossRef CAS.
- For example routes to arylacetic acid derivatives, see the following references and references therein:
(a) M. R. Biscoe and S. L. Buchwald, Org. Lett., 2009, 11, 1773–1775 CrossRef CAS PubMed;
(b) T. Hama, S. Ge and J. F. Hartwig, J. Org. Chem., 2013, 78, 8250–8266 CrossRef CAS PubMed;
(c) P. J. Moon, S. Yin and R. J. Lundgren, J. Am. Chem. Soc., 2016, 138, 13826–13829 CrossRef CAS PubMed;
(d) P. Xie, Y. Xie, B. Qian, H. Zhou, C. Xia and H. Huang, J. Am. Chem. Soc., 2012, 134, 9902–9905 CrossRef CAS PubMed;
(e) Q.-Y. Meng, T. E. Schirmer, A. L. Berger, K. Donabauer and B. König, J. Am. Chem. Soc., 2019, 141, 11393–11397 CrossRef CAS PubMed.
- For discussions of stoichiometric hydroboration on challenging styrene substrates, see:
(a) H. C. Brown, J. V. N. Vara Prasad and S. H. Zee, J. Org. Chem., 1986, 51, 439–445 CrossRef CAS;
(b) H. C. Brown and R. L. Sharp, J. Am. Chem. Soc., 1966, 88, 5851–5854 CrossRef CAS;
(c) L. C. Vishwakarma and A. Fry, J. Org. Chem., 1980, 45, 5306–5308 CrossRef CAS;
(d) P. V. Ramachandran, S. Madhi and M. J. O'Donnell, J. Fluorine Chem., 2006, 127, 1252–1255 CrossRef CAS.
- For reviews and examples of metal-catalyzed and other approaches to hydroboration, see:
(a) K. Semba, T. Fujihara, J. Terao and Y. Tsuji, Tetrahedron, 2015, 71, 2183–2197 CrossRef CAS;
(b) K. Burgess and M. J. Ohlmeyer, Chem. Rev., 1991, 91, 1179–1191 CrossRef CAS;
(c) I. Beletskaya and A. Pelter, Tetrahedron, 1997, 53, 4957–5026 CrossRef CAS;
(d) J. H. Docherty, J. Peng, A. P. Dominey and S. P. Thomas, Nat. Chem., 2017, 9, 595–600 CrossRef CAS;
(e) R. Corberán, N. W. Mszar and A. H. Hoveyda, Angew. Chem., Int. Ed., 2011, 50, 7079–7082 CrossRef;
(f) J. V. Obligacion and P. J. Chirik, J. Am. Chem. Soc., 2013, 135, 19107–19110 CrossRef CAS PubMed;
(g) Z. Kuang, K. Yang, Y. Zhou and Q. Song, Chem. Commun., 2020, 56, 6469–6479 RSC;
(h) T. Taniguchi, Eur. J. Org. Chem., 2019, 6308–6319 CrossRef CAS.
- An alternative oxidation/reduction route to terminal alcohols is the regioselective reductive opening of styrene oxide compounds. For selected recent examples, see:
(a) C. Yao, T. Dahmen, A. Gansäuer and J. Norton, Science, 2019, 364, 764–767 CrossRef CAS PubMed;
(b) X. Liu, L. Longwitz, B. Spiegelberg, J. Tönjes, T. Beweries and T. Werner, ACS Catal., 2020, 10, 13659–13667 CrossRef CAS;
(c) M. Magre, E. Paffenholz, B. Maity, L. Cavallo and M. Rueping, J. Am. Chem. Soc., 2020, 142, 14286–14294 CrossRef CAS PubMed;
(d) W. Liu, W. Li, A. Spannenberg, K. Junge and M. Beller, Nat. Catal., 2019, 2, 523–528 CrossRef CAS.
- For reviews on anti-Markovnikov hydration and oxidation of alkenes, see:
(a) J. Guo and P. Teo, Dalton Trans., 2014, 43, 6952–6964 RSC;
(b) M. Beller, J. Seayad, A. Tillack and H. Jiao, Angew. Chem., Int. Ed., 2004, 43, 3368–3398 CrossRef CAS PubMed;
(c) J. J. Dong, W. R. Browne and B. L. Feringa, Angew. Chem., Int. Ed., 2015, 54, 734–744 CrossRef CAS PubMed . For two recent examples of anti-Markovnikov addition of oxygen reagents to terminal alkenes, see:;
(d) S.-Q. Lai, B.-Y. Wei, J.-W. Wang, W. Yu and B. Han, Angew. Chem., Int. Ed., 2021, 60, 21997–22003 CrossRef CAS PubMed;
(e) L. Quach, S. Dutta, P. M. Pflüger, F. Sandfort, P. Bellotti and F. Glorius, ACS Catal., 2022, 12, 2499–2504 CrossRef CAS.
- G. Dong, P. Teo, Z. K. Wickens and R. H. Grubbs, Science, 2011, 333, 1609–1612 CrossRef CAS PubMed.
-
(a) S. C. Hammer, G. Kubik, E. Watkins, S. Huang, H. Minges and F. H. Arnold, Science, 2017, 358, 215–218 CrossRef CAS;
(b) S. Wu, J. Liu and Z. Li, ACS Catal., 2017, 7, 5225–5233 CrossRef CAS;
(c) For a review, see: S. Wu, Y. Zhou and Z. Li, Chem. Commun., 2019, 55, 883–896 RSC.
-
(a) X. Hu, G. Zhang, F. Bu and A. Lei, ACS Catal., 2017, 7, 1432–1437 CrossRef CAS;
(b) Y. Chen, J. Zhang, Z. Tang and Y. Sun, J. Photochem. Photobiol. A, 2020, 392, 112340 CrossRef CAS.
-
(a) C. Luo and J. S. Bandar, J. Am. Chem. Soc., 2018, 140, 3547–3550 CrossRef CAS PubMed;
(b) C. Luo, J. V. Alegra-Requena, S. J. Sujansky, S. P. Pajk, L. C. Gallegos, R. S. Paton and J. S. Bandar, J. Am. Chem. Soc., 2022, 144, 9586–9596 CrossRef CAS PubMed;
(c) C. Luo and J. S. Bandar, Synlett, 2018, 29, 2218–2224 CrossRef CAS;
(d) Additional information regarding catalyst and condition optimization for both the superbase and inorganic catalyst systems can be found in the ESI.†.
- For reviews and references on P4-t-Bu superbase catalysis, see:
(a) R. Schwesinger and H. Schlemper, Angew. Chem., Int. Ed., 1987, 26, 1167–1169 CrossRef;
(b)
Superbases for Organic Synthesis: Guanidines, Amidines, Phosphazenes and Related Organocatalysts, ed. T. Ishikawa, John Wiley & Sons, Ltd: Chichester, UK, 2009 Search PubMed;
(c) T. R. Puleo, S. J. Sujansky, S. E. Wright and J. S. Bandar, Chem. - Eur. J., 2021, 27, 4216–4229 CrossRef CAS PubMed.
- For an example of addition of hydroxide to a highly activated vinyl N-heteroarene, see: J. M. Bartolomé-Nebreda, S. A. Alonso de Diego, M. Artola, F. Delgado, O. Delgado, M. L. Martín-Martín, C. M. Martínez-Viturro, M. A. Pena, H. M. Tong, M. Van Gool, J. M. Alonso, A. Fontana, G. J. Macdonald, A. Megens, X. Langlois, M. Somers, G. Vanhoof and S. Conde-Ceide, J. Med. Chem., 2015, 58, 978–993 CrossRef.
-
P. G. M. Wuts, Greene's Protective Groups in Organic Synthesis, 5th edn, Wiley, Hoboken, NJ, 2014 Search PubMed.
- T. R. Puleo, A. J. Strong and J. S. Bandar, J. Am. Chem. Soc., 2019, 141, 1467–1472 CrossRef CAS PubMed.
-
(a) E. Eichler, F. Yan, J. Sealy and D. M. Whitfield, Tetrahedron, 2001, 57, 6679–6693 CrossRef CAS;
(b) S. H. Yu and D. M. Whitfield, Tetrahedron, 2011, 67, 5750–5761 CrossRef CAS.
-
(a) G. A. Olah, V. P. Reddy and G. K. S. Prakash, Chem. Rev., 1992, 92, 69–95 CrossRef CAS;
(b) C. F. Wilcox, L. M. Loew and R. Hoffman, J. Am. Chem. Soc., 1973, 95, 8192–8193 CrossRef CAS.
- M. Madesclaire, Tetrahedron, 1986, 42, 5459–5495 CrossRef CAS.
- For an example of selective hydroboration of an unactivated alkene in the presence of a styrene, see: J. Y. Dong, E. Manias and T. C. Chung, Macromolecules, 2002, 35, 3439–3447 CrossRef CAS.
- We found that P4-t-Bu catalyzes the addition of alcohol 4 to alkene 22 in 95% yield whereas the inorganic catalyst system provides a maximum 68% addition yield; see ESI† for details.
- For a discussion of the challenges of oxa-Michael addition and hydration reactions, see:
(a) C. F. Nising and S. Bräse, Chem. Soc. Rev., 2012, 41, 988–999 RSC;
(b) E. Hartmann, D. J. Vyas and M. Oestreich, Chem. Commun., 2011, 47, 7917–7932 RSC;
(c) K. Zheng, X. Liu and X. Feng, Chem. Rev., 2018, 118, 7586–7656 CrossRef CAS PubMed.
- For example other routes to compounds similar to those in Scheme 4, see:
(a) S. El Sayed, A. Bordet, C. Weidenthaler, W. Hetaba, K. L. Luska and W. Leitner, ACS Catal., 2020, 10, 2124–2130 CrossRef CAS ; and references therein.;
(b) S. Kuwabe, K. E. Torraca and S. L. Buchwald, J. Am. Chem. Soc., 2001, 123, 12202–12206 CrossRef CAS PubMed;
(c) J. Alvarado, J. Fournier and A. Zakarian, Angew. Chem., Int. Ed., 2016, 55, 11625–11628 CrossRef CAS PubMed;
(d) A. E. Frissen, A. T. M. Marcelis and H. C. Van Der Plas, Tetrahedron, 1989, 45, 803–812 CrossRef CAS;
(e) R. Willand-Charnley, B. W. Puffer and P. H. Dussault, J. Am. Chem. Soc., 2014, 136, 5821–5823 CrossRef CAS PubMed.
- For examples of bioactive dihydrobenzofurans, see:
(a) R. J. Nevagi, S. N. Dighe and S. N. Dighe, Eur. J. Med. Chem., 2015, 97, 561–581 CrossRef CAS PubMed;
(b) D. P. Zlotos, R. Jockers, E. Cecon, S. Rivara and P. A. Witt-Enderby, J. Med. Chem., 2014, 57, 3161–3185 CrossRef CAS PubMed;
(c) Z. Chen, M. Pitchakuntla and Y. Jia, Nat. Prod. Rep., 2019, 36, 666–690 RSC.
- The corresponding benzofurans of products in Scheme 4 are expensive (>$500/gram) or not commercially available according to a search of the online chemical catalog, https://www.emolecules.com, accessed May 17, 2022.
|
This journal is © The Royal Society of Chemistry 2022 |
Click here to see how this site uses Cookies. View our privacy policy here.